- Institute for Physical Science and Technology, University of Maryland, College Park, MD, United States
The early 1970s saw a new and surprising feature in the composition of solar energetic particles (SEPs), resonant enhancements up to 10,000-fold in the ratio 3He/4He that could even make 3He dominant over H in rare events. It was soon learned that these events also had enhancements in the abundances of heavier elements, such as a factor of ∼10 enhancements in Fe/O, which was later seen to be part of a smooth increase in enhancements vs. mass-to-charge ratio A/Q from H to Pb, rising by a factor of ∼1000. These events were also associated with streaming 10–100 keV electrons that produce type III radio bursts. In recent years we have found these “impulsive” SEP events to be accelerated in islands of magnetic reconnection from plasma temperatures of 2–3 MK on open field lines in solar jets. Similar reconnection on closed loops traps the energy of the particles to produce hot (>10 MK), bright flares. Sometimes impulsive SEP intensities are boosted by shock waves when the jets launch fast coronal mass ejections. No single theory yet explains both the sharp resonance in 3He and the smooth increase up to heavier elements; two processes seem to occur. Sometimes the efficient acceleration even exhausts the rare 3He in the source region, limiting its fluence.
Introduction
The solar energetic particle (SEP) events observed first (Forbush 1946) were the largest and most energetic examples we know, where GeV protons produce a shower of particles cascading through the atmosphere to ground level in excess of that from the galactic cosmic rays (GCRs). However, these ground-level events (GLEs) provided little information on the composition of the incoming beam.
Measurement of multiple element abundances in SEPs began when Fichtel and Guss (1961) used nuclear emulsion detectors on a 4-min sounding-rocket flight from Ft. Churchill, Manitoba to observe a sampling of elements with 6 ≤ Z ≤ 16. The principle elements up to the Fe abundance peak were observed in the next solar cycle by Bertsch et al. (1969) using the same technique. Resolution of He isotopes and the continuous time coverage needed to observe smaller events would only begin when detector telescopes were flown on satellites.
3He
The first clearly-enhanced abundance ratio of 3He/4He = (2.1 ± 0.4) × 10−2 was reported by Hsieh and Simpson (1970), perhaps 50 times the value seen in the corona or solar wind, although differences in the spectra of the He isotopes were noted here.
Nearly every scientist involved in the early study of SEPs had previous experience with GCRs. After acceleration by shock waves at supernovae, GCRs spend ∼107 years colliding with interstellar H to produce secondary 2H, 3He, and isotopes of Li, Be, and B. Thus the observation of 3He/4He of 2% by Hsieh and Simpson (1970) was immediately misinterpreted as evidence that the SEPs had traversed enough material to fragment some 4He into 3He, just like the GCRs. However, it was soon found that there were many events like that seen by Serlemitsos and Balasubrahmanyan (1975) with 3He/4He = 1.52 ± 0.10 but 3He/2H > 300. How could there be more 3He than 4He and yet no 2H? Such abundances were definitely not compatible with fragmentation. Subsequently, there were limits established on Be/O and B/O in SEP events that were found to be <2 × 10−4 (e.g., McGuire et al., 1979; Cook et al., 1984). Thus these 3He-rich events were not just an accident of fragmentation; they must involve a completely new resonance phenomenon.
Figure 1 shows a sample of 3He-rich events as we see them above 2 MeV amu−1. As event intensities increase it becomes possible to see rarer ion species. Event 1 has only pre-event background levels of H, O, and Fe and 4He barely appears. 3He/4He varies greatly in events and vs energy, often peaking in the region 1–10 MeV amu−1 (Mason, 2007), while Fe/O is much more stable once events are large enough to provide a measurable sample, so Fe/O is often used to define “impulsive” events (e.g., Reames et al., 2014a). As discussed below, very high-Z elements begin to appear in the larger impulsive events and 3He fluence may be limited by depletion of 3He ions in the source volume.
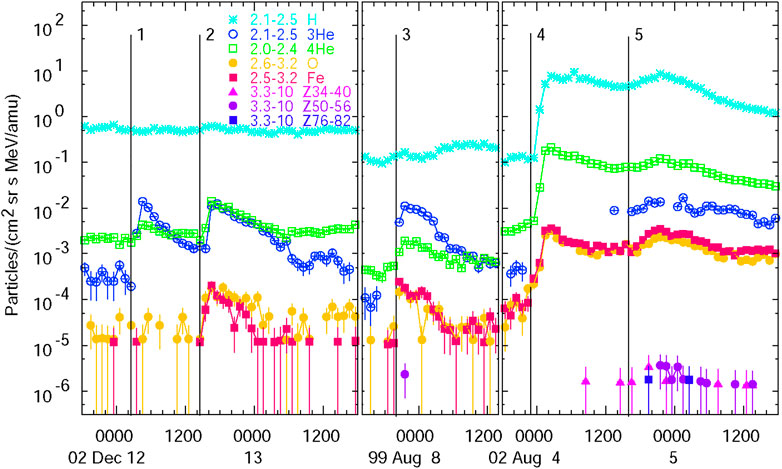
FIGURE 1. Ion intensities at the indicated MeV amu−1 are shown in a sample of 3He-rich or “impulsive” SEP events of increasing intensity. 3He exceeds 4He in Events 1 and 3. Fe and O are below background in Event 1, but Fe/O ≈ 1 in the remaining events. H is below background in Events 1–3. 3He/4He < 0.1 in Event 4 and is omitted because of possible resolution errors; it is ≈0.1 in Event 5 and high-Z heavy elements are beginning to appear (Reames and Ng, 2004). Other properties of the last four of these events are listed in Reames et al. (2014a).
Electrons
Some of the earliest information on SEPs in space was provided by the radio emission produced by ∼10–100 keV electrons streaming out from the Sun. The radio emission frequency varies as the square root of the local electron density which decreases sharply with distance from the Sun. In an early review article on solar radio bursts, Wild et al. (1963) distinguished type III radio bursts as produced by electrons streaming out rapidly along the magnetic field from a source at the Sun, and type II bursts which moved out at the slower ∼1,000 km s−1 speed of an interplanetary shock wave. Thus they saw two types of events: “electron events,” which produced the type III bursts, and “proton events,” accelerated at shock waves where associated electrons produce the type II burst as they are accelerated then overtaken, often at the flanks of the shock.
Early instruments flown on satellites measured 40 keV electrons associated with X-ray bursts at the Sun and type III radio bursts in space (Lin, 1970, 1974). These events were clearly different from the large proton events and some seemed to be “pure” electron events, i.e., lacking measurable ion intensities. The electrons events conformed to the picture presented by Wild et al. (1963).
It was not until 1985 that “pure” electron events turned out to be 3He-rich events (Reames et al., 1985). 3He-rich events were strongly associated with type III bursts, both the metric radio events, near the Sun (Reames et al., 1985), and the kilometric events below 2 MHz produced as the electrons continued out beyond ∼6 solar radii (Reames and Stone, 1986).
Abundances of Elements
Measurements of element abundances soon began to show periods when Fe/O ≥ 1 (Mogro-Compero and Simpson, 1972; Gloeckler et al., 1975), an enhancement by a factor of ∼10, relative to “coronal” abundances determined by the average of gradual SEP events (e.g., Reames, 1995a, 2014; Reames, 2021a), and such enhancements were shown to correspond with 3He-rich events (e.g., Mason et al., 1986), as seen in Figure 1. Reames (1988) looked at daily averages of SEPs to measure the overall distribution of abundances. He found a bimodal pattern with two branches of Fe/O. The branch near Fe/O ≈ 1 was 3He rich, electron rich, and proton (H/4He) poor relative to the branch near Fe/O ≈ 0.1. These bimodal abundances were helpful in distinguishing the physics of impulsive and gradual SEP events.
