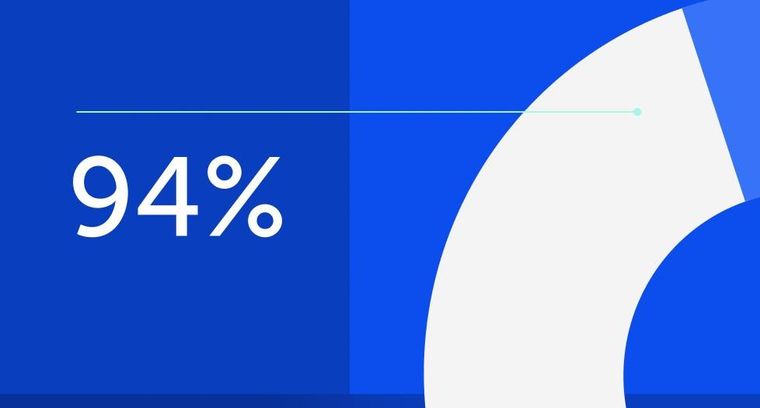
94% of researchers rate our articles as excellent or good
Learn more about the work of our research integrity team to safeguard the quality of each article we publish.
Find out more
REVIEW article
Front. Astron. Space Sci., 04 January 2022
Sec. Astrobiology
Volume 8 - 2021 | https://doi.org/10.3389/fspas.2021.747821
This article is part of the Research TopicBioregenerative Life-Support Systems for Crewed Missions to the Moon and MarsView all 26 articles
Bioregenerative life support systems (BLSS) are conceived of and developed so as to provide food sources for crewed missions to the Moon or Mars. The in situ resource utilization (ISRU) approach aims to reduce terrestrial input into a BLSS by using native regoliths and recycled organic waste as primary resources. The combination of BLSS and ISRU may allow sustainable food production on Moon and Mars. This task poses several challenges, including the effects of partial gravity, the limited availability of oxygen and water, and the self-sustaining management of resources. Lunar and Martian regoliths are not available on Earth; therefore, space research studies are conducted on regolith simulants that replicate the physicochemical properties of extra-terrestrial regoliths (as assessed in situ by previous missions). This review provides an overview of the physicochemical properties and mineralogical composition of commercially available Lunar and Martian regolith simulants. Subsequently, it describes potential strategies and sustainable practices for creating regolith simulants akin to terrestrial soil, which is a highly dynamic environment where microbiota and humified organic matter interact with the mineral moiety. These strategies include the amendment of simulants with composted organic wastes, which can turn nutrient-poor and alkaline crushed rocks into efficient life-sustaining substrates equipped with enhanced physical, hydraulic, and chemical properties. In this regard, we provide a comprehensive analysis of recent scientific works focusing on the exploitation of regolith simulant-based substrates as plant growth media. The literature discussion helps identify the main critical aspects and future challenges related to sustainable space farming by the in situ use and enhancement of Lunar and Martian resources.
Current scientific inventions and technological advancements may allow space travel and, in the far future, the development of bioregenerative life support systems (BLSS) on other celestial bodies (Zubrin and Wagner, 2011; NASA, 2018). From this perspective, the colonization of the Moon or Mars is closer to reality than it is to science fiction.
Studies focusing on various aspects of life on other celestial bodies have helped make it possible to contemplate extra-terrestrial colonization. These include studies on celestial bodies with a gravitational pull different from that on Earth (Hoson et al., 2000), the recycling of oxygen and water (Primm et al., 2018), and other issues related to sustainable food production in controlled environments or BLSS. The provision of terrestrial resources to permanent extra-terrestrial human settlements is not economically sustainable (Verseux et al., 2016), not only because of the high cost and resource/energy requirements, but also due to the difficulty and time needed to plan and execute launches (Llorente et al., 2018). Given the long-term nature of space missions and future space settlements, BLSS also need to be self-sustaining so as to reduce inputs from Earth and to deal with any challenges threatening the success of the missions. An efficient BLSS must be capable of purifying water, revitalizing the atmosphere, and producing food in a closed loop system (Menezes et al., 2015; Foing et al., 2018; Llorente et al., 2018). This can also be accomplished through in situ resource utilization (ISRU), which requires the use of native materials (Karl et al., 2018) and waste as primary resources (Menezes et al., 2015). Instead of relying on a closed loop, new materials found on site could be brought into the life support systems, thus making them sustainable and expandable.
Numerous studies have evaluated the feasibility of life on other planets. Some have conducted hydraulic and engineering tests to assess the practicality of building (Gertsch et al., 2008) and manufacturing (Chow et al., 2017; Karl et al., 2018), whereas others have experimented with microbial (Verseux et al., 2016; Kölbl et al., 2017) and plant growth (Gilrain et al., 1999; Kozyrovska et al., 2006; Wamelink et al., 2014) in an extra-terrestrial environment. Space farming based on local resource exploitation (Maggi and Pallud, 2010; Ramírez et al., 2019) is a promising strategy for food production (Ming and Henninger, 1989) on extra-terrestrial habitats, as it can allow water recycling, organic waste composting, and oxygen production or CO2 consumption (Verseux et al., 2016; Llorente et al., 2018). This would also reduce the launch mass from Earth and the waste generated by human settlement (food cost-cutting). Another important aspect is the psychological comfort plants can provide for astronauts during their long period of isolation (Nechitailo and Mashinsky, 1993; Ivanova et al., 2005; Marquit et al., 2008; Bates et al., 2009).
