- Key Laboratory of Gene Engineering of the Ministry of Education, State Key Laboratory of Biocontrol, School of Life Sciences, Sun Yat-sen University, Guangzhou, China
Intrinsic circadian clocks generate circadian rhythms of physiology and behavior, which provide the capabilities to adapt to cycling environmental cues that result from the self-rotation of the Earth. Circadian misalignment leads to deleterious impacts on adaptation and health in different organisms. The environmental cues on the interplanetary journey to and on Mars dramatically differ from those on Earth. These differences impose numerous adaptive challenges, including challenges for humans’ circadian clock. Thus, adaptation of circadian rhythms to the Martian environment is a prerequisite for future landing and dwelling on Mars. Here, we review the progress of studies associated with the influence of the Martian environment on circadian rhythms and propose directions for further study and potential strategies to improve the adaptation of the circadian clock for future Mars missions.
Introduction
Human factors determine the success or failure of a space mission (Oluwafemi et al., 2021). Manned space exploration creates many physiological challenges for astronauts (Kandarpa et al., 2019; Garrett-Bakelman et al., 2019). Astronauts encounter a variety of environmental factors that are dramatically different from the Earth’s surface during the journey to and from and after dwelling on the Moon or Mars.
After humans landed on the Moon more than half a century ago, the next objective is to land on Mars. Mars is a red planet with a mean radius of 0.53 that of Earth and possesses the most similar environment to Earth among the solar planets. Mars is also the most likely planet to find extraterrestrial life and is the most habitable for humans (Levchenko et al., 2018). The average distance between Earth and Mars is 54.6 million kilometers, and a to and from journey between Earth and Mars would last more than 500 days (Tafforin, 2015). To date, the space agencies of the US and China have proposed plans for manned Mars exploration (Parihar et al., 2015; China Global Television Network, 2021). To live on Mars, artificial ecosystems must be established, and other organisms must be transported from Earth, including crops and animals, as food sources. For instance, in the Controlled Ecological Life Support System (CELSS) experiment, volunteers in a simulated cabin cultured crops such as zophobas, spirulina, maize, potato, sweet potato, other vegetables, and fruits (Tong, 2018). After landing on Mars, the survival and adaptation of these species will be critical issues.
Altered circadian rhythms in many other species have also been observed in space conditions (Mergenhagen and Mergenhagen, 1989; Hoban-Higgins et al., 2003; Sulzman et al., 1984; Wang et al., 2014; Guo et al., 2014). The circadian rhythms of Earthly organisms will be subject to change to a number of environmental cues on Mars and during the journey, including light, radiation, gravity, magnetic field, isolation, and confinement. As noted by Barger et al., “Clearly, without appropriate circadian rhythm and fatigue management countermeasures, maintaining daily life on the Mars day presents a significant challenge” (Barger et al., 2012).
Circadian Rhythms and Entrainment
Circadian rhythms of numerous physiological and behavioral variables, e.g., locomotor activity, growth, body temperature, photosynthesis, hormone secretion, and oxygen consumption, have been documented across kingdoms. Circadian rhythms are generated and controlled by endogenous circadian clocks. In higher animals, circadian clocks constitute pacemakers as central oscillators and peripheral oscillators (Bliss and Heppner, 1976). In humans, suprachiasmatic nuclei (SCN) are the pacemakers that orchestrate circadian rhythms of the remaining peripheral tissues (Welsh et al., 2010).
At the molecular level, eukaryotic circadian clock systems consist of positive and negative elements that form the transcription-translational feedback loop (TTFL). Basically, in TTFL the positive elements function as transcription factors (TFs) binding to the promoter regions of the negative elements to trigger the transcription of the latter; the transcribed and translated proteins act as negative elements to repress the capability of the positive elements as TFs (Bell-Pedersen et al., 2005; Zhang et al., 2011) (Figure 1). The circadian clock genes between animals, such as fruit flies and mammals, have certain homology. In contrast, there is no significant conservation between the clock counterparts in bacteria, fungi, plants, and animals (Figure 1). However, the regulatory mechanisms are highly conserved across kingdoms. In addition to TTFL, multiple regulatory layers are involved in the regulation of circadian clock gene expression and function, including at epigenetic, post-transcriptional and post-translational levels (Gallego and Virshup, 2007; Doherty and Kay, 2010; Hirano et al., 2016; Proietto et al., 2015; Hurley et al., 2016).
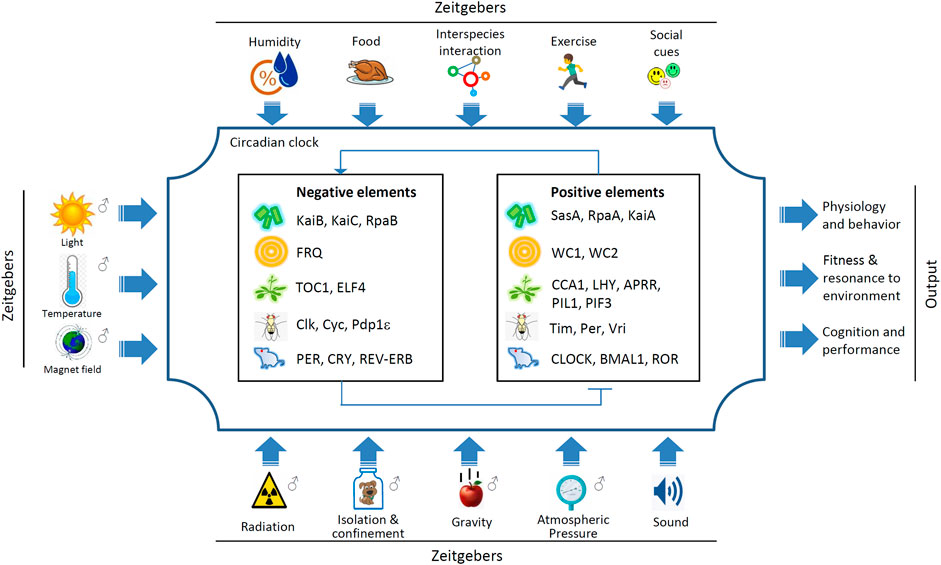
FIGURE 1. The molecular control of circadian clock systems. The negative and positive elements of circadian clock factors in representative models are depicted, and bacteria (S. elongatus), fungi (N. crassa), plants (A. thaliana), insects (D. melanogaster), and mammals (Mus musculus) are depicted (Bell-Pedersen et al., 2005; Doherty and Kay, 2010; Zhang et al., 2011). Environmental cues that dramatically differ from those on Earth are presented. Note that in this figure, cognition refers to animals only and performance refers to humans only. Isolation and confinement apply to animals and humans.
In prokaryotes, Cyanobacteria Synechococcus elongatus is one of the few species that have been found to possess an endogenous circadian clock. In S. elongatus, the core circadian clock protein KaiABC can dynamically interact and generate oscillations of phosphorylation changes in the KaiC protein. In addition, transcriptional regulation and KaiABC oscillation promote the circadian robustness of each other reciprocally under different growth conditions (Zwicker et al., 2010).
Circadian clocks endow the capabilities (also called fitness) to adapt to cycling environments. Consequently, circadian misalignment leads to comprehensive negative effects on physiology, psychology and behavior. Organisms show the highest adaptability to the environment when the periods of their endogenous circadian clocks are the closest to the environmental cycling periods (Ouyang et al., 1998; Woelfle et al., 2004; Johnson 2005; Sharma 2003). The free running period (FRP) of humans is approximately 24.9 h (Miles et al., 1977). Forced desynchronization under short light-dark (LD) cycles has also been used to measure the length of FRP, and the result is approximately 24.2 h (Czeisler et al., 1999; Duffy et al., 2011). However, short LD cycles may add superimposed impacts on the endogenous circadian system. In the entrainment range, a circadian rhythm can be aligned with the period of the cycling environmental cue. Environmental cues, including natural factors (e.g., light, temperature, gravity, magnetic field, sound, atmospheric pressure) and social factors (e.g., exercise, social interaction, interspecies interaction), are termed zeitgeber (German) or time givers (Figure 1) (Honma and Honma, 2001). The entrainment ranges differ for different organisms and different physiological or behavioral variables may exist in the same species (Guo et al., 2020).
Desynchronization of circadian rhythms in ambient environments with periods beyond the entrainment ranges causes decreased adaptation, competence, fitness, growth rate, fertility, and shortened lifespan (López-Otín and Kroemer, 2021; Allada and Bass, 2021; Sharma, 2003; Pittendrigh and Minis, 1972; Mormont et al., 2000). Circadian rhythm is a hallmark of human health (López-Otín and Kroemer, 2021), and circadian misalignment accounts for a variety of diseases, impaired mood (e.g., depression), decreased cognition and performance (Woller and Gonze, 2021; Ma et al., 2019; Guo et al., 2020; Bechtold et al., 2010; Mallis and DeRoshia, 2005). A circadian rhythm with less robust rhythmicity usually possesses a wider entrainment range. The locomotor rhythmicity of Clock mutant mice could be aligned with LD28 and LD32 compared to wild type, which only adapts to the LD24 regimen (Udo et al., 2004; Erzberger et al., 2013). However, a more robust circadian rhythm shows higher adaptability under LD24 (Solovev et al., 2019).