Reames et al. (1994) found that on average in 3He-rich events, the elements He, C, N, and O were unenhanced relative to coronal abundances, Ne, Mg, and Si were enhanced a factor of ∼2.5 and Fe was enhanced a factor of ∼7. This pattern would occur if He–O were fully ionized and Ne, Mg, and Si were in a stable state with two orbital electrons, which occurs in the temperature range 3–5 MK. This suggested that patterns of element abundance enhancements could be used to determine source plasma temperatures, since the pattern of Q values and A/Q, thus element enhancements, was dependent upon temperature (Reames et al., 2014b; Reames, 2018). The temperature variations among impulsive events turned out to be small, so the technique was more useful for gradual SEP events (Reames, 2016) with larger variations in temperature.
The extensive enhancement of very heavy elements was suggested early when Shirk and Price (1974) studied etch pits in a glass window of the Apollo 16 lunar command module and found (Z > 44)/Fe = 120+120–60 at 0.6 ≤ E ≤ 2.0 MeV amu−1 from a small SEP event in April 1972. Routine measurement that resolved elements above Fe with δZ/Z ∼ 2% began with the launch of the Wind spacecraft in November 1994. Reames (2000) found significant enhancements up to (70 ≤ Z ≤ 82), at 3.3 ≤ E ≤ 10 MeV amu−1, but only in impulsive SEP events. These measurements improved statistically with time (e.g., Reames and Ng, 2004) until Reames et al. (2014a) found the dependence of enhancements rising at the 3.64 ± 0.15 power of A/Q (at ≈3 MK) from He to Pb, with the (76 ≤ Z ≤ 82)/O interval enhanced by a factor of ≈900. Below 1 MeV amu−1, Mason et al. (2004) found enhancements varying as a power of A/Q of 3.26 and the interval 180 ≤ A ≤ 200 was enhanced a factor of ≈200. With the exception of 3He, enhancements are not strongly energy dependent.
When the power-law fits to abundance enhancements vs A/Q for elements Z ≥ 6 in an event were extrapolated down to H at A/Q = 1 (Reames, 2019b), there were small impulsive SEP events that seemed to fit the protons extremely well (called SEP1 events; Reames 2020) and some larger events with a large proton excess (called SEP2 events) as shown by the examples in Figure 2.
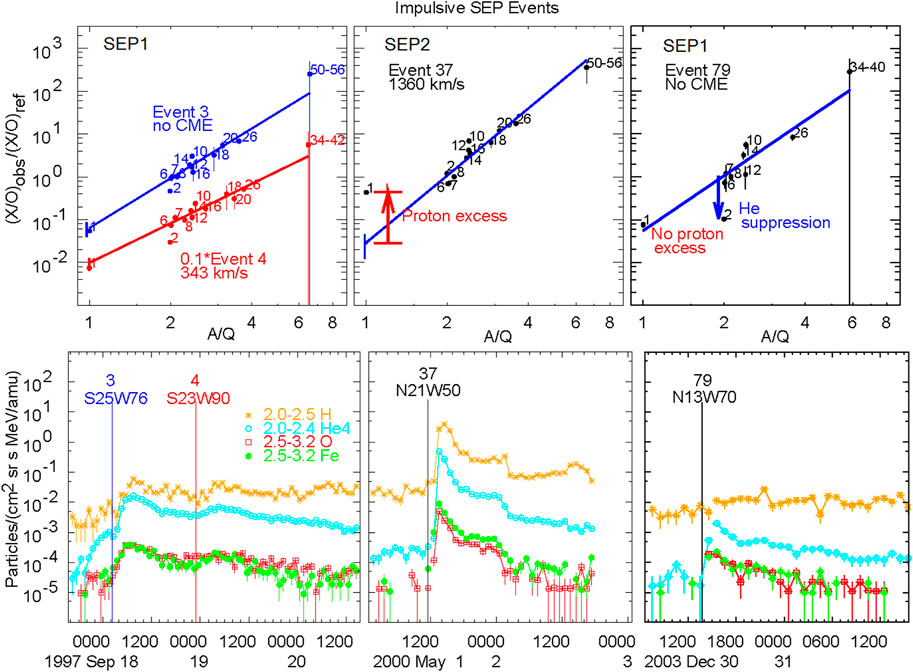
FIGURE 2. Lower panels show time histories of H, 4He, O and Fe, at the indicated MeV amu−1, for four impulsive SEP events, two small SEP1 events on the left and a larger SEP2 event in the center, and a 4He-poor SEP1 event on the right. Event numbers shown above source coordinates refer to the event list of Reames et al. (2014a). Power-law fits to the abundance enhancements, noted by Z, in each event, are shown in the upper panels and CME speeds are listed when CMEs are seen. The center Event 37 is an SEP2 class event because of its large proton excess noted. For the SEP1 events on the left and right, the protons abundances lie on the extrapolated power-law fits (Reames, 2019b, 2020).
We will see below that the event in the central panels of Figure 2 was one of the first impulsive events to be associated with a narrow CME produced by a solar jet (Kahler et al., 2001). These SEP2 events are generally more intense and were often associated with CMEs fast enough (1,360 km s−1 in this case) to drive shock waves that could reaccelerate the SEP1 impulsive suprathermal ions from the earlier magnetic reconnection as well as ions, at least H ions and occasionally He, from the ambient plasma. The correlation of SEP proton intensity with proton excess is shown in Figure 3 along with the suggested explanation of the excess.
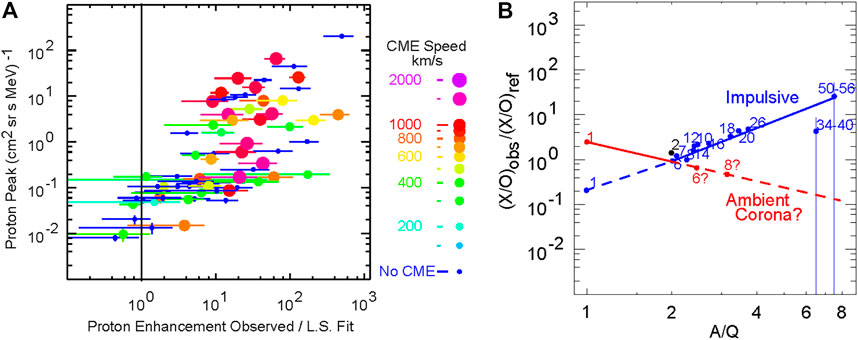
FIGURE 3. Panel (A) shows the peak proton intensity at 2–2.5 MeV vs the proton excess relative to the Z > 2 power-law fit for impulsive SEP events with the symbol size and color determined by CME speed as shown. Events with fast CMEs have proton excesses. Panel (B) suggests two possible contributions to the plot of enhancement vs A/Q for the shock-enhanced SEP2 impulsive events: reaccelerated impulsive SEP1 seed particles (blue) and accelerated ambient coronal seed particles (red). Note that a shock wave can accelerate outside the volume that contains the SEP1 seed particles. If the shock is very strong, the ambient corona could dominate and the event could become a shock-dominated gradual event.