An ISRU approach for fresh food production is crucial to guarantee sustainability in extra-terrestrial BLSS. Using the local regolith as “soil” for plant growth would be a viable way to grow food, even though “extra-terrestrial soil” is very different from vital and fertile “terrestrial soil” (Certini et al., 2009; Juilleret et al., 2016; Certini et al., 2020). The appropriate term for the surficial unconsolidated fine mineral material on other planetary bodies is regolith, as it lacks living matter and is still very similar to the underlying parent rock. Soil taxonomy defines soil as “a natural body that comprised solids (minerals and organic matter), liquid, and gases that occur on the land surface, occupies space, and is characterized by one or both of the following: horizons, or layers, that are distinguishable from the initial material as a result of additions, losses, transfers, and transformations of energy and matter or the ability to support rooted plants in a natural environment” (Soil Survey Staff, 2014). Regolith does not have associated organic matter or a microbiome. In theory, regolith can be classified as soil if it has undergone the same processes that the Earth-based regolith undergoes to become soil (e.g., the presence of oxygen, the influences of wind and water, and activity by living organisms). Likewise, regolith can be defined as soil if it has undergone organic processes. Due to the lack of a standardized method for evaluating regolith efficacy, many published studies claiming to have assessed regolith for plant growth have used microbiome-contaminated regolith, which is in effect a soil.
The Lunar science community uses the word “soil” in an engineering geology sense, identifying “Lunar soil” with the finer-grained fraction of the unconsolidated material (regolith) on the Lunar surface (Heiken et al., 1991). A more complex and informative definition of extra-terrestrial soils (along with attempts of classification) has been provided (Certini et al., 2009; Certini et al., 2020). These native soils can be defined as the (bio)geochemically/physically altered material on the surface of a planetary body that encompasses surficial extra-terrestrial telluric deposits. According to this definition, the soil is a material that retains information about its environmental history, and whose formation does not require the presence of life. In this context, and considering the known geochemical features of extra-terrestrial regoliths, the surface deposits on planetary and other celestial bodies—such as Venus, Mars and Earth-Moon—should be considered soils in a pedological sense (Certini et al., 2009). Moreover, the chemical diversity across in situ and regional soils on Mars suggests the existence of many different soil types and processes (Certini et al., 2020). In this review, we frequently use the term “soil” when referring to the surface of the Moon and Mars as a potential crop substrate, and adopt this term when discussing the literature.
The Lunar regolith has been studied on the Moon, and has also been analyzed on Earth using samples brought back by the Apollo missions. The regolith on Mars has been analyzed by rovers and robotic spacecrafts. These studies have elucidated the physical and chemical properties of Lunar and Martian native soils. Nevertheless, there is only a minimal quantity of Lunar material on Earth that is closely guarded, and no samples have been brought back from Mars to date. Therefore, most commercial regolith simulants have been produced by closely replicating the specific physicochemical properties of extra-terrestrial surfaces. Most existing simulants were developed to address specific application fields, and although their chemical interactions or properties related to mechanical abrasion have been assessed to mitigate potential risks (Rickman et al., 2013), their agricultural properties have rarely been evaluated. Naturally, plant growth, morphology, and physiology on the Moon or other planets are expected to be greatly affected by the sterile and nutrient-poor nature of extra-terrestrial soil and the different gravitational and climatic conditions. However, these regolith simulants, although not sterile, may play an essential role in improving our understanding of the environmental phenomena on the Moon and Mars. They may also help solve potential problems related to the exploitation of Lunar and Martian regolith as plant growth substrates.
This review aims to provide a comprehensive overview of the potential for existing Martian and Lunar simulants to serve as substrates for growing crops in BLSS. First, we assess selected Lunar and Martian regolith simulants on the basis of their physicochemical and mineralogical properties. Second, we describe previously tested strategies and sustainable practices for using these simulants as plant growth media, with emphasis on the main critical aspects and challenges in deploying these systems. In this review, we consulted 74 scientific papers and 10 technical reports on Lunar and Martian regolith simulants published between 1970 and 2021. The main critical aspects of space agriculture are presumed to be related to nutrient availability, air and fluid movements in different gravitational conditions, and potentially toxic elements in the substrates. Potential future challenges include a lack of adequate knowledge about the extra-terrestrial environment and the development of best agronomic practices for the first space colony.
This review provides an overview of the petrographic/mineralogical compositions and bulk chemistry of Lunar and Martian regolith simulants developed over the last 3–4 decades, which are mostly available for research purposes. We included simulants listed in the Planetary Simulant Database (https://simulantdb.com/) of the Colorado School of Mines. Of these, we selected 30 simulants whose petrographic/mineralogical and chemical characteristics had been described in 27 scientific papers and included in the Planetary Simulant Database (Table 1). According to the Planetary Simulant Database classification scheme, the included simulants were divided into four categories: 11 Lunar Mare simulants (McKay et al., 1994; Kanamori et al., 1998; Sibille et al., 2006; Sueyoshi et al., 2008; Matsushima et al., 2009; Zheng et al., 2009; Stoeser et al., 2010b; He et al., 2010; Rahmatian and Metzger, 2010; van Susante and Dreyer, 2010; Suescun-Florez et al., 2015); 6 Lunar Highlands simulants (McKay et al., 1994; Battler and Spray, 2009; Li et al., 2009; Stoeser et al., 2010a; Zeng et al., 2010); 4 Lunar Dust simulants (Sueyoshi et al., 2008; Sun et al., 2017; Tang et al., 2017; Britt and Cannon, 2019; Cannon and Britt, 2019); and 9 Martian simulants (Zeng et al., 2015; Lee, 2017; Archer et al., 2018; Stevens et al., 2018; Cannon et al., 2019; Caporale et al., 2020; Clark et al., 2020).
TABLE 1. List of the simulants considered in the present work, divided by reference category. In detail the availability on the market and the reference analysis for chemical and mineralogical characteristics.