Different Environmental Cues on Mars and Their Effects on Circadian Rhythms
Light Conditions and Day Length
Light is the strongest zeitgeber to entrain circadian rhythms in most species. However, Mars and Earth have dramatically different light conditions, e.g., light intensity, light spectrum, and solar day-night cycling period (Mcphee and Charles, 2009). These factors make circadian adaptation more complex to achieve and results in negative effects on humans and other Earthly organisms to visit and live on Mars.
Light Intensity and Spectrum on Mars
Blue light at approximately 460 nm is the most effective at entraining circadian rhythms in many organisms, e.g., Cyanobacteria, Neurospora crassa, Arabidopsis thaliana, Drosophila melanogaster, and mammals, including humans. In addition, red light modulates cyanobacteria and the plant circadian clock (Cashmore et al., 1999; McClung, 2006; Linden 2002; Mullineaux, 2001). Regarding light intensity, only bright white light (over 2,500 lux) appears to be a sufficiently effective circadian zeitgeber in humans (Broadway et al., 1987). However, the sky radiant energy density on Mars is about 43% (1.5 in W m−2μm−1 sr−1–10.2 in W m−2μm−1 sr−1) of that on Earth, which is due to the distance from the sun, dust in the air, season, and weather (Moores et al., 2007; Whiteway et al., 2008; National Aeronautics and Space Administration (NASA), 2020). Although the intrinsic period of the human circadian clock is ∼25 h, under weak lighting conditions, the rhythms of the activity–rest cycle and melatonin could only be entrained to a period of 24 h but not 23.5 h or 24.6 h (Wright et al., 2001).
The light spectrum on Mars is also dramatically different. With an atmosphere rich in dust particles, blue light is preferentially absorbed, and as a result, the sky looks redder (chromaticity of x = 0.35, y = 0.34 standard deviations of σx = 0.01 and σy = 0.02) than that on Earth by δx = 0.04 and δy = 0.01 (Maki et al., 1999; Maki et al., 2021; Selsis et al., 2008), which is adverse for maintaining circadian rhythms in many Earth organisms. In addition to the circadian system, light parameters are crucial for many other physiological processes, and light intensity, light-dark ratio, and dark length are determinants for growth, development and reproduction (Saunders, 1982; Bennie et al., 2016; Irwin, 2018).
Martian Day Length
The solar day length of Mars is 24 h 39 min and 35 s (∼24.65 h), which is the closest to that of Earth among the solar planets or satellites. Repeated failure to enter this period will result in circadian desynchrony and survival for Earthly organisms and, consequently, disrupted sleep, impaired cognition and decision-making, and poor performance for animals and humans. During the Mars Pathfinder and Mars MER missions, ground mission control personnel were required to work on a Mars day schedule to interact with the spacecraft. They found it challenging to continuously stay on the 24.65 h period which led to increased fatigue, sleepiness, and irritability, and decreased concentration and energy. During the nearly 3-months Mars Pathfinder Mission, the Sojourner Rover supporting personnel abandoned the Mars day work schedule 1 month after the initiation (Barger et al., 2012). These data suggest that the Martian daily cycling period is outside the entrainment range of the human circadian system and that the circadian rhythms and performance of the personnel living on the Martian schedule will be impaired.
The 180-days CELSS experiment commenced in June 2016 to explore physiological, psychological, and behavioral changes during long-term isolation and exposure to Mars solar days (Shi et al., 2018; Yang et al., 2021). In the four volunteers of this experiment, the levels of total protein and globulin decreased and the bilirubin level increased under the isolation environment; the levels of total protein and globulin increased on Day 75 in the Mars solar day period (Chen et al., 2020). CELSS lasted for 180 days during which the volunteers lived under a Mars day (24.65 h) during the period from 71 to 107 days. One of the four volunteers wrote a diary every day and had it published (Tong, 2018). Yang et al. (2021) analyzed the published diary using a natural language processing tool and found a decrease in positive emotion during the Mars day period and an increased ratio of diaries with lower positive scores than average, although these changes were not significant. This single case report suggests that psychological changes may occur due to misalignment with the Mars day schedule. Comprehensive evaluation of the changes in physiological and behavioral rhythms under a simulated Martian daily period is necessary, as desynchronization between different rhythms may occur under a non-24 h environmental period.
Microgravity and Low Gravity
Gravity is almost zero during orbital flight or on the journey to and from Mars. The gravity on Mars is 3.711 m/s2, which is approximately 38% of the gravity on Earth (9.807 m/s2) (Heer et al., 2000). Microgravity has been shown to induce changes in circadian rhythms in many tested organisms (Sulzman et al., 1984; Hoban-Higgins et al., 2003; Wang et al., 2014; Guo et al., 2014; Holley et al., 2003). Chlamydomonas reinhardii displayed an increased amplitude of photoaccumulation rhythm under microgravity (Mergenhagen and Mergenhagen, 1989). Desert beetles showed differential locomotor periods and activity patterns in constant light and constant dark under microgravity or hypergravity (Hoban-Higgins et al., 2003).
For humans and animals, exposure to low gravity causes shifts in body fluids, space motion sickness, atrophy, bone demineralization and mass loss, immune system deregulation and disruption of senses (Bettiol et al., 2018). Circadian rhythms, including body temperature, sleep-wake cycle, cardiovascular variables, locomotor activity, affected cognition and performance of astronauts, are prone to change under microgravity (Pavy Le-Traon and Roussel, 1993; Flynn-Evans et al., 2016; Mallis and DeRoshia, 2005; Guo et al., 2014; Ma et al., 2015; Garrett-Bakelman et al., 2019; Zhang et al., 2021). RNA-sequencing data from the NASA GeneLab space mission revealed that clock gene expression in spaceflight mice revealed an expression pattern different from the ground control, and spaceflight promoted asynchrony of gene expression of circadian clock genes between peripheral tissues. Genes showing altered circadian expression are associated with jet lag, sleep disorder, cancer, lifestyle-related diseases, and mental disorders (Fujita et al., 2020).
Microorganisms in space are prone to changes in their metabolism, antibiotic efficacy, pathogenicity and virulence and exhibit increased virulence, enhanced formation of biofilms, or development of resistance to specific antibiotics (Green et al., 2021; Gilbert et al., 2020; Huang et al., 2018; Klaus and Howard et al., 2006; Bijlani et al., 2021). Immune cell stimulation, Toll-like receptors and pathogen-associated molecular patterns can be altered in microgravity, which affects immunological crosstalk and response. As a consequence, immune dysfunction caused by exposure to microgravity may increase the chance of bacterial infection (Krieger et al., 2021; Gertz et al., 2020; ElGindi et al., 2021; Wilson et al., 2007). As the circadian clock extensively regulates immunity, it is likely to be implicated or relayed in the alteration of immunity in space (Scheiermann et al., 2013).
Low Magnetic Field
Organisms living on Earth are under the influence of Earth’s magnetic field, which affects development, metabolism, and information processing in their daily lives (Lindauer and Martin, 1985). The magnetic field has been linked to the circadian system, although the effects and mechanisms remain largely vague (Lewczuk et al., 2014). Small changes in Earth’s magnetic field result in modified circadian rhythms in fiddler crabs and other organisms (Brown et al., 1964; Bliss and Heppner, 1976). The orientation and navigation of many organisms is closely associated with the circadian clock, and the orientation may differentially rely on celestial bodies, meteorological variables, geomagnetism and intrinsic factors (Borgioli et al., 1999). Circadian clock-dependent orientation was altered in sparrows (Passer domesticus) under a zero magnetic field (Bliss and Heppner, 1976). The orientation of Dugesia and Nassarius showed circalunar rhythmicity, which could be modified by changing the direction or strength of the magnetic field (Brown et al., 1970; Bitz and Sargent, 1974). The magnetoreceptor is located in the abdomen of honey bees. Inconsistent results were reported regarding the effects of a magnetic field on the circadian rhythm of honey bees; one found no influence, and the other found that a strong magnetic field led to changes in the circadian rhythms (Gould et al., 1978; Neumann, 1988). The effects of magnetic fields on circadian rhythms are not aligned for all organisms; for instance, no significant effects were found in the filamentous fungus Neurospora (Bitz and Sargent, 1974).
Melatonin is a hormone synthesized in a circadian fashion in the pineal glands of vertebrates, including humans, that regulates sleep, circadian rhythm, and antioxidation (Turek and Gillette, 2004). In the pied flycatcher, melatonin synthesis was significantly repressed in a changed magnetic field (Schneider et al., 1994). The spiking of guinea pig pineal cells was changed by altering the magnetic field at the Earth-strength level (Semm et al., 1980), suggesting that the magnetic field affects circadian rhythms at physiological and tissue levels. The melatonin of a migratory bird, the pied flycatcher, exhibits a circadian rhythm that can be modified by an artificial magnetic field (Schneider et al., 1994).
At the molecular level, CRY1 determines magnetosensitivity, which is dependent on light but independent of the circadian clock (Gegear et al., 2008). A near null magnetic field (NNMF) caused changes in circadian clock genes (LHY, PRR7, and GI) in Arabidopsis (Agliassa and Maffei, 2019). CRY1 shows promoted phosphorylation, which may be associated with Arabidopsis seedling growth in a 500 μT magnetic field under pulsed light (Hammad et al., 2020). In Drosophila, putative magnetoreceptor (MagR)/CG8198, which is a homolog of the bacterial iron-sulfur cluster assembly IscA1, binds with the CRY1 protein, a highly conserved blue light-absorbing flavoprotein involved in the positive elements of circadian circuits. This Cry/MagR complex displays an intrinsic magnetic moment, suggesting that it is a Drosophila putative magnetosensor (Qin et al., 2016; Wan et al., 2021). On the other hand, an altered magnetic field elicits changed expression of circadian clock genes. Mouse SCN slices expressing PERIOD2:LUCIFERASE were exposed to MS consisting of a 50-mT field, and the amplitude and period of the luciferase rhythms were differentially affected at different circadian time points (Kassahun et al., 2020). These data demonstrate that magnetic fields exert influences on circadian rhythms.