At a temperature of 2.5–3 MK the elements 4He and C are both fully ionized with A/Z = 2, so 4He/C should represent the underlying coronal abundance, yet ∼6% of impulsive SEP events are extremely 4He poor (Reames 2019a) with 4He/C ≈ 15 vs an average 137 ± 8. One such event is shown as Event 79 in the right panel of Figure 2 where H, near background level, lies near the power-law fit of high-Z elements, but He lies well below it; another is shown as Event 3 in the central panel of Figure 1, where 4He is obviously much closer to O and Fe than it is in other events. It has been suggested that the high first ionization potential (FIP) of He, of 24.6 eV can delay its ionization and elevation into the corona, but Ne with FIP = 21.6 eV is unaffected. Any possible Z2/A effects from matter traversal would seem to suppress C more than 4He. What causes this occasional 4He poverty?
Note that we have studied variations of H/O and 4He/O separately in the above and not H/4He. Thus in Figure 2 we identify separate processes where H variation from the extrapolated power-law fit of Z > 2 depends upon the presence and activity of fast shock waves, while 4He (presumably) depends upon the especially high FIP of He. Studying the ratio H/4He would have blurred together the effects of these extremely different physical processes. This important feature was not initially obvious.
Isotope resolution has been extended as high as Fe (e.g., Leske et al., 1999, 2007). These measurements show variations in A/Q that supplement similar measurements that have been shown with power-law fits using multiple elements.
Ionization States
Luhn et al. (1984, 1987) provided important early measurements of ionic charge states, Q, up to Fe for energies 0.34–1.8 MeV amu−1. For large gradual events they found average values of QSi = 11.0 ± 0.3 and QFe = 14.1 ± 0.2, appropriate for a temperatures of ≈2 MK. However, for 3He-rich events they found QSi ≈ 14 and QFe = 20.5 ± 1.2, which meant either a source temperature of ≈10 MK, or stripping of the ions after acceleration. How could the average abundances of Ne, Mg, and Si get enhanced if they were all fully ionized with A/Q = 2 during acceleration, just like He, C, and O? This dilemma was finally resolved when DiFabio et al. (2008) found that the ionization states in the impulsive SEP events increased with ion speed, suggesting that the ions had come to ionization equilibrium during traversal of a small amount of material after acceleration. DiFabio et al. (2008) concluded that acceleration must have occurred near 1.5 solar radii.
Acceleration Theory
Most of the early attempts to explain the enhancement of 3He involved the preferential absorption of some form of wave energy, in resonance with the gyrofrequency of 3He, to produce selective preheating that would enhance the tail of thermal distribution of 3He so as to inject more ions into some unspecified acceleration mechanism. Ibragimov and Kocharov (1977) and Kocharov and Kocharov (1978, 1984) were first, considering ion-sound wave heating, but Weatherall (1984) pointed out that this could not account for all abundances. Fisk (1978) and Varvoglis and Papadopoulis (1983) suggested selective heating by absorption of electrostatic ion cyclotron waves. Winglee (1989) considered the ion-ion streaming instability to enhance heavy ions, and Riyopoulos (1991) considered electrostatic two-ion (H–4He) hybrid waves.
Temerin and Roth (1992) considered the ubiquitous associated streaming electrons that produced electromagnetic ion cyclotron (EMIC) waves that were adequate to actually resonantly accelerate the 3He that absorbed the waves while mirroring in the magnetic field, in analogy with “ion conics” seen in the Earth’s aurorae. Roth and Temerin (1997) suggested that heavier ions were enhanced through their second harmonic, but there was no smooth power law in A/Q. Miller et al. (1993a, b) considered the effects of other electron-beam-generated wave modes and Steinacker et al. (1997) considered warm-plasma broadening of spectral lines to produce a “He valley” of wave absorption.
Litvinenko (1996) considered effects of EMIC waves and Coulomb energy losses on the 3He spectrum and Liu et al. (2004, 2006) were able to fit the complex spectra of 3He and 4He with a model of stochastic acceleration by a power-law spectrum of plasma-wave turbulence. Roughly speaking, as the ions are accelerated to higher energy, the 3He begins to form a distinct energy peak as the 3He in the source volume becomes depleted, while the 4He does not.
Separately, explanation of the power-law dependence of enhancements on A/Q was first found by Drake et al. (2009) in particle-in-cell simulations of collapsing islands of magnetic reconnection. Ions are Fermi accelerated as they scatter back and forth from the ends of the collapsing islands. A similar process is found to accelerate electrons (Arnold et al., 2021) and its efficiency depends upon the strength of the out-of-plane guide field. However, 3He has not been discussed at all in this process, although there would seem to be abundant opportunities for mirroring ions to absorb waves.
There is evidence that the contribution of 3He is often saturated, i.e., all the 3He in the accelerating volume is actually accelerated. Reames (1999) estimated the total number of energetic 3He ions integrated over energy, space, and time and found it could be comparable with the number that exist in a typical flare volume. Subsequently, (Ho et al., 2005; Ho et al., 2019; Petrosian et al., 2009) found that this effect limited the maximum possible fluence of 3He. It has been suggested (Kahler, private communication) that comparing the calculated number of SEP 3He with the calculated number of 3He in the observed volume of a jet or reconnection region, on an event-by-event basis, might help determine where and what fraction of the 3He is accelerated. Is there enough 3He in the reconnection volume or is it accelerated throughout the jet as the Temerin and Roth (1992) model may suggest? Can anything be said about possible second-harmonic acceleration of some heavy ions like Fe, or the rare, low-energy resonant peaks of Si or S (Mason et al., 2016), for which the low-energy spectra roll over like that of 3He? Is SEP output bounded by the reconnection volume? Accelerating volumes are limited in impulsive SEPs while, in contrast, in gradual SEP events, source shock waves of huge area sweep out extensive volumes.
Flares, CMEs, Shocks, and Jets
Reconnection of the solar magnetic field drives nearly all solar activity, and flares provide a ubiquitous visible marker of magnetically trapped heating from that reconnection. Perhaps it is not surprising that the earliest evidence of GLEs was associated with obvious, bright flares, but this association was taken much too literally.
Radio data were the first to distinguish two acceleration mechanisms for SEPs in space (Wild et al., 1963), type III bursts that associate with electrons streaming from open magnetic reconnection in impulsive SEP events, and type II bursts that associate with shock acceleration and large gradual SEP events, but, unfortunately, the radio evidence was largely ignored in early SEP history.
After CMEs were identified and their observation became common, Kahler et al. (1984) found a 96% correlation between large SEP events and fast, wide CMEs. Shock waves driven by the CMEs could explain the extremely broad longitude span of the events. Mason et al. (1984) found that the minimal rigidity dependences of abundance variations across longitude were inconsistent with a point source origin and they discussed large-scale shock acceleration that they labelled LSSA. The importance of CMEs and shocks was not taken seriously by some flare enthusiasts until Gosling (1993, 1994) review article entitled “The solar flare myth” was published. This article was then found to “wage an assault on the last 30 years of solar-flare research” (Zirin, 1994) even though most published “solar-flare research” ignored SEPs entirely. However, the importance of CMEs and the existence of two mechanisms of SEP acceleration began to be recognized (Reames, 1988, 1995b, 1999, 2013, 2015, 2020, 2021a, 2021b; Zank et al., 2000, 2007; Kahler, 2001; Cliver et al., 2004; Lee, 2005; Gopalswamy et al., 2012; Lee et al., 2012; Mewaldt et al., 2012; Desai and Giacalone, 2016; Kouloumvakos et al., 2019).