There is no universal simulant that comprehensively represents the mineralogy of the Lunar and Martian surfaces. Similar to the Earth’s crust, the surficial layers of the Moon and other planets show high heterogeneity and spatial variability. Therefore, it is difficult to create a simulant for every mineralogical combination or potential application. To develop a simulant, it is crucial to find terrestrial rocks with compositions and qualitative and quantitative mineralogical patterns similar to those of Lunar and Martian regoliths. Mineralogical assemblages can be modified to reproduce the general variability on the Moon and Mars. However, simulants comprised of the majority of minerals found in Lunar and Martian surficial regoliths often lack some minor or rare phases (including phosphates, sulfides, and phyllosilicates) that affect ISRU and plant growth. This limitation can be overcome by the exogenous addition of minerals that are deficient in the selected rocks. However, even with this addition, it is difficult to replicate all regolith characteristics in a single simulant (Seiferlin et al., 2008). As a result, many research teams have produced their own simulants over the years (Cannon et al., 2019). In any case, no existing simulants contain moisture or biological components (Gertsch et al., 2008).
The surface morphology of the Moon is dotted with meteorite and micrometeorite impact sites (Gertsch et al., 2008). Samples brought back to Earth by the Apollo missions at the end of the 1970s revealed that the Lunar regolith was a mixture of varying amounts of two primary rocks: 1) the Lunar Mare dark basalt and 2) the lighter-colored, feldspar-rich anorthosite of the Lunar Highlands. These are mixed with an approximately constant proportion of impact melt glass (McKay et al., 1994). Based on the mineralogical composition of this regolith, Earth & Space 2006 and the 2nd NASA/ARO/ASCE Workshop on Granular Materials in Lunar and Martian Exploration (Malla et al., 2006) proposed two compositional end-members of Lunar simulants to be used as an ideal set of root simulants: 1) low-Ti basalt for Lunar Mare and 2) high-Ca anorthosite for Lunar Highlands. Indeed, the Lunar Mare simulants FJS-3, Oshima simulant, FJS-2, OPRL2N, and FJS-1 are 81–100% basaltic (Supplementary Table S1), whereas the anorthositic rocks in the Lunar Highland simulants NU-LHT/1M/2M/3M/1D/2C, OPRH2N/H2W, and OPRH3N/H3W are 43–80% basaltic (Supplementary Table S1). As shown analytically (Supplementary Table S1) and synthetically (Figure 1; Supplementary Table S2), the mineralogical compositions of only 11 Lunar simulants have been characterized.
FIGURE 1. Data of the figure are relative only to simulants (19/30) whose mineralogical composition is reported for more than 73% of the total mass in the literature (Supplementary Table S1). The mineralogical classes are split as follow: Oxides (Ilmenite; Magnetite; Ti-magnetite; Hematite; Ferrihydrite; Cr-spinel); Carbonates (Calcite; Fe-carbonate; Mg-carbonate); Phosphates (Apatite); Sulphates (Gypsum; Selenite; Mg-sulfate; Anhydrite); Sulfides (Pyrrhotite; Sulfide); Nesosilicates—inosilicates (Olivine + pyroxene + ilmenite; Olivine; Clinopyroxene; Augite; Enstatite; Pyroxene + Hornblende; Pyroxene; Diopside; Hypersthene); Tectosilicates—Quartz, Zeolite; Tectosilicates—Plagioclases (Plagioclase; Anorthite; Albite; Labradorite); Tectosilicates—K-feldspars (Sanidine; K-feldspar; Orthoclase); Phyllosilicates (Smectite; Saponite; Chlorite); Other minerals (undifferentiated). Glass + opaque (Plagioclase glass, basaltic glass, hydrated silica, Volcanic glass; Glass; Opaque + Glass).
The mineralogy of the Lunar Mare and Highlands simulants primarily consists of plagioclases, mafic minerals (nesosilicates and inosilicates such as olivine and pyroxene), and glass plus opaque (with a prevalence of glass) (Figure 1; Supplementary Table S2). On average, compared to Lunar Highland simulants, Lunar Mare simulants are more enriched with mafic minerals (7 vs. 35%, respectively) and oxides (0.2 vs. 2.9%, respectively). However, Lunar Highland simulants contain higher levels of anorthite plagioclases than Lunar Mare simulants (59 vs. 34%, respectively).
During the period of the Apollo missions, exploration activities on the Lunar surface were seriously hampered by dust. Consequently, an additional type of simulant was proposed, called Lunar Dust. This simulant was created based on the data on Lunar dust collected by the Lunar Soil Characterization Consortium (Taylor et al., 2001; Wallace et al., 2009). Available data on the petrographic characteristics of this simulant indicate a wide range of rock types, ranging from gabbro to anorthosite (Supplementary Table S1). In accordance with the mineralogy of the Lunar dust regolith, the Lunar Dust simulant is enriched by glass and opaque than the Lunar Mare and Lunar Highland simulants (53 vs. 25% and 33%, respectively), and exhibits lower levels of nesosilicates, inosilicates, and tectosilicates (Figure 1; Supplementary Table S2).