Mars has a crustal magnetic field magnetized by an ancient global dynamo field ∼4 Ga ago; however, it does not have a global dipole magnetic field like Earth. The dipole core field at the surface is weaker than the present core field at the surface of the Earth (Mittelholz et al., 2018; Stevenson, 2001). These differences in the Mars magnetic field will exert influences on the circadian rhythms of Earth emigrants.
Exposure to Exceptional Radiation
Regarding the surface radiation of Earth, species are primarily exposed to low linear energy transfer (LET) photon radiation (i.e., X-rays and γ-rays with LET values of <10 keV/μm). In contrast, space radiation comprises electrons, protons (hydrogen nuclei), neutrons, alpha particles (helium nuclei) and heavy nuclei with very high energies (high charge and energy particles) and LET values of >10 keV/μm (Mishra and Luderer, 2019). In interplanetary space, the primary components of the radiation field comprise galactic cosmic rays (GCRs) and solar cosmic radiation. The journey to Mars is beyond the protective magnetosphere of the Earth, and the long period increases the exposure of astronauts to the spectrum of galactic cosmic rays (GCRs), which are low fluence but highly energetic and fully ionized nuclei (Hellweg and Baumstark-Khan, 2007; Parihar et al., 2015). During interplanetary travel, astronauts will be exposed to radiation belts containing charged particles trapped by the planet magnetic field. The estimated cumulative dose of radiation from a 3-years-long Mars mission is 0.4 Gy (Cucinotta and Durante, 2006). Owing to the lack of protection by the magnetosphere and the loss of atmosphere, the radiation is much higher on the surface of Mars than on Earth, which imposes many hazards on human health. Radiation on Mars and on the journey has been considered the most critical issue for long-term space missions (Hellweg and Baumstark-Khan, 2007; McPhee and Charles, 2009).
One essential hypothesis of circadian adaptive advantage is “escape from light” (Ditty et al., 2009), suggesting that circadian rhythms are protective against radiation damage. Gamma radiation has been demonstrated to affect the circadian patterns of mouse activity and potato O2 consumption (Brown et al., 1970). In addition to dictating circadian rhythm, circadian clock genes play a role in regulating cell division and tumorigenesis. Multiple circadian components have been implicated in the defense against radiation hazards. PER2 regulates the expression of a series of genes associated with the cell cycle and tumor suppression. Fu et al. found that after gamma radiation, Per2 mutation resulted in a marked increase in tumor development and reduced apoptosis in thymocytes compared to control mice (Fu et al., 2002). PER1 modulates the expression of key cell cycle regulators that sensitize human cancer cells to DNA damage-induced apoptosis. PER1 interacts with the checkpoint proteins ATM and checkpoint kinase 2 (CHK2). Abnormal expression of Per1 resulted in disturbed apoptosis induced by DNA damage or radiation (Gery et al., 2006; Zhu et al., 2019). CHK2 is a key component of the DNA damage response that links DNA damage–activated kinases with checkpoint effectors in the cell cycle and DNA repair machinery, and its expression is under circadian control. Neurospora PRD4, the homolog of CHK2, consistently interacts with FRQ, the counterpart of the negative element circadian clock in Neurospora. DNA damage induced by radiation resets the circadian clock in a CHK2-dependent fashion (Pregueiro et al., 2006). Based on these findings, maintenance of circadian rhythms may help alleviate radiation hazards.
Isolation and Confinement
During the long-distance interplanetary journey between Mars and Earth, astronauts will encounter long-term isolation and confinement (Oluwafemi et al., 2021). After the astronauts reach Mars and live there, they will spend most of their time in a closed ecological facility. The negative consequences of isolation comprise decrements in mood, sleep deprivation, problems in concentration, and interpersonal tensions (Tafforin, 2015).
Isolation and confinement impose effects on circadian rhythms and sleep. Wrist actigraph records indicated that weightlessness simulated by head-down bed rest led to decreased activity, which was immediately recovered after bed rest (Liang et al., 2014). During the Mars500 project, which is a simulation of manned flight to Mars, the volunteers displayed increased sleep and rest times across the mission until the last 2 months (Basner et al., 2013), reflecting the effects of simulated isolation on sleep-wake dynamics.
Similar experiments or trainings include the Isolation Study for European Manned Space Infrastructure (ISEMSI), Experimental campaign for European Manned Space Infrastructure (EXEMSI), Human Behavior in Extended Space flight (HUBES), Simulation of Flight International Crew on Space Station (SFINCSS) and programs conducted at the Flashline Mars Arctic Research Station (Tafforin 2015).
Social and Interspecies Interaction
Psychological challenges, including crew interaction, must also be considered (Oluwafemi et al., 2021; Dietrich 2004; Kanas et al., 2000). Social interaction has been shown to affect circadian rhythms in a variety of species, including rodents, roosters, Drosophila, and cave glowworms (Levine et al., 2002; Shimmura et al., 2015; Maynard and Merritt 2013; Oluwafemi et al., 2021). Interspecies interactions also contribute to circadian modification. Reciprocal influences exist extensively between some symbiotic species, and the circadian rhythms of the hosts are modulated by symbionts, such as the bobtail squid Euprymna scolopes and its luminous symbiont Vibrio fischeri (Heath-Heckman et al., 2013) and Convoluta roscoffensis and its symbiont green alga living inside the worm (Palmer, 2002). Here, we refer to social interaction as not only the interaction between human individuals but also the interaction between humans and other species.
Abundant microorganisms colonize the human body, including mucosal and skin environments, which is closely associated with human physiology and health. A prevalent proportion of the human microbiota displays a diurnal oscillatory pattern in its abundance (Ma et al., 2019; Collado et al., 2018; Thaiss et al., 2014). Furthermore, the symbiosis between the host and gut microbiota plays a critical role in maintaining the homeostasis of the host and dysbiosis of microbiota, which accounts for immune dysregulation and an impaired digestive system and metabolism (Knight and Girling, 2003; Thaiss et al., 2014). Symbiotic microbiota functions to bridge circadian rhythms with diverse effects, including development immunity and metabolism (Brooks et al., 2021; Kuang et al., 2019; Thaiss et al., 2014; Ma et al., 2019; Collado et al., 2018). Much evidence has revealed that space environment factors or analog conditions (e.g., microgravity, radiation, low fluid shear, and diet) cause altered composition of gut microbiota (Siddiqui et al., 2021; Acres et al., 2021; Cervantes and Hong, 2016; Saei and Barzegari et al., 2012; Li et al., 2015; Jiang et al., 2019). Improving the balance of symbiotic microbiota and the association between the circadian clock and microbiota in space remain areas to be further investigated.
Temperature is also a critical circadian zeitgeber as periodic temperature change can reset the phase and amplitude of circadian rhythm in many species (Rensing and Ruoff, 2002). However, to survive on Mars we have to maintain a similar ambient temperature region in the habitats to that on Earth. Atmospheric pressure, has also been demonstrated to affect circadian rhythms, for instance, the phosphorylation rhythms of KaiC protein in Cyanobateria (Kitahara et al., 2019), however, the study on this issue is very limited. Therefore, the potential effects of these two factors are not discussed in this review.
Countermeasures to Improve Circadian Rhythms Under Martian Conditions
Regardless of whether we are still living on Earth, some pilot studies have been carried out to assess potential countermeasures against circadian misalignment on Mars. To date, light is the only approach used as a countermeasure in almost all of the available reports. In the Phoenix Mars Lander (PML) mission, the personnel worked on a Mars day (24.65 h) for 78 days with additional manual illumination (0–4,000 lux) when at work as a countermeasure. However, the daily sleep durations were 5.98 ± 0.94 h for those who showed a synchronized sleep-wake cycle to the Mars day and 4.91 ± 1.22 h for those who failed to synchronize their sleep-wake cycles (Barger et al., 2012). As 7–8 h sleep is critical for sleep duration (Chen et al., 2020), this data suggests that all of the personnel may have insufficient sleep (Sgobba and Schlacht, 2018; Flynn-Evans et al., 2016). Certain human rhythms can be entrained to the Martian period; for instance, under moderately bright light (∼450 lux) or with bright light pulses, the sleep-wake cycles of the volunteers were synchronized with LD24.65 h (Scheer et al., 2007; Gronfier et al., 2007). However, assessment of the potential desynchronization between tissues and its physiological and behavioral consequences is critical since the adaptation of the sleep-wake cycle does not equal sychronization at other levels.
Candidate organisms to send to Mars could be screened. Nonhuman species (especially crops) with less robust rhythms and organisms with longer intrinsic periods might be more likely to adapt to the Martian environment (Figure 2). For instance, hydrodictyon shows high plasticity in its circadian period compared to other organisms (Bünning, 1964), suggesting that entrainment plasticity is adjustable. It is noteworthy to mention that despite the entrainment range of the locomotor rhythms, at the molecular and metabolic levels, they may have much narrower windows. Altered expression of circadian clock genes, such as Clock, also extends the entrainment range or accelerates adaptation to shifted environmental cues (Udo et al., 2004).