For a brief interval, distinguishing impulsive and gradual SEP events seemed simple. Impulsive events were 3He-rich and gradual events were not. Then Mason et al. (1999) found a small but significant enhancement of 3He in a large SEP event that would be called gradual in all other respects. It became clear that shock waves in gradual SEP events could reaccelerate residual suprathermal ions left over from previous impulsive SEP events. In fact these pre-accelerated ions might be preferred in some cases, as in quasi-perpendicular shocks when ions needed to overtake the shock from downstream (Tylka et al., 2001, 2005; Tylka and Lee, 2006). In fact, it became evident that large pools of 3He-rich, Fe-rich suprathermal ions were extremely common, and available for shocks to traverse (Richardson et al., 1990; Desai et al., 2003; Wiedenbeck et al., 2008; Bučík et al., 2014, 2015; Chen et al., 2015). Whenever there was no large SEP event in progress the default suprathermal ion abundances below ∼1 MeV amu−1 seemed to be 3He-rich and Fe-rich, suggesting a large number of small unresolved jets (nanojets?) could generate SEPs faster than the solar wind could sweep them away. Thus, for 24% of gradual events, the Z ≥ 2 elements are dominated by reaccelerated ions from impulsive events, called SEP3 events (Reames, 2020), and in 69% of gradual events the shock predominantly accelerates ions, even Z > 2 ions, from the ambient coronal plasma (SEP4 events; Reames, 2020a; Reames, 2021a; Reames, 2021b).
Impulsive SEP events were small and difficult to associate with coronal features, but Kahler et al. (2001) were able to associate several of the larger impulsive SEP events with narrow CMEs. Figure 4 shows a narrow (54⁰), fast (1,360 km s−1) CME from the impulsive event of May 1, 2000 SEP, seen by the Solar and Heliospheric Observatory (SOHO; https://sohowww.nascom.nasa.gov/), for which SEPs were shown in the central panel of Figure 2. Such CMEs had been associated with type III bursts and solar jets (Shimojo and Shibata, 2000). Unlike flares, jets involve magnetic reconnection on open field lines so the SEPs (and the CMEs) easily escape. Tracking of impulsive SEPs back to the Sun led to jets, often on the boundary between active regions and coronal holes (Wang et al., 2006; Nitta et al., 2006, 2015). However, onset times are poorly defined in small SEP events, making associations with type III bursts, etc. more difficult. Reconnection is often triggered by large-scale waves moving across the corona (Bučík et al., 2016). It is now possible to consider the nature of the associated jets directly for many 3He-rich events (Bučík et al., 2018a, 2018b, 2021; see review; Bučík, 2020). It has even been possible to compare the temperatures derived from the extreme ultraviolet (EUV) images of coronal source regions of 24 solar jets associated with 3He-rich events (Bučík et al., 2021) with the abundance-derived temperatures from SEPs (Reames et al., 2014b).
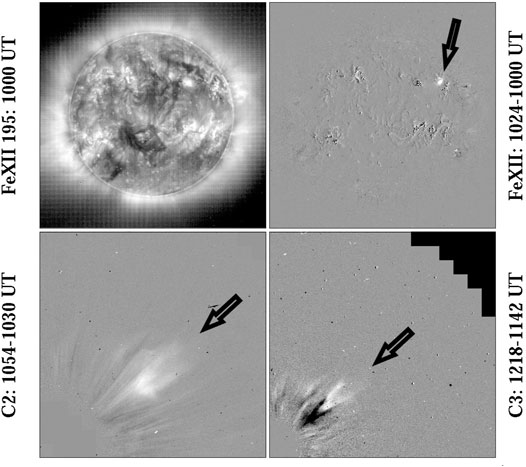
FIGURE 4. Images of the impulsive SEP event of May 1, 2000 (see also Figure 2) are: upper left a full disk SOHO/EIT image at 195 Å before the flare, upper right subtracted EIT image at 195 Å with arrow showing new small white source in the NW quadrant, lower left subtracted image of NW quadrant of SOHO/LASCO/C2 coronagraph 2–6 RS with arrow showing narrow CME, lower right subtracted NW quadrant of C3 image 4–30 RS with arrow showing narrow CME (Kahler et al., 2001). Tracking and magnetic configuration for this event are shown by Nitta et al. (2006) and by Wang et al. (2006).
However, impulsive SEP events are not necessarily derived purely from magnetic reconnection; the CMEs from these jets are often fast enough to drive shock waves which can reaccelerate SEPs from the reconnection as is certainly the case for the 1,360 km s−1 CME in the May 1, 2000 event shown in Figure 4. It may be possible to distinguish the pure (SEP1) events from those reaccelerated by a shock (SEP2) from the proton excess produced in the latter events as shown in Figure 3.
A sketch showing a jet formed by newly emerging magnetic field is shown in Figure 5. When the emerging field has opposite polarity from that of the preexisting field, reconnection takes place, not at a single point but in a series of “islands.” In realistic jets, the opposing fields rarely exactly cancel, but rather leave an out-of-plane, residual “guide field.” Particle acceleration occurs as particles, mainly electrons (Arnold et al., 2021), are Fermi-accelerated as they pitch-angle scatter in the evolving fields. As the SEPs and CME plasma are ejected on open field lines at the upper right in Figure 5, newly closing loops capture some SEPs in the lower left region labeled “flare” where they deposit their energy as heat. While much of the jet, including the SEPs, retain temperatures of 2–3 MK and emit EUV (Reames et al., 2014b; Bučík et al., 2021), the “flare” region with its captured SEP energy heats to 10–20 MK and emits X-rays. These X-rays often provide the source location. It is important to realize that the presence of the X-rays does not define the nature of the SEPs in space; both environments coexist since the reconnection that opens some field lines closes others. Some day someone will be able to provide us with an overlay of X-ray and EUV images that will map details of these source regions. As reconnection events become larger and more complex, both X-ray and EUV regions increase, contributing to a “big flare syndrome” (Kahler 1982), i.e., a misleading correlation between X-rays and the SEPs in space.
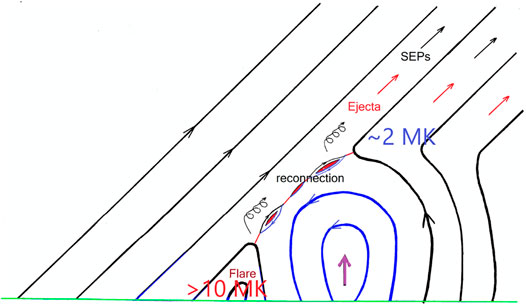
FIGURE 5. A jet is produced when newly emerging magnetic flux (blue) reconnects with oppositely directed field (black) in the red region. This reconnection region is not a uniform surface but forms multiple islands of reconnection. Energetic particles and plasma can escape toward the upper right and a newly-enclosed flaring region labeled “Flare” forms at the lower left that is heated by trapping SEPs. Real jets can be much more complex, involving twisted fields, etc.
What about solar flares? There is evidence from measurements of Doppler-broadened γ-ray lines that the ions accelerated in large solar flares are 3He-rich (Mandzhavidze et al., 1999; Murphy et al., 2016) and Fe-rich (Murphy et al., 1991), just like the impulsive SEPs from jets that we see in space. The SEPs accelerated on closed loops, dominated by electrons, soon scatter into the loss cone and plunge into the denser corona below, scattering against ions to produce X-ray bremstrahlung, and heating the plasma which expands back up into the loops creating a hot (>10 MK), bright flare. SEPs from jets are not hot (∼2–3 MK; Reames et al., 2014b; Bučík et al., 2021) because the SEPs and the CME plasma escape. Flares exist precisely because their energy is magnetically trapped and can only escape as heat, light, or neutral particles. Reconnection of closed fields with other closed fields cannot produce open fields, except when those fields are eventually carried outward by a CME.
