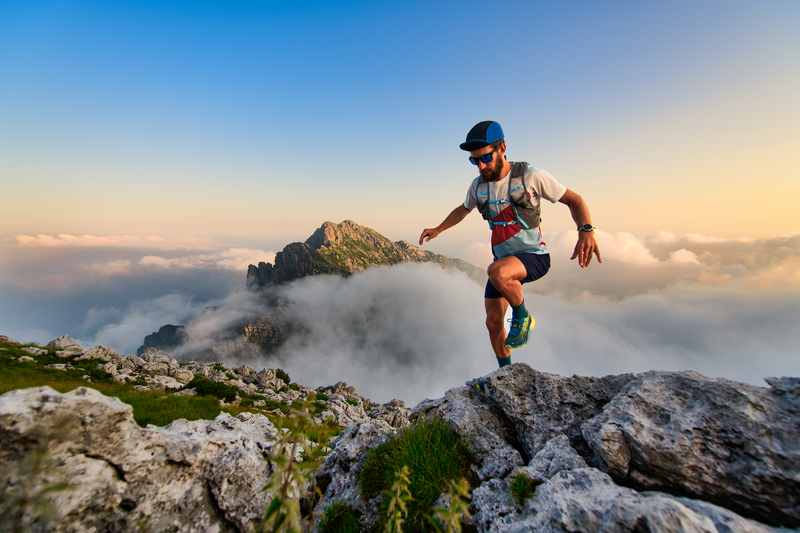
95% of researchers rate our articles as excellent or good
Learn more about the work of our research integrity team to safeguard the quality of each article we publish.
Find out more
EDITORIAL article
Front. Astron. Space Sci. , 10 November 2021
Sec. Space Physics
Volume 8 - 2021 | https://doi.org/10.3389/fspas.2021.801868
This article is part of the Research Topic Advances in Space Plasma Turbulence: Theory and Observations View all 7 articles
Editorial on the Research Topic
Advances in Space Plasma Turbulence: Theory and Observations
The Solar System is permeated by a continuous flow of a supersonic highly ionized gas (plasma) that gives rise to the heliosphere. This extends up to about 150 AU from the Sun, eventually interacting with the plasma forming the local interstellar medium (Burlaga et al., 2008; Burlaga et al., 2020). Since the beginning of the satellite era, it has been observed that the interplanetary plasma is in a turbulent state (Coleman and Paul, 1968) dominated by nonlinear interactions that induce a cross-scale transfer of energy, the so-called turbulent cascade, over a broad range of scales. These interactions have been reproduced in numerical simulations both at macroscopic scales, where the plasma can be treated as a magnetofluid and the dynamic is governed by the magnetohydrodynamic (MHD) equations (Biskamp, 2003), and at smaller scales, where particles become demagnetized and kinetic process are dominant. Since the matter in the Universe is predominantly in the plasma state, the opportunity to analyze in-situ the heliospheric plasma is of great interest for the study of physical processes in distant objects, such as supernova remnants blast waves, the hot gas within cluster of galaxies and the interstellar medium. It is widely accepted that the magnetic turbulent cascade in space plasmas (i.e., in the solar wind, in the plasma close to the Earth, and in the planetary magnetospheres) spontaneously generates coherent structures, also called magnetic discontinuities, over a broad range of scales where magnetic energy tends to clusterize, giving rise to magnetic intermittency (Marsch and Tu, 1997; Sorriso-Valvo et al., 1999; Perri et al., 2012; Perrone et al., 2020). In the last years, several methodologies have been developed for detecting such structures (Tsurutani and Smith, 1979; Greco et al., 2008; Li, 2008; Greco et al., 2018). Coherent structures can also be observed in numerical simulations and are characterized by the enhancement of the work done by the fields on the particles, measured via J·E (where J is the current density vector and E is the electric field). This quantity represents the magnetic energy dissipation within the structure (Wan et al., 2015). Distortions of the particle distribution function are also observed at kinetic level (Valentini et al., 2016). Therefore, coherent structures represent locations where dissipation and acceleration processes are likely to occur. For example, at ion and electron scales a fraction of magnetic discontinuities are expected to reconnect and convert magnetic energy into plasma heating and particle acceleration. On the other hand, in the kinetic range of turbulence the interaction between charged particles and plasma wave modes contribute to the energy transfer, and eventually to the energy dissipation (Leamon et al., 2000; Gary and Smith, 2009; Schekochihin et al., 2009; Sorriso-Valvo et al., 2019). Recent high-resolution spacecraft observations provided new insights in cross-scale connection of intermittent discontinuities, going from proton down to electron scales (Burch et al., 2016; Yordanova et al., 2016; Vörös et al., 2017). The investigation of kinetic turbulence in the near-Earth environment is today boosted by the Magnetospheric Multiscale (MMS) mission, which has permitted high resolution measurements of electric, magnetic fields, ion and electron velocity distribution functions (VDFs). Additionally, the recent launch of Parker Solar Probe and Solar Orbiter are advancing our understanding of solar wind turbulence both at large, intermediate, and small scales in a region of the interplanetary space close to the source of solar wind plasma.