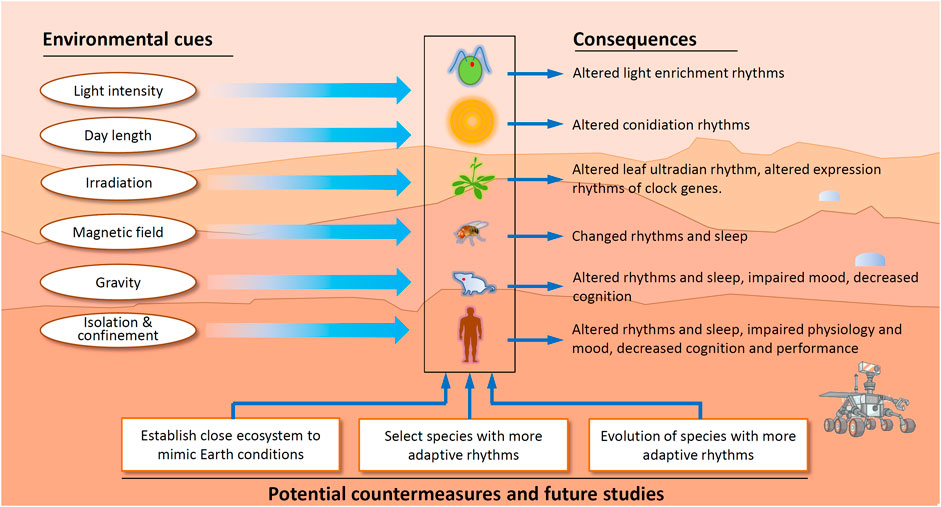
FIGURE 2. Martian factors with effects on the circadian clock and strategies for future investigation and improvement of circadian rhythms in representative species. C. rheinhardi, N. crassa, Arabidopsis, mouse and human are depicted in the central column (Sulzman et al., 1984; Mergenhagen and Mergenhagen, 1989; Mallis and DeRoshia, 2005; Guo et al., 2014; Ma et al., 2015; Agliassa and Maffei, 2019; Kandarpa et al., 2019; Zhang et al., 2021).
Food and exercise may also be used as countermeasures. Food is effective in entraining peripheral circadian clocks (Mendoza, 2007). Exercise also acts as a countermeasure to adjust circadian rhythm, but its effects are roughly opposite to those of melatonin according to the PRCs, which has been validated in a long-term space mission (Mendt et al., 2021). It will be interesting to assess whether atrophy and osteoporosis can be alleviated by exercise and whether timing has an influence on exercise effects, as the physiology and function of muscle and bone metabolism are under circadian control (Lefta et al., 2011; Zhang et al., 2020; Mayeuf-Louchart et al., 2015; Song et al., 2018; Swanson et al., 2018). Exercise affects circadian phase and amplitude with a roughly reversed PRC pattern to light entrainment (Lewis et al., 2018; Juhl et al., 2021; Moosavi et al., 2021; Refinetti 2016). However, the effects of these factors also require further assessment. In addition, other environmental factors may also affect circadian rhythms and sleep-wake cycles, for instance, the continuous and inevitable noise in the spacecraft during extended interplanetary travel. To this end, the reduction of noise through technology updates will be the solution.
Conclusion and Prospects
Astronauts and other Earth organisms will encounter many threats to their circadian rhythms during their travel to or from Mars and dwelling on Mars. Among the different cues from Earth, compared to light conditions, the conditions of low gravity, low magnetic field and stronger radiation are very challenging to change or overcome. These facts raise the following question: living on Mars with an Earth circadian clock or adapting the circadian clock to the Mars environment, which is more effective? This is not a black-or-white question; instead, it is a multidimensional question. For crops, plants, lower animals, and microorganisms, it is possible to screen those showing better adaptation to the Mars environment. Nevertheless, this does not apply to humans, at least at the beginning of Mars exploration, in light of ethics.
Establish an Enclosed Ecosystem to Mimic Earth Conditions
For humans, in the long future, the practicable strategy is to establish Earth-like closed ecosystems to shield hazardous cues and simulate Earth conditions, where most of the time living and working is spent (Bamsey et al., 2009). For crops, in the short-term future, people will cultivate crops in greenhouses in which the conditions are controlled to be similar to those on Earth. This process consumes a considerable amount of energy while artificial selection requires a large amount of time.
Select Emigrant Organisms With More Adaptive Rhythms
For organisms such as crops, farm animals or poultry, those with periods slightly longer than 24 h, adaptability to low light intensity and red light, a wide entrainment range or less robust rhythmicity might be considered prior candidates to transport to Mars. For the former, it might be easier for them to adapt to the Mars period; for the latter, arrhythmic organisms or organisms with less robust rhythms may be more competent than rhythmic organisms under a cycling environment with a period far beyond the entrainment range of rhythmic organisms (Ouyang et al., 1998; Erzberger et al., 2013).
Evolution of Circadian Clock on Mars
In an even longer-term future, the organisms on Mars may not always live in a closed ecosystem similar to Earth’s environment. More or less, fast or slow, circadian clocks and other aspects will start to evolve. We have limited knowledge about the evolutionary processes of the circadian clock, but further understanding of the circadian rhythms in caverns or other special environments may help. Moreover, we may impose artificial selection pressure to accelerate evolution in crops (Cavallari et al., 2011; Müller et al., 2016; Arnold et al., 2018; Lu et al., 2010).
In this article, there is little discussion on circalunar and circannual rhythms. Organisms on Earth are more or less modulated by the Moon (Raible et al., 2017). Mars has two satellites, Phobos and Deimos, suggesting a more complex influence. In addition, the Mars year is approximately 687 days, nearly double the year length of Earth, which also challenges Earthly organisms. As the regulation and underlying mechanisms of circalunar and circannual rhythms of Earth lives remain less investigated than circadian rhythms, there is even more research necessary on this topic in regards to live on Mars.
Author Contributions
RL, YH, and JG wrote the first draft of the manuscript and all authors contributed to manuscript revision, read, and approved the submitted version.
Funding
This work was supported by the Experiments for Space Exploration Program and the Qian Xuesen Laboratory, China Academy of Space Technology (TKTSPY-2020-04-21), the National Natural Science Foundation of China (Grants 31871188 and 3217090153), the Space Medical Experiment Program (HYZHXM03007) and the Open Fund of the National Key Laboratory of Human Factors Engineering in the Astronaut Center of China (SYFD062008K).
Conflict of Interest
The authors declare that the research was conducted in the absence of any commercial or financial relationships that could be construed as a potential conflict of interest.
Publisher’s Note
All claims expressed in this article are solely those of the authors and do not necessarily represent those of their affiliated organizations, or those of the publisher, the editors and the reviewers. Any product that may be evaluated in this article, or claim that may be made by its manufacturer, is not guaranteed or endorsed by the publisher.
Acknowledgments
We apologize for not citing all relevant important references due to the space limit.
References
Acres, J. M., Youngapelian, M. J., and Nadeau, J. (2021). The Influence of Spaceflight and Simulated Microgravity on Bacterial Motility and Chemotaxis. NPJ Microgravity 7 (1), 7. doi:10.1038/s41526-021-00135-x
Agliassa, C., and Maffei, M. E. (2019). Reduction of Geomagnetic Field (GMF) to Near Null Magnetic Field (NNMF) Affects Some Arabidopsis thaliana Clock Genes Amplitude in a Light Independent Manner. J. Plant Physiol. 232, 23–26. doi:10.1016/j.jplph.2018.11.008
Allada, R., and Bass, J. (2021). Circadian Mechanisms in Medicine. N. Engl. J. Med. 384 (6), 550–561. doi:10.1056/NEJMra1802337
Arnold, W., Ruf, T., Loe, L. E., Irvine, R. J., Ropstad, E., Veiberg, V., et al. (2018). Circadian Rhythmicity Persists through the Polar Night and Midnight Sun in Svalbard Reindeer. Sci. Rep. 8, 14466. doi:10.1038/s41598-018-32778-4
Bamsey, M., Berinstain, A., Graham, T., Neron, P., Giroux, R., Braham, S., et al. (2009). Developing Strategies for Automated Remote Plant Production Systems: Environmental Control and Monitoring of the Arthur Clarke Mars Greenhouse in the Canadian High Arctic. Adv. Space Res. 44, 1367–1381. doi:10.1016/j.asr.2009.08.012
Barger, L. K., Sullivan, J. P., Vincent, A. S., Fiedler, E. R., McKenna, L. M., Flynn-Evans, E. E., et al. (2012). Learning to Live on a Mars Day: Fatigue Countermeasures during the Phoenix Mars Lander mission. Sleep 35, 1423–1435. doi:10.5665/sleep.2128
Basner, M., Dinges, D. F., Mollicone, D., Ecker, A., Jones, C. W., Hyder, E. C., et al. (2013). Mars 520-d mission Simulation Reveals Protracted Crew Hypokinesis and Alterations of Sleep Duration and Timing. Proc. Natl. Acad. Sci. U S A. 110, 2635–2640. doi:10.1073/pnas.1212646110
Bechtold, D. A., Gibbs, J. E., and Loudon, A. S. (2010). Circadian Dysfunction in Disease. Trends Pharmacol. Sci. 31, 191–198. doi:10.1016/j.tips.2010.01.002
Bell-Pedersen, D., Cassone, V. M., Earnest, D. J., Golden, S. S., Hardin, P. E., Thomas, T. L., et al. (2005). Circadian Rhythms from Multiple Oscillators: Lessons from Diverse Organisms. Nat. Rev. Genet. 6, 544–556. doi:10.1038/nrg1633
Bennie, J., Davies, T. W., Cruse, D., and Gaston, K. J. (2016). Ecological Effects of Artificial Light at Night on Wild Plants. J. Ecol. 104, 611–620. doi:10.1111/1365-2745.12551
Bettiol, L., Kamaletdinova, G., Patel, D., Lakmal, Y., Oluwafemi, F., De La Torre, A., et al. (2018). “Manned Mars mission Risks Evaluation [Conference Presentation],” in IAC-18- B3.9.5. Proceedings of the 69th International Astronautical Congress, Bremen, Germany, October 1-5, 2018.