Shock waves, driven by fast, wide CMEs are the basis of gradual SEP events. However, the narrow (<60⁰) CMEs emitted from solar jets are also fast enough to drive significant shocks, such as the 1,360 km s−1, 54⁰ width CME in the May 1, 2000 events discussed above. Yet, often, these fast, narrow CMEs from jets lack type II radio bursts that can signify shock acceleration, and the CME’s contribution to SEP acceleration may be questioned (e.g., Bronarska et al., 2018; Kahler et al., 2019). At quasi-parallel shock waves, ions are accelerated by scattering back and forth across the shock against Alfvén waves (often self-generated), but non-relativistic electrons cannot resonate with Alfvén waves, so they can only be accelerated in the VS x B electric field in more quasi-perpendicular regions of the shock. A big wide hemispherical shock surely has some regions where type II electrons get accelerated, while a narrow CME may produce only quasi-parallel regions—accelerating few electrons but plenty of ions. The shock from the May 1, 2000 event may not accelerate enough electrons, but it surely accelerates more ions than any of the shocks we have been able to observe directly in situ with shock speeds as low as 300 km s−1 (Reames 2012). The one feature that seems to show the presence or absence of shock acceleration in impulsive SEP events is the proton excess shown in Figures 2, 3 above (Reames 2019b), but the response of electrons and protons can be completely different.
Some people continue to defined “flare” to include every energetic phenomenon on the Sun, including flares, jets, CMEs, shocks, and even the SEPs at 1 AU. That, of course, makes flare research the most important discipline of all, studying the cause of everything solar, by definition. This deliberately blurs the physics, elevating the importance of flares and diminishing that of CMEs; this was exactly Gosling (1993) objection to “The Solar Flare Myth.” Applying “flare” to everything is not only meaningless but helps no one understand any physics. Beginning with Carrington (1860), many of us still think of “the flare” as that localized sudden bright flash of white light, Hα, or X-ray emission driven because reconnection energy is trapped in closed magnetic loops on the Sun—an event recorded by the Solar Flare Patrol and documented by its timing, latitude, longitude, and C-M-X-scale soft X-ray intensity. These flares are limited in spatial extent. In contrast, the shock wave that accelerates SEPs in a large gradual event is a nominally hemispherical structure initially active from 2 to over 3 RS (Reames 2009a, b; Cliver et al., 2004), thus enclosing an accelerating volume perhaps ∼10 times the volume of the Sun itself—this is not a flare. Thus the source of SEPs has a major effect on their spatial distributions, among many other things, and lumping all possible sources together, to inflate the egos of a few flare researchers, is no help. please distinguish CMEs from flares in your publications.
This field began with a frequent assumption that all SEPs were somehow actually accelerated in flares. First we found that SEPs in gradual events were accelerated by CME-driven shocks instead. Then we found that the remaining impulsive SEPs in space came from jets. Now we find that it may be more correct to say that flares are caused by SEPs trapped on loops than the converse.
Author Contributions
All work on this article was performed by DR.
Conflict of Interest
The author declares that the research was conducted in the absence of any commercial or financial relationships that could be construed as a potential conflict of interest.
Publisher’s Note
All claims expressed in this article are solely those of the authors and do not necessarily represent those of their affiliated organizations, or those of the publisher, the editors and the reviewers. Any product that may be evaluated in this article, or claim that may be made by its manufacturer, is not guaranteed or endorsed by the publisher.
Acknowledgments
The author thanks Steve Kahler for helpful comments on this article.
References
Arnold, H., Drake, J., Swisdak, M., Guo, F., Dahlin, J., Chen, B., et al. (2021). Electron Acceleration during Macroscale Magnetic Reconnection. Phys. Rev. Lett. 126, 13. doi:10.1103/PhysRevLett.126.135101
Bertsch, D. L., Fichtel, C. E., and Reames, D. V. (1969). Relative Abundance of Iron-Group Nuclei in Solar Cosmic Rays. ApJ 157, L53. doi:10.1086/180383
Bronarska, K., Wheatland, M. S., Gopalswamy, N., and Michalek, G. (2018). Very Narrow Coronal Mass Ejections Producing Solar Energetic Particles. A&A 619, A34. doi:10.1051/0004-6361/201833237
Bučík, R., Innes, D. E., Mall, U., Korth, A., Mason, G. M., and Gómez-Herrero, R. (2014). Multi-Spacecraft Observations of Recurrent 3He-Rich Solar Energetic Particles. ApJ 786, 71. doi:10.1088/0004-637X/786/1/71
Bučík, R., Innes, D. E., Chen, N. H., Mason, G. M., Gómez-Herrero, R., and Wiedenbeck, M. E. (2015). Long-lived Energetic Particle Source Regions on the Sun. J. Phys. Conf. Ser. 642, 012002. doi:10.1088/1742-6596/642/1/012002
Bučík, R., Innes, D. E., Mason, G. M., and Wiedenbeck, M. E. (2016). Association of 3He-Rich Solar Energetic Particles With Large-Scale Coronal Waves. ApJ 833, 63. doi:10.3847/1538-4357/833/1/63
Bučík, R., Innes, D. E., Mason, G. M., Wiedenbeck, M. E., Gómez-Herrero, R., and Nitta, N. V. (2018a). 3He-rich Solar Energetic Particles in Helical Jets on the Sun. ApJ 852, 76. doi:10.3847/1538-4357/aa9d8f
Bučík, R., Wiedenbeck, M. E., Mason, G. M., Gómez-Herrero, R., Nitta, N. V., and Wang, L. (2018b). 3He Rich Solar Energetic Particles from Sunspot Jets. ApJ 869, L21. doi:10.3847/2041-8213/aaf37f
Bučík, R. (2020). 3He-Rich Solar Energetic Particles: Solar Sources. Space Sci. Rev. 216, 24. doi:10.1007/s11214-020-00650-5
Bučík, R., Mulay, S. M., Mason, G. M., Nitta, N. V., Desai, M. I., and Dayeh, M. A. (2021). Temperature in Solar Sources of 3He-Rich Solar Energetic Particles and Relation to Ion Abundances. ApJ 908, 243. doi:10.3847/1538-4357/abd62d