The Martian surface was shaped by the combined action of the wind (physical erosion) and water (chemical weathering) and lava flows (Zeng et al., 2015; Cannon et al., 2019), all of which contributed to the formation of the Martian “soil” (Bandfield et al., 2011). The data collected by the Curiosity rover over the last decade have shed light on the composition and physical properties of the Martian regolith. Peters et al. (2008) reported that the Martian regolith is classified as a fine-grained and cohesionless rocky soil that is mixed with dust due to planet storms. The surface is covered by a basaltic sand that is mainly composed of plagioclases and mafic minerals (including nesosilicates and inosilicates such as olivine and pyroxene) (Peters et al., 2008; Zeng et al., 2015; Filiberto, 2017). The Martian regolith also contains relatively lower levels of phyllosilicates (smectite and saponite), sulphate salts (such as gypsum, anhydrite, and alunite-jarosite) (McSween and Keil, 2000; Gaillard et al., 2013; McCollom et al., 2013), and the iron oxides (such as magnetite, hematite, and ferrihydrite) (Benison et al., 2008; Peters et al., 2008; Zeng et al., 2015; Cannon et al., 2019) that make Mars “the red planet” (Grotzinger et al., 2014; Hurowitz et al., 2017).
The bulk chemistry and physicochemical properties of Lunar and Martian regolith simulants have been analyzed to assess their ability to support extra-terrestrial farming in the future. Studies are primarily focused on the essential nutrients required by plants to complete their life cycle. Plants can obtain large quantities of macronutrients (including N, P, K, S, Ca, and Mg) from the growth media. These, along with C, H, and O (derived from the atmosphere) contribute to over 95% of a plant’s entire biomass (when measured as dry matter). Because micronutrients are required in lower quantities, their levels in plant tissues are measured in parts per million. These include Cl, B, Zn, Fe, Mo, Mn, Cu, and Ni.
In the absence of any organic matter, the main chemical compositions and mineralogy of Lunar and Martian simulants are very similar to those of their respective reference samples from the Lunar and Martian surfaces (Mortley et al., 2000; Beegle et al., 2007; Peters et al., 2008; Zeng et al., 2015; Kölbl et al., 2017). The data from rovers show low variability in bulk chemical composition of regolith in the areas where measurements have been acquired (Zeng et al., 2015). Nevertheless, we need to assume that the unexplored areas of the Lunar and Martian surfaces may exhibit different mineralogy and chemical compositions. NASA’s Perseverance rover has successfully cored Martian rocks, and data received from this rover will certainly broaden our knowledge of Martian geochemistry. Figure 2 shows the mean chemical compositions (as oxide percentages) of the 30 simulants listed in Table 1. The oxides of manganese (MnO), titanium (TiO), chromium (Cr2O3), phosphorus (P2O5), and sulfur (SO3) occur at very low concentrations in each simulant category. Therefore, for the sake of clarity, these are all collated in the “others” category (Figure 2). SiO2 is the principal constituent in all the simulants. SiO2 levels are consistently ∼45% in the Lunar simulants, and ∼41% in the Martian ones. This difference is likely due to the higher occurrence of amorphous materials in Lunar simulants (Figure 1). Likewise, Lunar simulants show higher Al2O3 and CaO levels than Martian simulants, whereas the opposite is true for Fe (FeO and Fe2O3) and MgO (Figure 2). These trends are explained by higher levels of Ca-plagioclases in Lunar simulants and of Fe-(hydr)oxides (magnetite, hematite, ferrihydrite), nesosilicates (olivine), and inosilicates (pyroxene) in Martian ones. Lunar and Martian simulants also contain significant levels of other nutrients essential for plant growth. These include potassium (average, 0.9%; 0.7% of K2O), phosphorus (0.3%; 0.3% of P2O5), sulfur (0.1%; 4.4% of SO3), and manganese (0.1%; 0.1% of MnO), which are derived from K-feldspars, phosphates, sulfates, and Mn oxides, respectively (Figure 1). The simulants also contain inorganic carbon in the form of carbonates, and their levels are higher in Martian simulants than in Lunar ones. Simulants also contain non-negligible amounts of sodium (Lunar: 2.8% of Na2O; Martian: 2.4% from Na-plagioclases) and potentially toxic elements (e.g., Cr as Cr2O3), which may induce salt or other abiotic stresses in rhizosphere-competent microorganisms and plants (Caporale et al., 2020). Simulants lack key nutrients for plants such as N, which is frequently absent in minerals and occurs in biomolecules. Micronutrients such as Mo, Ni, B, Cu, and Zn are generally occluded in accessory minerals in trace concentrations (i.e., in the order of parts per million).
FIGURE 2. Bulk chemistry of 30 lunar and martian simulants listed in Table 1 (the source of the information used to build the graph and the analytical techniques used to produce chemical data are reported in Table 1 and in the text).
The total amount of the most essential elements may be more than adequate to satisfy the requirements for plant growth in simulants. However, plants generally take up only the bioavailable forms of elements (such as the readily soluble and exchangeable forms), and not the elements occluded in mineral structures that are released only after mineral weathering. For plants, nutrient availability in soil is governed by the pseudo-equilibrium between aqueous and solid phases, rather than by the total nutrient content. Factors such as pH, redox potential, electrical conductivity (EC), texture, type and relative abundance of fine solid particles play a key role in regulating nutrient availability in a plant growth medium (Adamo et al., 2018). Thus, these factors should be assessed when growing plants in regolith simulant-based substrates. Unfortunately, the pH and EC of simulants are not provided in the Planetary Simulant Database. Studies report that the Martian and Lunar simulants have a pH above 6, and have alkaline properties in some cases (Gilrain et al., 1999; Zaets et al., 2011; Wamelink et al., 2014; Caporale et al., 2020; Eichler et al., 2021), suggesting low rates of mineral weathering and cation release. The only data regarding EC in Martian simulants indicate low values of 0.2–0.3 dS m−1 (Gilrain et al., 1999; Caporale et al., 2020), suggesting no adverse effect of salinity on plant growth.
Agronomic techniques and crop management also affects nutrient availability and dynamics in a growth substrate. Thus, the addition of sustainable amounts of organic amendments (e.g., compost or manure) or mineral fertilizers to Lunar and Martian simulants may enhance the bioavailability of essential nutrients and provide missing vital nutrients such as N and organic C. These practices can also aid in pH adjustment and have positive effects on microbial rhizosphere activity and nutrient biogeochemical cycles.