Bijlani, S., Stephens, E., Singh, N. K., Venkateswaran, K., and Wang, C. C. C. (2021). Advances in Space Microbiology. iScience 24, 102395. doi:10.1016/j.isci.2021.102395
Bitz, D. M., and Sargent, M. L. (1974). A Failure to Detect an Influence of Magnetic Fields on the Growth Rate and Circadian Rhythm of Neurospora Crassa. Plant Physiol. 53, 154–157. doi:10.1104/pp.53.2.154
Bliss, V. L., and Heppner, F. H. (1976). Circadian Activity Rhythm Influenced by Near Zero Magnetic Field. Nature 261, 411–412. doi:10.1038/261411a0
Borgioli, C., Martelli, L., Porri, F., D'Elia, A., Marchetti, G. M., and Scapini, F. (1999). Orientation in Talitrus Saltator (Montagu): Trends in Intrapopulation Variability Related to Environmental and Intrinsic Factors. J. Exp. Mar. Biol. Ecol. 238, 29–47. doi:10.1016/S0022-0981(99)00012-X
Broadway, J., Arendt, J., and Folkard, S. (1987). Bright Light Phase Shifts the Human Melatonin Rhythm during the Antarctic winter. Neurosci. Lett. 79, 185–189. doi:10.1016/0304-3940(87)90694-x
Brooks, J. F., Behrendt, C. L., Ruhn, K. A., Lee, S., Raj, P., Takahashi, J. S., et al. (2021). The Microbiota Coordinates Diurnal Rhythms in Innate Immunity with the Circadian Clock. Cell 184 (16), 4154–4167.e12. doi:10.1016/j.cell.2021.07.001
Brown, F. A., Barnwell, F. H., and Webb, H. M. (1964). Adaptation of the Magnetoreceptive Mechanism of Mud-Snails to Geomagnetic Strength. Biol. Bull. 127, 221–231. doi:10.2307/1539221
Brown, F. A., Hastings, J. W., and Palmer, J. D. (1970). The Biological Clock: Two Views. New York: Academic Press.
Cashmore, A. R., Jarillo, J. A., Wu, Y. J., and Liu, D. (1999). Cryptochromes: Blue Light Receptors for Plants and Animals. Science 284, 760–765. doi:10.1126/science.284.5415.760
Cavallari, N., Frigato, E., Vallone, D., Fröhlich, N., Lopez-Olmeda, J. F., Foà, A., et al. (2011). A Blind Circadian Clock in Cavefish Reveals that Opsins Mediate Peripheral Clock Photoreception. Plos Biol. 9, e1001142. doi:10.1371/journal.pbio.1001142
Cervantes, J. L., and Hong, B. Y. (2016). Dysbiosis and Immune Dysregulation in Outer Space. Int. Rev. Immunol. 35, 67–82. doi:10.3109/0883018510.3109/08830185.2015.1027821
Chen, H., Lv, K., Ji, G., Yuan, Y., Lu, L., Liang, F., et al. (2020). Physiological Acclimatization of the Liver to 180-Day Isolation and the Mars Solar Day. Biomed. Res. Int. 2020, 2796510. doi:10.1155/2020/2796510
China Global Television Network (2021). China Global Television Network. Available at: https://news.cgtn.com/news/2021-06-26/China-plans-to-launch-first-crewed-mission-to-Mars-in-2033-11oS5qxFp0k/index.html (Accessed June 26, 2021).
Collado, M. C., Engen, P. A., Bandín, C., Cabrera-Rubio, R., Voigt, R. M., Green, S. J., et al. (2018). Timing of Food Intake Impacts Daily Rhythms of Human Salivary Microbiota: a Randomized, Crossover Study. FASEB J. 32, 2060–2072. doi:10.1096/fj.201700697RR
Cucinotta, F. A., and Durante, M. (2006). Cancer Risk from Exposure to Galactic Cosmic Rays: Implications for Space Exploration by Human Beings. Lancet Oncol. 7, 431–435. doi:10.1016/S1470-2045(06)70695-7
Czeisler, C. A., Duffy, J. F., Shanahan, T. L., Brown, E. N., Mitchell, J. F., Rimmer, D. W., et al. (1999). Stability, Precision, and Near-24-Hour Period of the Human Circadian Pacemaker. Science 284, 2177–2181. doi:10.1126/science.284.5423.2177
Dietrich, M. (2004). Human Missions to Mars: New Psychological Challenges and Research Issues. Acta Astronautica 55, 781–790. doi:10.1016/j.actaastro.2004.05.013
Ditty, J. L., Mackey, S. R., and Johnson, C. H. (2009). Bacterial Circadian Programs. Berlin: Springer-Verlag Berlin Heidelberg, 212–219.
Doherty, C. J., and Kay, S. A. (2010). Circadian Control of Global Gene Expression Patterns. Annu. Rev. Genet. 44, 419–444. doi:10.1146/annurev-genet-102209-163432
Duffy, J. F., Cain, S. W., Chang, A. M., Phillips, A. J. K., Münch, M. Y., Gronfier, C., et al. (2011). Sex Difference in the Near-24-Hour Intrinsic Period of the Human Circadian Timing System. Proc. Nat. Acad. Sci. U S A. 108, 15602–15608. doi:10.1073/pnas.1010666108
ElGindi, M., Sapudom, J., Ibrahim, I. H., Al-Sayegh, M., Chen, W., Garcia-Sabaté, A., et al. (2021). May the Force Be with You (Or Not): The Immune System under Microgravity. Cells 10, 1941. doi:10.3390/cells10081941
Erzberger, A., Hampp, G., Granada, A. E., Albrecht, U., and Herzel, H. (2013). Genetic Redundancy Strengthens the Circadian Clock Leading to a Narrow Entrainment Range. J. R. Soc. Interf. 10, 20130221. doi:10.1098/rsif.2013.0221
Flynn-Evans, E. E., Barger, L. K., Kubey, A. A., Sullivan, J. P., and Czeisler, C. A. (2016). Circadian Misalignment Affects Sleep and Medication Use before and during Spaceflight. NPJ Microgravity 2, 15019. doi:10.1038/npjmgrav.2015.19
Fu, L., Pelicano, H., Liu, J., Huang, P., and Lee, C. (2002). The Circadian Gene Period2 Plays an Important Role in Tumor Suppression and DNA Damage Response In Vivo. Cell 111, 41–50. doi:10.1016/s0092-8674(02)00961-3
Fujita, S. I., Rutter, L., Ong, Q., and Muratani, M. (2020). Integrated RNA-Seq Analysis Indicates Asynchrony in Clock Genes between Tissues under Spaceflight. Life 10, 196. doi:10.3390/life10090196
Gallego, M., and Virshup, D. M. (2007). Post-translational Modifications Regulate the Ticking of the Circadian Clock. Nat. Rev. Mol. Cel. Biol. 8, 139–148. doi:10.1038/nrm2106
Garrett-Bakelman, F. E., Darshi, M., Green, S. J., Gur, R. C., Lin, L., Macias, B. R., et al. (2019). The NASA Twins Study: A Multidimensional Analysis of a Year-Long Human Spaceflight. Science 364, eaau8650. doi:10.1126/science.aau8650
Gegear, R. J., Casselman, A., Waddell, S., and Reppert, S. M. (2008). Cryptochrome Mediates Light-dependent Magnetosensitivity in Drosophila. Nature 454, 1014–1018. doi:10.1038/nature07183
Gertz, M. L., Chin, C. R., Tomoiaga, D., MacKay, M., Chang, C., Butler, D., et al. (2020). Multi-omic, Single-Cell, and Biochemical Profiles of Astronauts Guide Pharmacological Strategies for Returning to Gravity. Cell Rep 33, 108429. doi:10.1016/j.celrep.2020.108429
Gery, S., Komatsu, N., Baldjyan, L., Yu, A., Koo, D., and Koeffler, H. P. (2006). The Circadian Gene Per1 Plays an Important Role in Cell Growth and DNA Damage Control in Human Cancer Cells. Mol. Cel 22 (3), 375–382. doi:10.1016/j.molcel.2006.03.038
Gilbert, R., Torres, M., Clemens, R., Hateley, S., Hosamani, R., Wade, W., et al. (2020). Spaceflight and Simulated Microgravity Conditions Increase Virulence of Serratia marcescens in the Drosophila melanogaster Infection Model. NPJ Microgravity 6, 4. doi:10.1038/s41526-019-0091-2
Gould, J. L., Kirschvink, J. L., and Deffeyes, K. S. (1978). Bees Have Magnetic Remanence. Science 201, 1026–1028. doi:10.1126/science.201.4360.1026
Green, M. J., Aylott, J. W., Williams, P., Ghaemmaghami, A. M., and Williams, P. M. (2021). Immunity in Space: Prokaryote Adaptations and Immune Response in Microgravity. Life 11, 112. doi:10.3390/life11020112
Gronfier, C., Wright, K. P., Kronauer, R. E., and Czeisler, C. A. (2007). Entrainment of the Human Circadian Pacemaker to longer-Than-24-h Days. Proc. Natl. Acad. Sci. U S A. 104, 9081–9086. doi:10.1073/pnas.0702835104
Guo, J. H., Ma, X. H., Ma, H., Zhang, Y., Tian, Z. Q., Wang, X., et al. (2020). Circadian Misalignment in Submarine and Other Non-24 Hour Conditions – from Research to Application. Mil. Med. Res. 7, 39. doi:10.1186/s40779-020-00268-2
Guo, J. H., Qu, W. M., Chen, S. G., Chen, X. Y., Lv, K., Huang, Z. L., et al. (2014). Keeping the Right Time in Space: Importance of Circadian Clock and Sleep for Physiology and Performance of Astronauts. Mil. Med. Res. 1, 23. doi:10.1186/2054-9369-1-23