This Research topic in Frontiers in Astronomy and Space Sciences gathers research papers on pioneering observations, theories, and numerical models that describe physical processes related to the space plasma turbulence cascade and heating, to the magnetic structure formation, and addressing new perspectives in such a research field.
Solar wind structures that are not affected by the radial evolution of the plasma turbulence from the solar corona to 1 AU are analyzed in the review paper by Borovsky. An comprehensive overview of large scale and inertial range structures is reported. These include corotating interaction region stream interfaces, periodic density structures, anisotropic magnetic structures, non-evolving Alfvénic structures, ion composition boundaries with associated current sheets, electron-strahl-intensity boundaries and associated current sheets. According to the author, observations suggest that they are not destroyed by the magnetic turbulence evolution in the inner heliosphere. Such results call for a more in depth investigation on the nature of fluctuations in the solar wind and their role in the survival of structures in the plasma. Implications for future solar-wind measurements are also discussed.
The origin of solar-wind magnetic fluctuations as a result of an active turbulent cascade has been investigated by Borovsky through a statistical study of the orientation of strong current sheets (CSs). CSs have been found to be mostly perpendicular to the Parker-spiral direction (Borovsky, 2008). Thus, if the CSs are generated by the turbulent cascade, the passage of a shock wave would destroy and reconstruct them isotropically. In this paper the author analyzed 109 interplanetary shock crossings to check whether compression isotropizes the direction of the CSs or not. Indeed, the compression occurring at the shock crossing should reduce the angular change. The change in the normal orientation between successive CSs is computed. The statistical analysis performed upstream and downstream of the shocks for strong CS structures shows that the angular change maintains the same distribution both closer to the shock front or further out downstream. Therefore, compressions do not isotropize the CS direction, suggesting a possible solar origin of such structures.
Another investigation on the characteristics of magnetic field fluctuations is performed in the paper by Carbone et al. They introduce a Brownian–like framework to describe the high–frequency dynamics of turbulent magnetic fluctuations. In the presented model, the complex nonlinear plasma dynamics is described as a stochastic process via a random forcing term, whereas the dissipative collisionless processes are represented by a linear damping term. This approach is able to describe the main properties of the magnetic energy spectra observed at high frequency in the solar wind.
A fundamental plasma process that leads to energy dissipation in a collisionless turbulent plasma is presented in Califano et al. They show results from a thorough numerical study of electron-only reconnection, a mechanism contributing to plasma energization that has been recently observed by the MMS spacecraft (Phan et al., 2018). They simulate freely decaying, 2D-3V turbulence by solving the hybrid-Vlasov–Maxwell equations, coupled to a generalized Ohm’s law that decouples the magnetic field from the ion dynamics at ion scales, while allowing complete unfreezing of the magnetic flux with respect to the electron fluid motion at electron scales. By comparing the statistical and geometrical properties of current structures generated in the two simulations (a snapshot of which is shown in Figure 1), the authors demonstrate that electron-only reconnection is only observed when the energy is injected at small scales, while ion and electron reconnection is present for the standard simulation. They conclude that some small-scale mechanism, as for example micro-instabilities or shocks, need to be present in order to generate electron-only reconnection in a turbulent environment.
FIGURE 1. A snapshot of the hybrid-Vlasov–Maxwell numerical simulation showing shaded iso-contours of the out-of-plane electron flow. Adapted from Califano et al., this volume.
The interrelation between turbulence and magnetic reconnection is further explored in Zharkova and Xia, although from a different perspective. These authors use particle-in-cell numerical simulations with a strong guide field to describe the generation of turbulence by particle beams accelerated during reconnecting current sheets. The proposed mechanism produces ion-scale and electron-scale turbulence at some distance from the reconnection site. The authors describe the statistical properties of the turbulent fluctuations so generated, and discuss the possible scenarios for their role in the small-scale energy cascade process.