Potentially toxic elements such as Al and Cr usually precipitate and are poorly available in alkaline environments (Brautigan et al., 2012); therefore, their presence in non-negligible amounts should not hinder plant growth in simulants. However, analyzing the bioavailability of these elements in the substrates and their levels in plant tissues may help evaluate the potential risk they pose to space crews. Due to the phytochemistry of the cold and oxidizing environment, toxic perchlorate salts occur commonly on the Martian surface in concentrations of 0.5–1% (Oze et al., 2021); however, they were not present in any of the 30 simulants. Perchlorates can be taken up by plants and make their edible parts unsafe to eat. To remediate Martian soils rendered toxic by perchlorates, several papers have proposed a biochemical approach that involves transforming perchlorates into chloride and oxygen (Rikken et al., 1996; Davila et al., 2013).
Reduced gravity (e.g., in a spacecraft in orbit) causes changes in crucial hydrological variables and alters some fundamental characteristics of water flow and liquid distribution inside a porous medium. The characteristic retention curve of a porous medium is of paramount importance for water movement and plant growth. In the terrestrial environment, water movement towards root hairs is determined by both gravity, which decreases with water depletion, and capillary forces, which increase as water content decreases. In microgravity, capillary forces exert complete control over liquid distribution in a plant growth medium. By simulating a wetting and drying cycle using conventional unsaturated flow models adapted to microgravity, Jones and Or (1999) showed that the retention curve has narrower pore-size distributions in microgravity. This may be due to particle rearrangement, increased air entrapment, and enhanced hysteresis. The authors also reported a decrease in unsaturated hydraulic conductivities, which can be explained by the modified hydrodynamics in microgravity. Chamindu Deepagoda et al. (2014) used equations to quantify the water retention of substrates in terrestrial conditions and under different gravity conditions. Their analysis concluded that the Lunar simulant was the worst in terms of water retention capacity, likely because of its large pore space (0.52 cm3 cm−3) and particle size (0.25–1.0 mm).
Maggi and Pallud (2010) evaluated the effect of Martian gravity on soil processes by using a highly mechanistic model. This model had been previously tested for terrestrial crops, and couples soil hydraulics and nutrient biogeochemistry. The net leaching of NO3− solutes, gaseous fluxes of NH3, CO2, N2O, NO, and N2, the depth concentrations of O2, CO2, and dissolved organic carbon (DOC), and pH in the root zone were measured in two cropping units under a simulation of the gravitational conditions on Earth and Mars (9.81 and 0.38 g, respectively). These units were similarly fertilized and irrigated, but had different initial soil moisture content. The water and nutrient leaching of soil was reduced by 90% under Martian gravity. This enhanced the microbial metabolism, promoted faster decomposition of DOC, and much higher emission of NO, N2O, N2, and CO2. The authors concluded that cultivation on Mars would require less water for irrigation and lower external nutrient supply than on Earth. Unless the soil bulk density is very high, it is expected to have small influences on plant growth. Based on data from the Pathfinder lander, the potential bulk density of Martian soil has been estimated at 1.07–1.64 g cm−3 (Moore et al., 1999). The data from the Viking 1 lander suggests a bulk density of 1.15 g cm−3 for Martian soil (Moore and Jakosky, 1989), with a volatile loss of 0.1–1.0% by weight at 500°C (Peters et al., 2008). The bulk density of Lunar soil varies between 1.5 and 1.7 g cm−3 (Taylor, 2007).
Pore size distribution is potentially associated with the percolation process, such that an increase in particle size enhances the percolation process. The percolation threshold changes under reduced gravity, resulting in improved aeration conditions in the early stages of simulations. Although reduced gravity can influence gas and fluid movement, it does not necessarily lead to better rhizospheric conditions. This is because the root zone is primarily influenced by air in pore spaces (Chamindu Deepagoda et al., 2014). Therefore, if the small pore space is filled with air and the substrate is almost water-saturated, conditions could become critical for plant growth and would need to be managed carefully.
Particle size distribution is another critical aspect that influences the potential of a regolith to be a growth medium. On Earth, soils are made of particles (namely clay, silt, and sand particles) of different sizes. The optimal assemblage (loam texture) of certain proportions of sand, silt, and clay-sized particles can maximize the ability of the soil to sustain plant growth. The particle size distributions of Lunar and Martian simulants must be modified to create an optimal particle assemblage (Cannon et al., 2019) that promotes air permeability (to avoid anoxia stress) and geochemical and mechanical interactions to enhance element bioavailability (Beegle et al., 2007; Zeng et al., 2015; Cannon et al., 2019).
Caporale et al. (2020) conducted an interesting study on the mixing of organic compost with the MMS-1 Martian simulant. This study showed how the addition of green compost to the MMS-1 simulant affects the physical and hydrological properties of the mixture. As expected, the bulk density progressively decreased with increasing rates of compost in the mixture, ranging from 1.39 g cm−3 (pure simulant) to 0.60 g cm−3 (pure compost). The addition of compost to pure simulant proportionally increased the maximum amount of water retained. The retention curves of the pure simulant and a 70:30 (v/v) simulant:compost mixture tended to converge when the matrix suction was approximately 60 cm. In contrast, the retention curve of a 30:70 simulant:compost mixture was always higher than those of the other two substrates. However, the suitability of a substrate for the cultivation of a candidate crop cannot be established solely through analysis of the retention curve. In other words, higher values of the saturated water content do not necessarily translate to better performance. The authors also showed that compost addition to the pure simulant exerted a more significant effect on the macropore region than on the micropore domain. Water held in macropores exceeding 120 μm in diameter was not directly beneficial for root water uptake in lettuce (the plant considered in the study), and even caused root asphyxia. Moreover, the three mixtures considered had a similar distribution of pore sizes with diameters below 50 μm. Based on its hydraulic properties, the 70:30 mixture was best substrate, as the percentage increase in large pores (diameter, 50–120 μm) was more significant in this mixture than in the 30:70 one. In terms of water and nutrient transport processes, all the mixtures were acceptable as growth media in a hydroponic cultivation system, where a timely water supply is guaranteed. However, if the objective is to manage lettuce irrigation to minimize irrigation frequency, the 70:30 mixture was the most promising substrate due to its hydraulic properties. Thus, the concerns change if the aim is to have an optimized collection system with more efficient energy consumption and system usage.