Hammad, M., Albaqami, M., Pooam, M., Kernevez, E., Witczak, J., Ritz, T., et al. (2020). Cryptochrome Mediated Magnetic Sensitivity in Arabidopsis Occurs Independently of Light-Induced Electron Transfer to the Flavin. Photochem. Photobiol. Sci. 19, 341–352. doi:10.1039/c9pp00469f
Heath-Heckman, E. A., Peyer, S. M., Whistler, C. A., Apicella, M. A., Goldman, W. E., and McFall-Ngai, M. J. (2013). Bacterial Bioluminescence Regulates Expression of a Host Cryptochrome Gene in the Squid-Vibrio Symbiosis. mBio 4, e00167–13. doi:10.1128/mBio.00167-13
Heer, M., Boerger, A., Kamps, N., Mika, C., Korr, C., and Drummer, C. (2000). Nutrient Supply during Recent European Missions. Pflugers Arch. 441 (2-3 Suppl. l), R8–R14. doi:10.1007/s004240000334
Hellweg, C. E., and Baumstark-Khan, C. (2007). Getting Ready for the Manned mission to Mars: the Astronauts' Risk from Space Radiation. Naturwissenschaften 94, 517–526. doi:10.1007/s00114-006-0204-0
Hirano, A., Fu, Y. H., and Ptáček, L. J. (2016). The Intricate Dance of post-translational Modifications in the Rhythm of Life. Nat. Struct. Mol. Biol. 23, 1053–1060. doi:10.1038/nsmb.3326
Hoban-Higgins, T. M., Alpatov, A. M., Wassmer, G. T., Rietveld, W. J., and Fuller, C. A. (2003). Gravity and Light Effects on the Circadian Clock of a Desert Beetle, Trigonoscelis Gigas. J. Insect Physiol. 49, 671–675. doi:10.1016/s0022-1910(03)00068-4
Holley, D. C., DeRoshia, C. W., Moran, M. M., and Wade, C. E. (2003). Chronic Centrifugation (Hypergravity) Disrupts the Circadian System of the Rat. J. Appl. Physiol. 95, 1266–1278. doi:10.1152/japplphysiol.00707.2002
Honma, K. I., and Honma, S. (2001). Zeitgebers, Entrainment and Masking of the Circadian System. Sapporo. Japan: Hokkaido University Press.
Huang, B., Li, D. G., Huang, Y., and Liu, C. T. (2018). Effects of Spaceflight and Simulated Microgravity on Microbial Growth and Secondary Metabolism. Mil. Med. Res. 5, 18. doi:10.1186/s40779-018-0162-9
Hurley, J. M., Loros, J. J., and Dunlap, J. C. (2016). Circadian Oscillators: Around the Transcription-Translation Feedback Loop and on to Output. Trends Biochem. Sci. 41, 834–846. doi:10.1016/j.tibs.2016.07.009
Irwin, A. (2018). The Dark Side of Light: How Artificial Lighting Is Harming the Natural World. Nature 553, 268–270. doi:10.1038/d41586-018-00665-7
Jiang, P., Green, S. J., Chlipala, G. E., Turek, F. W., and Vitaterna, M. H. (2019). Reproducible Changes in the Gut Microbiome Suggest a Shift in Microbial and Host Metabolism during Spaceflight. Microbiome 7, 113. doi:10.1186/s40168-019-0724-4
Johnson, C. H. (2005). Testing the Adaptive Value of Circadian Systems. Methods Enzymol. 393, 818–837. doi:10.1016/S0076-6879(05)93043-7
Juhl, O. J., Buettmann, E. G., Friedman, M. A., DeNapoli, R. C., Hoppock, G. A., and Donahue, H. J. (2021). Update on the Effects of Microgravity on the Musculoskeletal System. NPJ Microgravity 7, 28. doi:10.1038/s41526-021-00158-4
Kanas, N., Salnitskiy, V., Grund, E. M., Gushin, V., Weiss, D. S., Kozerenko, O., et al. (2000). Social and Cultural Issues during Shuttle/Mir Space Missions. Acta Astronaut 47, 647–655. doi:10.1016/s0094-5765(00)00102-8
Kandarpa, K., Schneider, V., and Ganapathy, K. (2019). Human Health during Space Travel: An Overview. Neurol. India 67 (Suppl.), S176–S181. doi:10.4103/0028-3886.259123
Kassahun, B. T., Bier, M., and Ding, J. (2020). Perturbing Circadian Oscillations in an In Vitro Suprachiasmatic Nucleus with Magnetic Stimulation. Bioelectromagnetics 41, 63–72. doi:10.1002/bem.22235
Kitahara, R., Oyama, K., Kawamura, T., Mitsuhashi, K., Kitazawa, S., Yasunaga, K., et al. (2019). Pressure Accelerates the Circadian Clock of Cyanobacteria. Sci. Rep. 9, 12395. doi:10.1038/s41598-019-48693-1
Klaus, D. M., and Howard, H. N. (2006). Antibiotic Efficacy and Microbial Virulence during Space Flight. Trends Biotechnol. 24, 131–136. doi:10.1016/j.tibtech.2006.01.008
Knight, D. J., and Girling, K. J. (2003). Gut flora in Health and Disease. Lancet 361, 1831. doi:10.1016/s0140-6736(03)13438-1
Krieger, S. S., Zwart, S. R., Mehta, S., Wu, H., Simpson, R. J., Smith, S. M., et al. (2021). Alterations in Saliva and Plasma Cytokine Concentrations during Long-Duration Spaceflight. Front. Immunol. 12, 725748. doi:10.3389/fimmu10.3389/fimmu.2021.725748
Kuang, Z., Wang, Y., Li, Y., Ye, C., Ruhn, K. A., Behrendt, C. L., et al. (2019). The Intestinal Microbiota Programs Diurnal Rhythms in Host Metabolism through Histone Deacetylase 3. Science 365, 1428–1434. doi:10.1126/science.aaw3134
Lefta, M., Wolff, G., and Esser, K. A. (2011). Circadian Rhythms, the Molecular Clock, and Skeletal Muscle. Curr. Top. Dev. Biol. 96, 231–271. doi:10.1016/B978-0-12-385940-2.00009-7
Levchenko, I., Xu, S., Mazouffre, S., Keidar, M., and Bazaka, K. (2018). Mars Colonization: Beyond Getting There. Glob. Chall. 3, 1800062. doi:10.1002/gch2.201800062
Levine, J. D., Funes, P., Dowse, H. B., and Hall, J. C. (2002). Resetting the Circadian Clock by Social Experience in Drosophila melanogaster. Science 298, 2010–2012. doi:10.1126/science.1076008
Lewczuk, B., Redlarski, G., Zak, A., Ziółkowska, N., Przybylska-Gornowicz, B., and Krawczuk, M. (2014). Influence of Electric, Magnetic, and Electromagnetic fields on the Circadian System: Current Stage of Knowledge. Biomed. Res. Int. 2014, 169459. doi:10.1155/2014/169459
Lewis, P., Korf, H. W., Kuffer, L., Groß, J. V., and Erren, T. C. (2018). Exercise Time Cues (Zeitgebers) for Human Circadian Systems Can foster Health and Improve Performance: a Systematic Review. BMJ Open Sport Exerc. Med. 4, e000443. doi:10.1136/bmjsem-2018-000443
Li, P., Shi, J., Zhang, P., Wang, K., Li, J., Liu, H., et al. (2015). Simulated Microgravity Disrupts Intestinal Homeostasis and Increases Colitis Susceptibility. FASEB J. 29, 3263–3273. doi:10.1096/fj.15-271700
Liang, X., Zhang, L., Shen, H., Chen, X., Wan, Y., Li, L., et al. (2014). Effects of a 45-day Head-Down Bed Rest on the Diurnal Rhythms of Activity, Sleep and Heart Rate. Biol. Rhythm Res. 45, 596–601. doi:10.1080/09291016.2014.882093
Lindauer, M., and Martin, H. (1985). “The Biological Significance of the Earth’s Magnetic Field,” in Progress in Sensory Physiology. Editors H. Autrum, D. Ottoson, E. R. Perl, R. F. Schmidt, H. Shimazu, and W. D. Willis (Berlin, Heidelberg: Springer), Vol. 5. doi:10.1007/978-3-642-70408-6_3
Linden, H. (2002). Circadian Rhythms. A white Collar Protein Senses Blue Light. Science 297, 777–778. doi:10.1126/science.1075485
López-Otín, C., and Kroemer, G. (2021). Hallmarks of Health. Cell 184, 33–63. doi:10.1016/j.cell.2020.11.034
Lu, W., Meng, Q. J., Tyler, N. J., Stokkan, K. A., and Loudon, A. S. (2010). A Circadian Clock Is Not Required in an Arctic Mammal. Curr. Biol. 20, 533–537. doi:10.1016/j.cub.2010.01.042
Ma, H., Li, Y., Liang, H., Chen, S., Pan, S., Chang, L., et al. (2019). Sleep Deprivation and a Non-24 H Working Schedule lead to Extensive Alterations in Physiology and Behavior. FASEB J. 33, 6969–6979. doi:10.1096/fj.201802727R
Ma, L., Ma, J., and Xu, K. (2015). Effect of Spaceflight on the Circadian Rhythm, Lifespan and Gene Expression of Drosophila melanogaster. PLoS One 10, e0121600. doi:10.1371/journal.pone.0121600
Maki, J. N., Golombek, M., Banerdt, W., Smrekar, S., Deen, R., Abarca, H., et al. (2021). Color Properties at the Mars InSight landing Site. Earth Space Sci. 8, e2020EA001336. doi:10.1029/2020EA001336
Maki, J. N., Lorre, J. J., Sith, P. H., Brandt, R. D., and Steinwand, D. J. (1999). The Color of Mars: Spectrophotometric Measurements at the Pathfinder landing Site. Geophys. Res. 104 (E4), 8781–8794. doi:10.1029/98JE01767
Mallis, M. M., and DeRoshia, C. W. (2005). Circadian Rhythms, Sleep, and Performance in Space. Aviat. Space Environ. Med. 76 (6 Suppl. l), B94–B107.