Finally, Réville et al. have adopted a shell turbulence model to investigate the origin of the first ionization potential (FIP) effect. The FIP effect refers to the enrichment of elements that have low FIP with respect to photospheric abundances. It is believed to be indicative of chromospheric conditions and one of the current theories is that the FIP-bias has its origin in the turbulence and resulting ponderomotive force acting at the top of the chromosphere. The different amount of FIP effect observed in fast and slow wind has been considered an indication for a different origin of slow and fast wind. However, turbulence is known to contribute to solar wind acceleration, bearing the question of whether FIP fractionation occurs in open field regions as well. To address this problem Réville et al. perform a parameter study by combining global MHD simulations with a nonlinear shell model to simulate incompressible turbulence, and compare the effect of the turbulence properties on the FIP fractionation in both open field lines and coronal loops. This work shows that turbulence can explain the observed FIP-bias, depending on the injection scale of the turbulence, and that the FIP effect is observed not only in loops, but also in open field regions.
SP wrote the first draft of the Editorial. SP, LS-V, AT, and PH wrote parts of the Editorial. All authors contributed to manuscript revision, read, and approved the submitted version.
The authors declare that the research was conducted in the absence of any commercial or financial relationships that could be construed as a potential conflict of interest.
All claims expressed in this article are solely those of the authors and do not necessarily represent those of their affiliated organizations, or those of the publisher, the editors, and the reviewers. Any product that may be evaluated in this article or claim that may be made by its manufacturer is not guaranteed or endorsed by the publisher.
Borovsky, J. E. (2008). Flux Tube Texture of the Solar Wind: Strands of the Magnetic Carpet at 1 AU? J. Geophys. Res. (Space Physics). 113, A08110. doi:10.1029/2007ja012684
Burch, J. L., Torbert, R. B., Phan, T. D., Chen, L. J., Moore, T. E., Ergun, R. E., et al. (2016). Electron-Scale Measurements of Magnetic Reconnection in Space. Science. 352, aaf2939. doi:10.1126/science.aaf2939
Burlaga, L. F., Ness, N. F., Acuña, M. H., Lepping, R. P., Connerney, J. E. P., and Richardson, J. D. (2008). Magnetic Fields at the Solar Wind Termination Shock. Nature. 454, 75–77. doi:10.1038/nature07029
Burlaga, L. F., Ness, N. F., Berdichevsky, D. B., Jian, L. K., Park, J., and Szabo, A. (2020). Voyager 1 and 2 Observations of a Change in the Nature of Magnetic Fluctuations in the VLISM With Increasing Distance From the Heliopause. Astron. J. 160, 40. doi:10.3847/1538-3881/ab94a7
Coleman, P. J. J., and Paul, J. (1968). Turbulence, Viscosity, and Dissipation in the Solar-Wind Plasma. Astrophys. J. 153, 371. doi:10.1086/149674
Gary, S. P., and Smith, C. W. (2009). Short-Wavelength Turbulence in the Solar Wind: Linear Theory of Whistler and Kinetic Alfvén Fluctuations. J. Geophys. Res. 114, a–n. doi:10.1029/2009JA014525
Greco, A., Chuychai, P., Matthaeus, W. H., Servidio, S., and Dmitruk, P. (2008). Intermittent MHD Structures and Classical Discontinuities. Geophys. Res. Lett. 35, L19111. doi:10.1029/2008GL035454
Greco, A., Matthaeus, W. H., Perri, S., Osman, K. T., Servidio, S., Wan, M., et al. (2018). Partial Variance of Increments Method in Solar Wind Observations and Plasma Simulations. Space Sci. Rev. 214, 1. doi:10.1007/s11214-017-0435-8
Leamon, R. J., Matthaeus, W. H., Smith, C. W., Zank, G. P., Mullan, D. J., and Oughton, S. (2000). MHD‐Driven Kinetic Dissipation in the Solar Wind and Corona. Astrophys. J. 537, 1054–1062. doi:10.1086/309059
Li, G. (2008). Identifying Current-Sheet-Like Structures in the Solar Wind. Astrophys. J. 672, L65–L68. doi:10.1086/525847
Marsch, E., and Tu, C.-Y. (1997). Intermittency, Non-Gaussian Statistics and Fractal Scaling of MHD Fluctuations in the Solar Wind. Nonlin. Process. Geophys. 4, 101–124. doi:10.5194/npg-4-101-1997