A good knowledge of the physicochemical and hydraulic properties of Lunar and Martian regolith simulants is of paramount importance in developing and building off-world BLSS based on an ISRU approach, in which native substrates are exploited as plant growth media. Evaluating how simulant properties can influence plant growth, physiology, and health can help overcome deficiencies and critical concerns in a sustainable and effective way. The macro- and micronutrient levels, porosity, and water availability are fundamental parameters in assessing the capability of a substrate to sustain plant growth. Many studies have evaluated substrate efficiency (Ming et al., 1993; Ming and Henninger, 1994; Aglan et al., 1998; Mortley et al., 2000) and water management (Ramírez et al., 2019; Wamelink et al., 2019) over the years. To be integrated with crop production, a good regolith simulant should have certain physical characteristics, including the following: 1) optimal water holding capacity to maintain an effective level of humidity after irrigation; and 2) optimal air circulation in the porous medium to allow efficient gas exchange and root and microbial respiration. Plants commonly take up nutrients from the soil solution, either in a dissolved form, through exchange, or through easy release at the solid-water interface. The fluxes of water, air, and nutrients in a growth substrate are closely linked to its physicochemical properties (McFee and VanScoyoc, 1975). Therefore, particle interactions or aggregation and the consequent formation of a structured substrate are critical for better plant growth. For extra-terrestrial farming, it is well-established that several simulant properties—such as an alkaline pH, a high availability of sodium, the predominance of macro-vs. micropores, and a scant water holding capacity—can influence plant growth, health, and vigor (Wamelink et al., 2014; Caporale et al., 2020). Thus, it is essential to evaluate whether these soil simulants can be exploited to support future colonies.
As reported in Section 2.2, plants need macro and micronutrients for optimal growth, and these can be found in both inorganic and organic forms in soil on Earth (Hopkins and Huner, 2008; Fageria, 2009). Terrestrial soil minerals (mainly feldspars and micas) are the main source of K. Calcium, Mg, S, and Fe, are usually abundant in soil, and microelements such as Zn, Cu, Mn, B, Mo, Cl, and Ni originate from both minerals and organic matter. Carbon is absorbed from the atmosphere through photosynthesis (Hopkins and Huner, 2008), and organic matter is the primary source of both N and P. Lunar and Martian soils lack organic matter and biotic activity (Seiferlin et al., 2008), although there is some evidence of a biofilm (Thomas-Keprta et al., 2014; Eigenbrode et al., 2018). It is also worth noting that the Curiosity rover investigations at Gale crater on Mars discovered indigenous N in sedimentary and aeolian deposits (Stern et al., 2015), although its exact concentration and potential use for plant growth is debatable. Thus, Lunar and Martian soils (and, therefore, regolith simulant-based substrates) are potentially deficient in all macro- and micronutrients, which are derived exclusively (N), mostly (P, S), or partly (K, Ca, Mg, Fe, Zn, Cu, Mn, B, Cl, and Ni) from the degradation of organic components. Thus, regolith simulants cannot support sustainable ISRU for crop production without exogenous inputs of inorganic fertilizers or organic matter.
To date, Lunar and Martian simulants have been primarily studied for applications other than agronomy (de Vera et al., 2004; Gertsch et al., 2008; Kölbl et al., 2017; Karl et al., 2018), although they have potential use as a substrate for crop growth (Mortley et al., 2000). Several strategies and treatments can be applied to ameliorate the nutrient deficiency of simulants and enhance their performance as plant growth substrates. The first solution involves the use of a stable organic amendment, not only as a supply of organic carbon, but also to improve the physical features of regolith (Gilrain et al., 1999). The use of this amendment and soil tillage can also help mitigate the effects of microgravity on water leaching (Maggi and Pallud, 2010). Furthermore, selected pioneer plants can be grown initially (Kozyrovska et al., 2006) to improve the root zone during cultivation and provide plant residues for humification at the end of their life cycle.
The selection of candidate pioneer plant species is a key aspect to consider (Gilrain et al., 1999; Mortley et al., 2000; Kozyrovska et al., 2006; Wamelink et al., 2014; Ramírez et al., 2019). Plants produce O2 and fix CO2, serve as food for space crews, have a role in water recycling (Maggi and Pallud, 2010; Llorente et al., 2018), and are actively involved in soil structure formation. To improve ISRU, several researchers have proposed the development of microbial consortia (Zaets et al., 2011; Verseux et al., 2016; Llorente et al., 2018) that could improve the mineral uptake of plants.