Mayeuf-Louchart, A., Staels, B., and Duez, H. (2015). Skeletal Muscle Functions Around the Clock. Diabetes Obes. Metab. 17 (Suppl. 1), 39–46. doi:10.1111/dom.12517
Maynard, A. J., and Merritt, D. J. (2013). Synchronization of Circadian Bioluminescence as a Group-Foraging Strategy in Cave Glowworms. Integr. Comp. Biol. 53, 154–164. doi:10.1093/icb/ict011
Mcphee, J. C., and Charles, J. B. (2009). Human Health and Performance Risks of Space Exploration Missions. Washington, D.C., USA: National Aeronautics and Space Administration. Lyndon B. Johnson Space Center. NASA SP-2009-3405, 106.
Mendoza, J. (2007). Circadian Clocks: Setting Time by Food. J. Neuroendocrinol. 19, 127–137. doi:10.1111/j.1365-2826.2006.01510.x
Mendt, S., Gunga, H. C., Felsenberg, D., Belavy, D. L., Steinach, M., and Stahn, A. C. (2021). Regular Exercise Counteracts Circadian Shifts in Core Body Temperature during Long-Duration Bed Rest. NPJ Microgravity 7, 1. doi:10.1038/s41526-020-00129-1
Mergenhagen, D., and Mergenhagen, E. (1989). The Expression of a Circadian Rhythm in Two Strains of Chlamydomonas Reinhardii in Space. Adv. Space Res. 9, 261–270. doi:10.1016/0273-1177(89)90082-3
Miles, L. E. M., Raynal, D. M., and Wilson, M. A. (1977). Blind Man Living in normal Society Has Circadian Rhythms of 24.9 Hours (Letter). Science 198, 421–423. doi:10.1126/science.910139
Mishra, B., and Luderer, U. (2019). Reproductive Hazards of Space Travel in Women and Men. Nat. Rev. Endocrinol. 15, 713–730. doi:10.1038/s41574-019-0267-6
Mittelholz, A., Morschhauser, A., Johnson, C. L., Langlais, B., Lillis, R. J., Vervelidou, F., et al. (2018). The Mars 2020 Candidate Landing Sites: A Magnetic Field Perspective. Earth Space Sci. 5, 410–424. doi:10.1029/2018EA000420
Moores, J. E., Smith, P. H., Tanner, R., Schuerger, A. C., and Venkateswaran, K. J. (2007). The Shielding Effect of Small Scale Martian Surface Geometry on Ultraviolet Flux. Icarus 192, 417–433. doi:10.1016/j.icarus.2007.07.003
Moosavi, D., Wolovsky, D., Depompeis, A., Uher, D., Lennington, D., Bodden, R., et al. (2021). The Effects of Spaceflight Microgravity on the Musculoskeletal System of Humans and Animals, with an Emphasis on Exercise as a Countermeasure: a Systematic Scoping Review. Physiol. Res. 70, 119–151. doi:10.33549/physiolres.934550
Mormont, M. C., Waterhouse, J., Bleuzen, P., Giacchetti, S., Jami, A., Bogdan, A., et al. (2000). Marked 24-h Rest/activity Rhythms Are Associated with Better Quality of Life, Better Response, and Longer Survival in Patients with Metastatic Colorectal Cancer and Good Performance Status. Clin. Cancer Res. 6, 3038–3045. PMID: 10955782.
Müller, N. A., Wijnen, C. L., Srinivasan, A., Ryngajllo, M., Ofner, I., Lin, T., et al. (2016). Domestication Selected for Deceleration of the Circadian Clock in Cultivated Tomato. Nat. Genet. 48, 89–93. doi:10.1038/ng.3447
Mullineaux, C. W. (2001). How Do Cyanobacteria Sense and Respond to Light? Mol. Microbiol. 41, 965–971. doi:10.1046/j.1365-2958.2001.02569.x
NASA (2020). NASA. https://nssdc.gsfc.nasa.gov/planetary/factsheet/marsfact.html (Accessed November 27, 2021).
Neumann, M. F. (1988). Is There Any Influence of Magnetic or Astrophysical fields on the Circadian Rhythm of Honeybees? Behav. Ecol. Sociobiol. 23, 389–393. doi:10.1007/BF00303713
Oluwafemi, F. A., Abdelbaki, R., Lai, J. C., Mora-Almanza, J. G., and Afolayan, E. M. (2021). A Review of Astronaut Mental Health in Manned Missions: Potential Interventions for Cognitive and Mental Health Challenges. Life Sci. Space Res. 28, 26–31. doi:10.1016/j.lssr.2020.12.002
Ouyang, Y., Andersson, C. R., Kondo, T., Golden, S. S., and Johnson, C. H. (1998). Resonating Circadian Clocks Enhance Fitness in Cyanobacteria. Proc. Natl. Acad. Sci. U S A. 95, 8660–8664. doi:10.1073/pnas.95.15.8660
Palmer, J. D. (2002). The Living Clok: The Orchestrator of Biological Rhythms. Oxord: Oxord University Press, Inc.