Carrington, R. C. (1859). Description of a Singular Appearance Seen in the Sun on September 1, 1859. Monthly Notices R. Astronomical Soc. 20, 13–15. doi:10.1093/mnras/20.1.13
Chen, N.-h., Bučík, R., Innes, D. E., and Mason, G. M. (2015). Case Studies of Multi-day 3He-Rich Solar Energetic Particle Periods. A&A 580, A16. doi:10.1051/0004-6361/201525618
Cliver, E. W., Kahler, S. W., and Reames, D. V. (2004). Coronal Shocks and Solar Energetic Proton Events. ApJ 605, 902–910. doi:10.1086/382651
Cook, W. R., Stone, E. C., and Vogt, R. E. (1984). Elemental Composition of Solar Energetic Particles. ApJ 279, 827. doi:10.1086/161953
Desai, M. I., Mason, G. M., Dwyer, J. R., Mazur, J. E., Gold, R. E., Krimigis, S. M., et al. (2003). Evidence for a Suprathermal Seed Population of Heavy Ions Accelerated by Interplanetary Shocks Near 1 AU. ApJ 588, 1149–1162. doi:10.1086/374310
Desai, M., and Giacalone, J. (2016). Large Gradual Solar Energetic Particle Events. Living Rev. Sol. Phys. 13. doi:10.1007/s41116-016-0002-5
DiFabio, R., Guo, Z., Möbius, E., Klecker, B., Kucharek, H., Mason, G. M., et al. (2008). Energy‐dependent Charge States and Their Connection with Ion Abundances in Impulsive Solar Energetic Particle Events. Astrophysical J. 687, 623–634. doi:10.1086/591833
Drake, J. F., Cassak, P. A., Shay, M. A., Swisdak, M., and Quataert, E. (2009). A Magnetic Reconnection Mechanism for Ion Acceleration and Abundance Enhancements in Impulsive Flares. ApJ 700, L16–L20. doi:10.1088/0004-637X/700/1/L16
Fichtel, C. E., and Guss, D. E. (1961). Heavy Nuclei in Solar Cosmic Rays. Phys. Rev. Lett. 6, 495–497. doi:10.1103/PhysRevLett.6.495
Forbush, S. E. (1946). Three Unusual Cosmic-Ray Increases Possibly Due to Charged Particles from the Sun. Phys. Rev. 70, 771–772. doi:10.1103/PhysRev.70.771
Gloeckler, G., Hovestadt, D., Vollmer, O., and Fan, C. Y. (1975). Unusual Emission of Iron Nuclei from the Sun. ApJ 200, L45. doi:10.1086/181893
Gopalswamy, N., Xie, H., Yashiro, S., Akiyama, S., Mäkelä, P., and Usoskin, I. G. (2012). Properties of Ground Level Enhancement Events and the Associated Solar Eruptions during Solar Cycle 23. Space Sci. Rev. 171, 23–60. doi:10.1007/s11214-012-9890-4
Gosling, J. T. (1993). The Solar Flare Myth. J. Geophys. Res. 98, 18937–18949. doi:10.1029/93JA01896
Gosling, J. T. (1994). Correction to "The Solar Flare Myth". J. Geophys. Res. 99, 4259. doi:10.1029/94JA00015
Ho, G. C., Roelof, E. C., and Mason, G. M. (2005). The Upper Limit on 3He Fluence in Solar Energetic Particle Events. ApJ 621, L141–L144. doi:10.1086/429251
Ho, G. C., Mason, G. M., and Allen, R. C. (2019). 3He-Rich Solar Energetic Particle Events with No Measurable 4He Intensity Increases. Sol. Phys. 294, 33. doi:10.1007/s11207-019-1420-z
Hsieh, K. C., and Simpson, J. A. (1970). Galactic ^{3}He above 10 MeV Per Nucleon and the Solar Contributions of Hydrogen and Helium. ApJ 162, L197. doi:10.1086/180653
Ibragimov, I. A., and Kocharov, G. E. (1977). Possible Mechanism for Enrichment of Solar Cosmic Rays by Helium-Three and Heavy Nuclei. 15th Int. Conf. Cosmic Rays (Plovdiv: Bulgarian Acad. Sciences) 11, 340.
Kahler, S. W. (1982). The Role of the Big Flare Syndrome in Correlations of Solar Energetic Proton Fluxes and Associated Microwave Burst Parameters. J. Geophys. Res. 87, 3439. doi:10.1029/JA087iA05p03439
Kahler, S. W., Sheeley, N. R., Howard, R. A., Koomen, M. J., Michels, D. J., McGuire, R. E., et al. (1984). Associations between Coronal Mass Ejections and Solar Energetic Proton Events. J. Geophys. Res. 89, 9683. doi:10.1029/JA089iA11p09683
Kahler, S. W. (2001). The Correlation between Solar Energetic Particle Peak Intensities and Speeds of Coronal Mass Ejections: Effects of Ambient Particle Intensities and Energy Spectra. J. Geophys. Res. 106, 20947–20955. doi:10.1029/2000JA002231
Kahler, S. W., Reames, D. V., and Sheeley, Jr., N. R. (2001). Coronal Mass Ejections Associated with Impulsive Solar Energetic Particle Events. ApJ 562, 558–565. doi:10.1086/323847
Kahler, S. W., Ling, A. G., and Gopalswamy, N. (2019). Are Solar Energetic Particle Events and Type II Bursts Associated with Fast and Narrow Coronal Mass Ejections?. Sol. Phys. 294, 134. doi:10.1007/s11207-019-1518-3
Kocharov, L. G., and Kocharov, G. E. (1984). 3He-rich Solar Flares. Space Sci. Rev. 38, 89. doi:10.1007/BF00180337
Kocharov, G. E., and Kocharov, L. G. (1978). “Present State of Experimental and Theoretical Investigations of Solar Events Enriched by Helium-3” in Proc. 10th Leningrad Sympos. on Cosmic Rays, Leningrad. Leningrad: A. F. Yoffe Phys.-Tech. Inst., 37.
Kouloumvakos, A., Rouillard, A. P., Wu, Y., Vainio, R., Vourlidas, A., Plotnikov, I., et al. (2019). Connecting the Properties of Coronal Shock Waves with Those of Solar Energetic Particles. ApJ 876, 80. doi:10.3847/1538-4357/ab15d7
Lee, M. A. (2005). Coupled Hydromagnetic Wave Excitation and Ion Acceleration at an Evolving Coronal/interplanetary Shock. Astrophys J. Suppl. S 158, 38–67. doi:10.1086/428753
Lee, M. A., Mewaldt, R. A., and Giacalone, J. (2012). Shock Acceleration of Ions in the Heliosphere. Space Sci. Rev. 173, 247–281. doi:10.1007/s11214-012-9932-y
Leske, R. A., Mewaldt, R. A., Cohen, C. M. S., Cummings, A. C., Stone, E. C., Wiedenbeck, M. E., et al. (1999). Event-to-event Variations in the Isotopic Composition of Neon in Solar Energetic Particle Events. Geophys. Res. Lett. 26, 2693–2696. doi:10.1029/1999GL900561
Leske, R. A., Mewaldt, R. A., Cohen, C. M. S., Cummings, A. C., Stone, E. C., Wiedenbeck, M. E., et al. (2007). Solar Isotopic Composition as Determined Using Solar Energetic Particles. Space Sci. Rev. 130, 195–205. doi:10.1007/s11214-007-9185-3
Lin, R. P. (1970). The Emission and Propagation of ∼40 KeV Solar Flare Electrons. Sol. Phys. 12, 266. doi:10.1007/BF00227122
Lin, R. P. (1974). Non-relativistic Solar Electrons. Space Sci. Rev. 16, 189. doi:10.1007/BF00240886
Litvinenko, Y. E. (1996). “On the Formation of the Helium-3 Spectrum in Impulsive Solar Flares,” in High Energy Solar Physics, AIP Conf. Proc. 374. Editors R. Ramaty, N. Mandzhavidze, and X.-M. Hua (Woodbury, NY: AIP Press), 498. doi:10.1063/1.50985