The first studies of plant growth on Lunar regolith were conducted in the early 70s in the Lunar Receiving Laboratory, where small amounts of real Lunar materials (brought from the Moon by the Apollo missions) were mixed with growth media (such as wood pulp product) stabilized with acrylonitrile resin (Walkinshaw et al., 1970; Walkinshaw and Johnson, 1971; Weete et al., 1972; Weete and Walkinshaw, 1972). These studies were designed to determine whether Lunar materials contained any agents capable of generating an epiphytotic disease in representative species of the plant kingdom. The Lunar material was sterilized to avoid external contamination, and the entire laboratory was kept under quarantine conditions. The plant growth substrates containing extra-terrestrial materials were treated as inert media without considering their nutrient content and composition (Table 2). The main parameters monitored were seed germination capacity, growth alteration, phytotoxicity, and disease incidence. Walkinshaw et al. (1970) grew 35 representative plant species in aseptic conditions in different cultivation systems, including algae, seeds, spores, seedlings, gametophytes, and tissue cultures of higher plants. The authors noted the absence of disease agents in the plants tested under experimental conditions, and concluded that the Lunar material could potentially support the growth of a wide range of plant species. Specifically, ferns, liverworts, and tobacco were particularly effective in exploiting the Lunar material as a nutrient source. A year later, Walkinshaw and Johnson (1971) focused on the possible differences in chemical composition among plants grown on Lunar material. The results showed a direct interaction between plant species and Fe, Al, and Ti uptake from the Lunar substrate. Notably, cabbage and Brussels sprouts exhibited higher absorption of Mn.
Based on the findings of Walkinshaw et al. (1970), researchers further investigated the effect of the Lunar material on tobacco plants in terms of their constituent biomolecules and secondary metabolites. A tissue culture experiment over a period of 12 weeks used the Lunar material recovered from Walkinshaw’s experiment, which was washed and sterilized before the trial. Weete et al. (1972) found that tissue grown in contact with Lunar material had a higher concentration of total sterols than in the control. They also found differences in absolute and relative fatty acid concentrations. Moreover, the chlorophyll and carotenoid concentrations were higher in treated plants, with chlorophyll a being the major pigment present (Weete and Walkinshaw, 1972). According to the review by Ferl and Paul (2010), the Apollo-era plant experiments with Lunar samples provided many insights into the biological impact of the Lunar environment on terrestrial life forms, which were useful for future research in support of Lunar exploration. The modern molecular approaches (-omic sciences) were not available during the Apollo-era plant experiments. However, those studies provided useful preliminary information on how Lunar samples and Earth biota interacted with and affected each other.
As a part of NASA’s Advanced Life Support Program, Aglan et al. (1998) and Mortley et al. (2000), evaluated the response of sweet potato clones grown under microgravity in Lunar and Martian simulant media containing a buried microporous tube system for watering and fertigation. In these tests, the simulants mainly provided mechanical anchorage for the plant roots, and did not cause any adverse or toxic effects in the plants. Therefore, the authors concluded that both simulants showed potential for use as a substrate for crop production.
As a biotechnological approach to plant cultivation in an extreme environment (such as a Lunar base), Kozyrovska et al. (2006) proposed the growing of pioneer plants (Tagetes patula L.) in a Lunar rock anorthosite substrate. The simulants contained specific root-colonizing bacteria that could decompose the Lunar silicate rock and release the cations essential for plant growth. This strategy may prove to be a practical necessity in order to support plant growth in a substrate with low nutrient availability. The primary function of the pioneer plants with associated microorganisms is to form a soil with adequate fertility. This soil can then be used to grow plants of a second generation (such as wheat, rice, and soybean, among others) to provide Lunar explorers with fresh sources of vitamins, nutrients, and biomolecules. At the end of the growth cycle, the authors demonstrated that the first-generation plant residues could serve as a supply of green manure for humification and as a potential nutrient source.
The first large-scale controlled experiment evaluating potential plant growth (germination, growth, flowering, and seed formation) on the JSC1-1A Lunar and JSC-1A Martian regolith simulants was conducted by Wamelink et al. (2014). Fourteen different species of wild plants, crops, and nitrogen fixers (see Table 2) were grown for 50 days in isolation under Earth-like light and atmospheric conditions, while only using demineralized water and no fertilizers or substrate amendments. The results indicated that neither simulant was an adequate source of plant nutrients. Nevertheless, the Martian simulant outperformed the Lunar simulant in biomass production, as it had trace levels of ammonium nitrate and carbon and no stressors that could cause a higher pH or low water holding capacity. Tomato and wheat crops performed particularly well on the artificial substrates. Three species flowered, but only two produced seeds. In conclusion, the authors raised several open questions regarding the representativeness of the simulants, their water holding capacity, the availability of N and other nutrients on Mars and the Moon, and the influences of gravity, light, and other extra-terrestrial environmental conditions.
Waste management and efficient resource use are critical aspects of BLSS for both the Moon and Mars. A possible solution for the problem of waste management is composting, which can be incorporated into the agronomic treatment of regoliths as an amendment in line with the ISRU approach. By mixing compost with various regolith simulants, several studies have evaluated the role and potential utility of organic waste in plant cultivation and the management of extra-terrestrial settlements. These investigations not only provide a better approach to the management of residues, but elucidate the effects of organic matter amendment on mineral-based substrates (Gilrain et al., 1999; Wamelink et al., 2019; Duri et al., 2020). To help overcome the chemical constraints on plant growth in pure simulants, Gilrain et al. (1999) conducted preliminary studies in an ALS plant growing system using a variety of proportional combinations of the JSC Mars-1 regolith simulant and a municipal leaf compost. The Swiss chard was used as a candidate crop, and half of the treatments received a modified half-strength Hoagland’s solution. Plants grown in compost:simulant ratios of 1:0, 3:1, and 1:1 showed yields that were greater than those in the 1:3 and 0:1 ratios, and control plants irrigated with only water produced similar trends. However, overall plant growth was significantly lower, indicating that nutrient supply by both the compost and regolith simulant was not enough to sustain the entire plant growth cycle. The authors concluded that the compost mainly promoted plant growth by improving the physical features of the regolith that regulate water and/or nutrient availability.