Parihar, V. K., Allen, B., Tran, K. K., Macaraeg, T. G., Chu, E. M., Kwok, S. F., et al. (2015). What Happens to Your Brain on the Way to Mars. Sci. Adv. 1, e1400256. doi:10.1126/sciadv.1400256
Pavy Le-Traon, A., and Roussel, B. (1993). Sleep in Space. Acta Astronaut 29, 945–950. doi:10.1016/0094-5765(93)90014-n
Pittendrigh, C. S., and Minis, D. H. (1972). Circadian Systems: Longevity as a Function of Circadian Resonance in Drosophila melanogaster. Proc. Natl. Acad. Sci. U S A. 69, 1537–1539. doi:10.1073/pnas.69.6.1537
Pregueiro, A. M., Liu, Q., Baker, C. L., Dunlap, J. C., and Loros, J. J. (2006). The Neurospora Checkpoint Kinase 2: a Regulatory Link between the Circadian and Cell Cycles. Science 313, 644–649. doi:10.1126/science.1121716
Proietto, M., Bianchi, M. M., Ballario, P., and Brenna, A. (2015). Epigenetic and Posttranslational Modifications in Light Signal Transduction and the Circadian Clock in Neurospora Crassa. Int. J. Mol. Sci. 16, 15347–15383. doi:10.3390/ijms160715347
Qin, S., Yin, H., Yang, C., Dou, Y., Liu, Z., Zhang, P., et al. (2016). A Magnetic Protein Biocompass. Nat. Mater. 15, 217–226. doi:10.1038/nmat4484
Raible, F., Takekata, H., and Tessmar-Raible, K. (2017). An Overview of Monthly Rhythms and Clocks. Front. Neurol. 8, 189. doi:10.3389/fneur.2017.00189
Refinetti, R. (2016). Circadian Physiology. Boca Raton, Florida, USA: CRC Press, 328–330. doi:10.3389/fneur.2017.00189
Rensing, L., and Ruoff, P. (2002). Temperature Effect on Entrainment, Phase Shifting, and Amplitude of Circadian Clocks and its Molecular Bases. Chronobiol. Int. 19, 807–864. doi:10.1081/cbi-120014569
Saei, A. A., and Barzegari, A. (2012). The Microbiome: the Forgotten Organ of the Astronaut's Body-Pprobiotics beyond Terrestrial Limits. Future Microbiol. 7, 1037–1046. doi:10.2217/fmb.12.82
Scheer, F. A., Wright, K. P., Kronauer, R. E., and Czeisler, C. A. (2007). Plasticity of the Intrinsic Period of the Human Circadian Timing System. PLoS ONE 2, e721. doi:10.1371/journal.pone.0000721
Scheiermann, C., Kunisaki, Y., and Frenette, P. S. (2013). Circadian Control of the Immune System. Nat. Rev. Immunol. 13, 190–198. doi:10.1038/nri3386
Schneider, T., Thalau, H. P., and Semm, P. (1994). Effects of Light or Different Earth-Strength Magnetic fields on the Nocturnal Melatonin Concentration in a Migratory Bird. Neurosci. Lett. 168, 73–75. doi:10.1016/0304-3940(94)90419-7
Selsis, F., Kaltenegger, L., and Paillet, J. (2008). Terrestrial Exoplanets: Diversity,habitability and Characterization. Phys. Scr. T130, 014032. doi:10.1088/0031-8949/2008/T130/014032
Semm, P., Schneider, T., and Vollrath, L. (1980). Effects of an Earth-Strength Magnetic Field on Electrical Activity of Pineal Cells. Nature 288, 607–608. doi:10.1038/288607a0
Sgobba, T., and Schlacht, I. L. (2018). Chapter 15 - Habitability and Habitat Design in Space Safety and Human Performance. Amsterdam: Elsevier. doi:10.1016/B978-0-08-101869-9.00015-7
Sharma, V. K. (2003). Adaptive Significance of Circadian Clocks. Chronobiol. Int. 20, 901–919. doi:10.1081/cbi-120026099
Shi, Y. S., Jing, X. L., Tong, F. Z., Hu, Y., Sun, H. Y., Tian, L. P., et al. (2018). Research on Adaptive Process of Crew’s Affect and Cognition during 4-person 180-day CELSS Integrated Experiment. Space Med. Med. Eng. 31, 414–419. doi:10.16289/j.cnki.1002-0837.2018.04.004
Shimmura, T., Ohashi, S., and Yoshimura, T. (2015). The Highest-Ranking Rooster Has Priority to Announce the Break of Dawn. Sci. Rep. 5, 11683. doi:10.1038/srep11683
Siddiqui, R., Akbar, N., and Khan, N. A. (2021). Gut Microbiome and Human Health under the Space Environment. J. Appl. Microbiol. 130, 14–24. doi:10.1111/jam.14789
Solovev, I., Dobrovolskaya, E., Shaposhnikov, M., Sheptyakov, M., and Moskalev, A. (2019). Neuron-specific Overexpression of Core Clock Genes Improves Stress-Resistance and Extends Lifespan of Drosophila melanogaster. Exp. Gerontol. 117, 61–71. doi:10.1016/j.exger.2018.11.005
Song, C., Wang, J., Kim, B., Lu, C., Zhang, Z., Liu, H., et al. (2018). Insights into the Role of Circadian Rhythms in Bone Metabolism: A Promising Intervention Target? Biomed. Res. Int. 2018, 9156478. doi:10.1155/2018/9156478
Sulzman, F. M., Ellman, D., Fuller, C. A., Moore-Ede, M. C., and Wassmer, G. (1984). Neurospora Circadian Rhythms in Space: a Reexamination of the Endogenous-Exogenous Question. Science 225, 232–234. doi:10.1126/science.11540800
Swanson, C. M., Kohrt, W. M., Buxton, O. M., Everson, C. A., Wright, K. P., Orwoll, E. S., et al. (2018). The Importance of the Circadian System & Sleep for Bone Health. Metabolism 84, 28–43. doi:10.1016/j.metabol.2017.12.002
Tafforin, C. (2015). Confinement vs. Isolation as Analogue Environments for Mars Missions from a Human Ethology Viewpoint. Aerosp. Med. Hum. Perform. 86, 131–135. doi:10.3357/AMHP.4100.2015
Thaiss, C. A., Zeevi, D., Levy, M., Zilberman-Schapira, G., Suez, J., Tengeler, A. C., et al. (2014). Transkingdom Control of Microbiota Diurnal Oscillations Promotes Metabolic Homeostasis. Cell 159, 514–529. doi:10.1016/j.cell.2014.09.048
Turek, F. W., and Gillette, M. U. (2004). Melatonin, Sleep, and Circadian Rhythms: Rationale for Development of Specific Melatonin Agonists. Sleep Med. 5, 523–532. doi:10.1016/j.sleep.2004.07.009
Udo, R., Hamada, T., Horikawa, K., Iwahana, E., Miyakawa, K., Otsuka, K., et al. (2004). The Role of Clock in the Plasticity of Circadian Entrainment. Biochem. Biophys. Res. Commun. 318, 893–898. doi:10.1016/j.bbrc.2004.04.113
Wan, G., Hayden, A. N., Iiams, S. E., and Merlin, C. (2021). Cryptochrome 1 Mediates Light-dependent Inclination Magnetosensing in Monarch Butterflies. Nat. Commun. 12, 771. doi:10.1038/s41467-021-21002-z
Wang, D., Zhang, L., Liang, X., Shen, H., Chen, X., Wan, Y., et al. (2014). “Space Meets Time: Impact of Gravity on Circadian/Diurnal Rhythms,” in A Sponsored Supplement to Science: Human Performance in Space - Advancing Astronautics Research in China, 15–17.
Welsh, D. K., Takahashi, J. S., and Kay, S. A. (2010). Suprachiasmatic Nucleus: Cell Autonomy and Network Properties. Annu. Rev. Physiol. 72, 551–577. doi:10.1146/annurev-physiol-021909-135919
Whiteway, J., Daly, M., Carswell, A., Duck, T., Dickinson, C., Komguem, L., et al. (2008). Lidar on the Phoenix mission to Mars. J. Geophys. Res. 113, E00A08. doi:10.1029/2007JE003002
Wilson, J. W., Ott, C. M., Höner zu Bentrup, K., Ramamurthy, R., Quick, L., Porwollik, S., et al. (2007). Space Flight Alters Bacterial Gene Expression and Virulence and Reveals a Role for Global Regulator Hfq. Proc. Natl. Acad. Sci. U S A. 104, 16299–16304. doi:10.1073/pnas.0707155104
Woelfle, M. A., Ouyang, Y., Phanvijhitsiri, K., and Johnson, C. H. (2004). The Adaptive Value of Circadian Clocks: an Experimental Assessment in Cyanobacteria. Curr. Biol. 14, 1481–1486. doi:10.1016/j.cub.2004.08.023
Woller, A., and Gonze, D. (2021). Circadian Misalignment and Metabolic Disorders: A Story of Twisted Clocks. Biology 10, 207. doi:10.3390/biology10030207
Wright, K. P., Hughes, R., Kronauer, R. E., Dijk, D. J., and Czeisler, C. A. (2001). Intrinsic Near-24-H Pacemaker Period Determines Limits of Circadian Entrain-Ment to a Wea Synchronizer in Humans. Proc. Natl. Acad. Sci. U S A. 98, 14027–14032. doi:10.1073/pnas.201530198
Yang, H., Tong, F., Ma, X., Huang, T., Gan, X., Zhang, Y., et al. (2021). A Case Study of Emotional Changes of One Volunteer under the 180-day Combinational Environment Using Natural Language Processing Tool. Space Med. Med. Eng. 34, 222–228. doi:10.16289/j.cnki.1002-0837.2021.03.006
Zhang, H., Liang, J., and Chen, N. (2020). Do Not Neglect the Role of Circadian Rhythm in Muscle Atrophy. Ageing Res. Rev. 63, 101155. doi:10.1016/j.arr.2020.101155
Zhang, H., Wang, Y., Zhang, Z., Zhang, L., Tang, C., Sun, B., et al. (2021). Alterations in the Activity and Sleep of Drosophila melanogaster under Simulated Microgravity. NPJ Microgravity 7, 27. doi:10.1038/s41526-021-00157-5
Zhang, L., Weng, W., and Guo, J. (2011). Posttranscriptional Mechanisms in Controlling Eukaryotic Circadian Rhythms. FEBS Lett. 585, 1400–1405. doi:10.1016/j.febslet.2011.03.018
Zhu, L., Wang, Q., Hu, Y., and Wang, F. (2019). The Circadian Gene Per1 Plays an Important Role in Radiation-Induced Apoptosis and DNA Damage in Glioma. Asian Pac. J. Cancer Prev. 20, 2195–2201. doi:10.31557/APJCP.2019.20.7.2195
Keywords: Mars, circadian clock, gravity, radiation, magnetic field, isolation, confinement, countermeasure
Citation: Luo R, Huang Y, Ma H and Guo J (2022) How to Live on Mars With a Proper Circadian Clock?. Front. Astron. Space Sci. 8:796943. doi: 10.3389/fspas.2021.796943
Received: 18 October 2021; Accepted: 01 December 2021;
Published: 17 January 2022.
Edited by:
Yiliang Li, University of Hong Kong, Hong Kong SAR, ChinaReviewed by:
Zhongchen Wu, Shandong University, Weihai, ChinaXinli Wei, Institute of Microbiology (CAS), China
Copyright © 2022 Luo, Huang, Ma and Guo. This is an open-access article distributed under the terms of the Creative Commons Attribution License (CC BY). The use, distribution or reproduction in other forums is permitted, provided the original author(s) and the copyright owner(s) are credited and that the original publication in this journal is cited, in accordance with accepted academic practice. No use, distribution or reproduction is permitted which does not comply with these terms.
*Correspondence: Jinhu Guo, Z3VvamluaHVAbWFpbC5zeXN1LmVkdS5jbg==