Liu, S., Petrosian, V., and Mason, G. M. (2004). Stochastic Acceleration of 3He and 4He by Parallel Propagating Plasma Waves. ApJ 613, L81–L84. doi:10.1086/425070
Liu, S., Petrosian, V., and Mason, G. M. (2006). Stochastic Acceleration of 3He and4He in Solar Flares by Parallel‐propagating Plasma Waves: General Results. ApJ 636, 462–474. doi:10.1086/497883
Luhn, A., Klecker, B., Hovestadt, D., Gloeckler, G., Ipavich, F. M., Scholer, M., et al. (1984). Ionic Charge States of N, Ne, Mg, Si and S in Solar Energetic Particle Events. Adv. Space Res. 4, 161–164. doi:10.1016/0273-1177(84)90307-7
Luhn, A., Klecker, B., Hovestadt, D., and Moebius, E. (1987). The Mean Ionic Charge of Silicon in He-3-Rich Solar Flares. ApJ 317, 951. doi:10.1086/165343
Mandzhavidze, N., Ramaty, R., and Kozlovsky, B. (1999). Determination of the Abundances of Subcoronal 4He and of Solar Flare-Accelerated 3He and 4He from Gamma-ray Spectroscopy. Astrophys. J. 518, 918. doi:10.1086/307321
Mason, G. M., Gloeckler, G., and Hovestadt, D. (1984). Temporal Variations of Nucleonic Abundances in Solar Flare Energetic Particle Events. II - Evidence for Large-Scale Shock Acceleration. ApJ 280, 902. doi:10.1086/162066
Mason, G. M., Reames, D. V., von Rosenvinge, T. T., Klecker, B., and Hovestadt, D. (1986). The Heavy-Ion Compositional Signature in 3He-Rich Solar Particle Events. ApJ 303, 849. doi:10.1086/164133
Mason, G. M., Mazur, J. E., and Dwyer, J. R. (1999). 3He Enhancements in Large Solar Energetic Particle Events. Astrophys. J. Lett. 525, L133–L136. doi:10.1086/312349
Mason, G. M., Mazur, J. E., Dwyer, J. R., Jokipii, J. R., Gold, R. E., and Krimigis, S. M. (2004). Abundances of Heavy and Ultraheavy Ions in 3He‐rich Solar Flares. ApJ 606, 555–564. doi:10.1086/382864
Mason, G. M. (2007). 3He-Rich Solar Energetic Particle Events. Space Sci. Rev. 130, 231–242. doi:10.1007/s11214-007-9156-8
Mason, G. M., Nitta, N. V., Wiedenbeck, M. E., and Innes, D. E. (2016). Evidence for a Common Acceleration Mechanism for Enrichments of 3He And Heavy Ions in Impulsive Sep Events. ApJ 823, 138. doi:10.3847/0004-637X/823/2/138
McGuire, R. E., von Rosenvinge, T. T., and McDonald, F. B. (1979). A Survey of Solar Cosmic ray Composition. Proc. 16th Int. Cosmic Ray Conf, 5, 61..
Mewaldt, R. A., Looper, M. D., Cohen, C. M. S., Haggerty, D. K., Labrador, A. W., Leske, R. A., et al. (2012). Energy Spectra, Composition, and Other Properties of Ground-Level Events during Solar Cycle 23. Space Sci. Rev. 171, 97–120. doi:10.1007/s11214-012-9884-2
Miller, J., Viñas, A., and Reames, D. V. (1993a). Selective 3He and Fe Acceleration in Impulsive Solar Flares. 23rd Intl. Cosmic-ray Conf. (Calgary) 3, 13.
Miller, J., Viñas, A., and Reames, D. V. (1993b). Heavy Ion Acceleration and Abundance Enhancements in Impulsive Solar Flares. 23rd Intl. Cosmic-ray Conf. (Calgary) 3, 17.
Mogro-Campero, A., and Simpson, J. A. (1972). Enrichment of Very Heavy Nuclei in the Composition of Solar Accelerated Particles. ApJ 171, L5. doi:10.1086/180856
Murphy, R. J., Ramaty, R., Reames, D. V., and Kozlovsky, B. (1991). Solar Abundances from Gamma-ray Spectroscopy - Comparisons with Energetic Particle, Photospheric, and Coronal Abundances. ApJ 371, 793. doi:10.1086/169944
Murphy, R. J., Kozlovsky, B., and Share, G. H. (2016). Evidence for Enhanced3He in Flare-Accelerated Particles Based on New Calculations of the Gamma-ray Line Spectrum. ApJ 833, 196. doi:10.3847/1538-4357/833/2/196
Nitta, N. V., Reames, D. V., DeRosa, M. L., Liu, Y., Yashiro, S., and Gopalswamy, N. (2006). Solar Sources of Impulsive Solar Energetic Particle Events and Their Magnetic Field Connection to the Earth. ApJ 650, 438–450. doi:10.1086/507442
Nitta, N. V., Mason, G. M., Wang, L., Cohen, C. M. S., and Wiedenbeck, M. E. (2015). Solar Sources of 3He-Rich Solar Energetic Particle Events in Solar Cycle 24. ApJ 806, 235. doi:10.1088/0004-637X/806/2/235
Petrosian, V., Jiang, Y. W., Liu, S., Ho, G. C., and Mason, G. M. (2009). Relative Distributions of Fluences of 3 He and 4He In Solar Energetic ParticleS. ApJ 701, 1–7. doi:10.1088/0004-637X/701/1/1
Reames, D. V., von Rosenvinge, T. T., and Lin, R. P. (1985). Solar 3He-Rich Events and Nonrelativistic Electron Events - A New Association. ApJ 292, 716. doi:10.1086/163203
Reames, D. V., and Stone, R. G. (1986). The Identification of Solar He-3-Rich Events and the Study of Particle Acceleration at the Sun. ApJ 308, 902. doi:10.1086/164560
Reames, D. V. (1988). Bimodal Abundances in the Energetic Particles of Solar and Interplanetary Origin. ApJ 330, L71. doi:10.1086/185207
Reames, D. V., Meyer, J. P., and von Rosenvinge, T. T. (1994). Energetic-particle Abundances in Impulsive Solar Flare Events. ApJS 90, 649. doi:10.1086/191887
Reames, D. V. (1995a). Coronal Abundances Determined from Energetic Particles. Adv. Space Res. 15 (7), 41–51. doi:10.1016/0273-1177(94)00018-v
Reames, D. V. (1995b). Solar Energetic Particles: A Paradigm Shift. Rev. Geophys. 33, 585. doi:10.1029/95RG00188
Reames, D. V. (1999). Particle Acceleration at the Sun and in the Heliosphere. Space Sci. Rev. 90, 413–491. doi:10.1023/A:1005105831781
Reames, D. V. (2000). Abundances of Trans-iron Elements in Solar Energetic Particle Events. Astrophys. J. Lett. 540, L111–L114. doi:10.1086/312886
Reames, D. V., and Ng, C. K. (2004). Heavy‐Element Abundances in Solar Energetic Particle Events. ApJ 610, 510–522. doi:10.1088/0004-637X/723/2/128610.1086/421518
Reames, D. V. (2009a). Solar Release Times of Energetic Particles in Ground-Level Events. ApJ 693, 812–821. doi:10.1088/0004-637X/693/1/812
Reames, D. V. (2009b). Solar Energetic-Particle Release Times in Historic Ground-Level Events. ApJ 706, 844–850. doi:10.1088/0004-637X/706/1/844
Reames, D. V. (2012). Particle Energy Spectra at Traveling Interplanetary Shock Waves. ApJ 757, 93. doi:10.1088/0004-637X/757/1/93
Reames, D. V. (2013). The Two Sources of Solar Energetic Particles. Space Sci. Rev. 175, 53–92. doi:10.1007/s11214-013-9958-9
Reames, D. V. (2014). Element Abundances in Solar Energetic Particles and the Solar corona. Sol. Phys. 289, 977–993. doi:10.1007/s11207-013-0350-4