Perri, S., Goldstein, M. L., Dorelli, J. C., and Sahraoui, F. (2012). Detection of Small-Scale Structures in the Dissipation Regime of Solar-Wind Turbulence. Phys. Rev. Lett. 109, 191101. doi:10.1103/PhysRevLett.109.191101
Perrone, D., Bruno, R., D’Amicis, R., Telloni, D., Marco, R. D., Stangalini, M., et al. (2020). Coherent Events at Ion Scales in the Inner Heliosphere: Parker Solar Probe Observations During the First Encounter. Astrophys. J. 905, 142. doi:10.3847/1538-4357/abc480
Phan, T. D., Eastwood, J. P., Shay, M. A., Drake, J. F., Sonnerup, B. U. Ö., Fujimoto, M., et al. (2018). Electron Magnetic Reconnection Without Ion Coupling in Earth's Turbulent Magnetosheath. Nature. 557, 202–206. doi:10.1038/s41586-018-0091-5
Schekochihin, A. A., Cowley, S. C., Dorland, W., Hammett, G. W., Howes, G. G., Quataert, E., et al. (2009). Astrophysical Gyrokinetics: Kinetic and Fluid Turbulent Cascades in Magnetized Weakly Collisional Plasmas. Astrophys. J. Suppl. Ser. 182, 310–377. doi:10.1088/0067-0049/182/1/310
Sorriso-Valvo, L., Catapano, F., Retinò, A., Le Contel, O., Perrone, D., Roberts, O. W., et al. (2019). Turbulence-Driven Ion Beams in the Magnetospheric Kelvin-Helmholtz Instability. Phys. Rev. Lett. 122, 035102. doi:10.1103/PhysRevLett.122.035102
Sorriso-Valvo, L., Carbone, V., Veltri, P., Consolini, G., and Bruno, R. (1999). Intermittency in the Solar Wind Turbulence through Probability Distribution Functions of Fluctuations. Geophys. Res. Lett. 26, 1801–1804. doi:10.1029/1999GL900270
Tsurutani, B. T., and Smith, E. J. (1979). Interplanetary Discontinuities: Temporal Variations and the Radial Gradient from 1 to 8.5 AU. J. Geophys. Res. 84, 2773–2787. doi:10.1029/JA084iA06p02773
Valentini, F., Perrone, D., Stabile, S., Pezzi, O., Servidio, S., Marco, R. D., et al. (2016). Differential Kinetic Dynamics and Heating of Ions in the Turbulent Solar Wind. New J. Phys. 18, 125001. doi:10.1088/1367-2630/18/12/125001
Vörös, Z., Yordanova, E., Varsani, A., Genestreti, K. J., Khotyaintsev, Y. V., Li, W., et al. (2017). MMS Observation of Magnetic Reconnection in the Turbulent Magnetosheath. J. Geophys. Res. Space Phys. 122, 11–467. doi:10.1002/2017JA024535
Wan, M., Matthaeus, W. H., Roytershteyn, V., Karimabadi, H., Parashar, T., Wu, P., et al. (2015). Intermittent Dissipation and Heating in 3D Kinetic Plasma Turbulence. Phys. Rev. Lett. 114, 175002. doi:10.1103/PhysRevLett.114.175002
Keywords: plasma turbulence, in situ observations, numerical simulations, magnetic reconnection, dissipation processes
Citation: Perri S, Sorriso-Valvo L, Tenerani A and Hellinger P (2021) Editorial: Advances in Space Plasma Turbulence: Theory and Observations. Front. Astron. Space Sci. 8:801868. doi: 10.3389/fspas.2021.801868
Received: 25 October 2021; Accepted: 26 October 2021;
Published: 10 November 2021.
Edited and reviewed by:
Rudolf von Steiger, University of Bern, SwitzerlandCopyright © 2021 Perri, Sorriso-Valvo, Tenerani and Hellinger. This is an open-access article distributed under the terms of the Creative Commons Attribution License (CC BY). The use, distribution or reproduction in other forums is permitted, provided the original author(s) and the copyright owner(s) are credited and that the original publication in this journal is cited, in accordance with accepted academic practice. No use, distribution or reproduction is permitted which does not comply with these terms.
*Correspondence: Silvia Perri , c2lsdmlhLnBlcnJpQHVuaWNhbC5pdA==
Disclaimer: All claims expressed in this article are solely those of the authors and do not necessarily represent those of their affiliated organizations, or those of the publisher, the editors and the reviewers. Any product that may be evaluated in this article or claim that may be made by its manufacturer is not guaranteed or endorsed by the publisher.
Research integrity at Frontiers
Learn more about the work of our research integrity team to safeguard the quality of each article we publish.