Reames, D. V., Cliver, E. W., and Kahler, S. W. (2014a). Abundance Enhancements in Impulsive Solar Energetic-Particle Events with Associated Coronal Mass Ejections. Sol. Phys. 289, 3817–3841. doi:10.1007/s11207-014-0547-1
Reames, D. V., Cliver, E. W., and Kahler, S. W. (2014b). Variations in Abundance Enhancements in Impulsive Solar Energetic-Particle Events and Related CMEs and Flares. Sol. Phys. 289, 4675–4689. doi:10.1007/s11207-014-0589-4
Reames, D. V. (2015). What Are the Sources of Solar Energetic Particles? Element Abundances and Source Plasma Temperatures. Space Sci. Rev. 194, 303–327. doi:10.1007/s11214-015-0210-7
Reames, D. V. (2016). Temperature of the Source Plasma in Gradual Solar Energetic Particle Events. Solar Phys. 291. doi:10.1007/s11207-016-0854-9
Reames, D. V. (2018). Abundances, Ionization States, Temperatures, and FIP in Solar Energetic Particles. Space Sci. Rev. 214, 61. doi:10.1007/s11214-018-0495-4
Reames, D. V. (2019a). Helium Suppression in Impulsive Solar Energetic-Particle Events. Sol. Phys. 294, 32. doi:10.1007/s11207-019-1422-x
Reames, D. V. (2019b). Hydrogen and the Abundances of Elements in Impulsive Solar Energetic-Particle Events. Sol. Phys. 294, 37. doi:10.1007/s11207-019-1427-5
Reames, D. V. (2020). Four Distinct Pathways to the Element Abundances in Solar Energetic Particles. Space Sci. Rev. 216, 20. doi:10.1007/s11214-020-0643-5
Reames, D. V. (2021a). Solar Energetic Particles. in Lec. Notes Phys 978. Second Edition (Cham, Switzerland: Springer Nature). doi:10.1007/978-3-030-66402-2
Reames, D. V. (2021b). Sixty Years of Element Abundance Measurements in Solar Energetic Particles. Space Sci. Rev. 217, 72. doi:10.1007/s11214-021-00845-4
Richardson, I. G., Reames, D. V., Wenzel, K.-P., and Rodriguez-Pacheco, J. (1990). Quiet-time Properties of Low-Energy (Less Than 10 MeV Per Nucleon) Interplanetary Ions during Solar Maximum and Solar Minimum. ApJ 363, L9. doi:10.1086/185853
Riyopoulos, S. (1991). Subthreshold Stochastic Diffusion with Application to Selective Acceleration of 3He in Solar Flares. ApJ 381, 578. doi:10.1086/170682
Roth, I., and Temerin, M. (1997). Enrichment of 3He and Heavy Ions in Impulsive Solar Flares. ApJ 477, 940–957. doi:10.1086/303731
Serlemitsos, A. T., and Balasubrahmanyan, V. K. (1975). Solar Particle Events with Anomalously Large Relative Abundance of 3He. ApJ 198, 195. doi:10.1086/153592
Shimojo, M., and Shibata, K. (2000). Physical Parameters of Solar X‐Ray Jets. ApJ 542, 1100–1108. doi:10.1086/317024
Shirk, E. K., and Price, P. B. (1974). Observation of Transiron Solar-Flare Nuclei in an Apollo 16 Command Module Window. ApJ 190, 695. doi:10.1086/152927
Steinacker, J., Meyer, J. P., Steinacker, A., and Reames, D. V. (1997). The Helium Valley: Comparison of Impulsive Solar Flare Ion Abundances and Gyroresonant Acceleration with Oblique Turbulence in a Hot Multi‐Ion Plasma. ApJ 476, 403–427. doi:10.1086/303589
Temerin, M., and Roth, I. (1992). The Production of 3He and Heavy Ion Enrichment in 3He-Rich Flares by Electromagnetic Hydrogen Cyclotron Waves. ApJ 391, L105. doi:10.1086/186408
Tylka, A. J., Cohen, C. M. S., Dietrich, W. F., Maclennan, C. G., McGuire, R. E., Ng, C. K., et al. (2001). Evidence for Remnant Flare Suprathermals in the Source Population of Solar Energetic Particles in the 2000 Bastille Day Event. Astrophys. J. Lett. 558, L59–L63. doi:10.1086/323344
Tylka, A. J., Cohen, C. M. S., Dietrich, W. F., Lee, M. A., Maclennan, C. G., Mewaldt, R. A., et al. (2005). Shock Geometry, Seed Populations, and the Origin of Variable Elemental Composition at High Energies in Large Gradual Solar Particle Events. ApJ 625, 474–495. doi:10.1086/429384
Tylka, A. J., and Lee, M. A. (2006). A Model for Spectral and Compositional Variability at High Energies in Large, Gradual Solar Particle Events. ApJ 646, 1319–1334. doi:10.1086/505106
Varvoglis, H., and Papadopoulos, K. (1983). Selective Nonresonant Acceleration of He-3(2+) and Heavy Ions by H(+) Cyclotron Waves. ApJ 270, L95. doi:10.1086/184077
Wang, Y. M., Pick, M., and Mason, G. M. (2006). Coronal Holes, Jets, and the Origin of 3He‐rich Particle Events. ApJ 639, 495–509. doi:10.1086/499355
Weatherall, J. (1984). Turbulent Heating in Solar Cosmic-ray Theory. ApJ 281, 468. doi:10.1086/162119
Wiedenbeck, M. E., Cohen, C. M. S., Cummings, A. C., de Nolfo, G. A., Leske, R. A., Mewaldt, R. A., et al. (2008). Persistent Energetic 3He in the Inner Heliosphere. Proc. 30th Int. Cosmic Ray Conf. (Mérida) 1, 91.
Wild, J. P., Smerd, S. F., and Weiss, A. A. (1963). Solar Bursts. Annu. Rev. Astron. Astrophys. 1, 291–366. doi:10.1146/annurev.aa.01.090163.001451
Winglee, R. M. (1989). Heating and Acceleration of Heavy Ions during Solar Flares. ApJ 343, 511. doi:10.1086/167726
Zank, G. P., Rice, W. K. M., and Wu, C. C. (2000). Particle Acceleration and Coronal Mass Ejection Driven Shocks: A Theoretical Model. J. Geophys. Res. 105, 25079–25095. doi:10.1029/1999JA000455
Zank, G. P., Li, G., and Verkhoglyadova, O. (2007). Particle Acceleration at Interplanetary Shocks. Space Sci. Rev. 130, 255–272. doi:10.1007/s11214-007-9214-2
Keywords: solar energetic particles, solar jets, shock waves, solar system abundances, magnetic reconnection
Citation: Reames DV (2021) Fifty Years of 3He-Rich Events. Front. Astron. Space Sci. 8:760261. doi: 10.3389/fspas.2021.760261
Received: 17 August 2021; Accepted: 13 September 2021;
Published: 27 September 2021.
Edited by:
Radoslav Bucik, Southwest Research Institute (SwRI), United StatesReviewed by:
George Ho, Johns Hopkins University, United StatesSiming Liu, Purple Mountain Observatory (CAS), China
Ilan Roth, University of California, Berkeley, United States
Copyright © 2021 Reames. This is an open-access article distributed under the terms of the Creative Commons Attribution License (CC BY). The use, distribution or reproduction in other forums is permitted, provided the original author(s) and the copyright owner(s) are credited and that the original publication in this journal is cited, in accordance with accepted academic practice. No use, distribution or reproduction is permitted which does not comply with these terms.
*Correspondence: Donald V. Reames, ZHZyZWFtZXNAZ21haWwuY29t