A study reported the growth of 14 different plant species on a Martian soil simulant and, to a lesser extent, on a Lunar soil simulant (Wamelink et al., 2014). As a follow-up experiment, Wamelink et al. (2019) grew 10 different crop species (see Table 2) on the JSC1-1A Lunar and JSC-1A Martian regolith simulants (provided by NASA) containing organic residues from first harvests (fresh mown grass of Lolium perennne L.). A nutrient solution was also added to mimic the addition of human feces and urine. The main goal was the production of edible crops and their seeds for a next generation. The authors harvested the edible parts of nine out of ten crops. The biomass production was highest in the Earth control and the Martian soil simulant, but was significantly lower in the Lunar simulant. Only three species (radish, rye, and cress) produced seeds. Radish germination rates were lower in the Lunar simulant than in the Earth control soil and Marian simulant. The authors defined their study as a small step towards the implementation of a sustainable agricultural ecosystem for a Lunar or Martian colony. They further encouraged the search for the optimal organic matter content and physical characteristics of the simulants in future studies.
Recently, two butterhead lettuce (Lactuca sativa L. var. capitata) cultivars (green and red Salanova®) have been cultivated in the MMS-1 Mojave Mars simulant mixed with green compost at different rates (simulant:compost ratios, 0:100, 30:70, 70:30, and 100:0; v:v) in a phytotron open gas exchange growth chamber (Caporale et al., 2020; Duri et al., 2020). A detailed characterization of the physicochemical, mineralogical, and hydrological properties of the simulant, compost, and their mixtures was provided. This was the first characterization of MMS-1 in terms of its mineralogical composition (x-ray diffraction) and spectroscopic features (by mid-infrared MIR spectroscopy). MMS-1 was found to be a coarse-textured alkaline substrate mostly composed of plagioclase, amorphous material, and (to a lesser degree) of zeolite, hematite, and smectites. Although it was a source of nutrients for lettuce, it did not supply organic matter, N, and S, and provided very scant amounts of P. As reported above in Section 2.3, organic amendment improved the physical properties of the simulant (such as bulk density and water holding capacity). It also lowered the pH of the alkaline simulant, enhanced its cation exchange capacity, organic C and N levels, and the availability of macro- and micronutrients. The red Salanova® lettuce grown in the 30:70 mixture showed the best crop performance, photosynthetic activity, intrinsic water use efficiency, and quality traits (mineral, carotenoid, and phenolic contents). The 70:30 mixture showed a slight decline in lettuce yield and quality; however, the authors concluded that it was a more sustainable choice for space farming, as it exhibited more efficient use of limited resources (e.g., compost). The study by Caporale et al. (2020) found discrepancies between the measured bulk chemistry of the MMS-1 simulant and that provided by the producer. This supported the observations by Cannon et al. (2019), who suggested that the MMS-1 simulant was derived from different source material than the original MMS. In the absence of rigorous documentation by producers of simulants, this shows the need for an adequate characterization of commercial simulants prior to the designing and planning of any scientific experiments.
Over the past 2 years, the new data collected by rovers has allowed the scientific community to broaden its knowledge of Martian environmental features. For instance, the Martian surface has been found to have a high salt concentration (Ramírez et al., 2019) and high levels of perchlorates (Eichler et al., 2021). To evaluate the impact of abiotic stressors on plant growth and health, Ramírez et al. (2019) tested the responses of 65 potato genotypes grown in Mars-like soil from the La Joya desert in Southern Peru (characterized by high EC, ranging from 19.3 to 52.6 dS m−1). Only 40% of the genotypes survived and yielded crops (0.3–5.2 g tuber plant−1). At the end of the study, the authors stated that the selection of tolerant genotypes, appropriate sowing methods, and soil management strategies were crucial for crops to withstand the extreme salinity and yield produce. More recently, Eichler et al. (2021) tested the growth rates of lettuce and Arabidopsis plants cultivated on three pure Martian regolith simulants—JSC-Mars-1A, Mars Mojave simulant (MMS), and Mars Global simulant (MGS-1)—enriched with calcium perchlorate (2% w/v). None of these simulants could support plant growth in the absence of nutrient supplementation. However, with the addition of nutrients, both plant species grew on JSC-Mars-1A and MMS, but did not grow on MGS-1. The authors linked this failure to the high alkalinity of MGS-1, and suggested acidifying the simulant to achieve plant growth. Calcium perchlorate-enriched simulants were unable to sustain plant growth, even with nutrient supply.
Fackrell et al. (2021) have developed and characterized five new Martian simulants—Global soil (MBas), Phyllosilicate-smectite (MPSmec), Phyllosilicate-illite/chlorite (MPChl), Sulfate-rich (MSul) and Carbonate-rich (MCarb)—for applications in space farming tests. These simulants have been found to be mineralogically, chemically, and spectrally comparable to Martian regolith and bedrock (according to available data), and are exploitable for plant growth in future studies on Martian surface analogues. In the conclusion to their work, the authors strongly advised that the fertility and feasibility of a simulant should be assessed not only on the basis of its mineralogical/chemical composition, but also on how physical and (bio)chemical weathering of the substrate affects its nutrient bioavailability over time.
This paper was intended to be a comprehensive review of the potential for Lunar and Martian simulants being used as substrates for plant growth. Given the costs associated with shipping to either of these off-world sites, as well as the need to establish sustainable off-world operations, more research in this area is essential. This review analyzed more than 70 articles on the Lunar and Martian regolith simulants used as analogues in terrestrial experiments. We identified their main properties, critical aspects, currently available solutions to enable the growth of higher plants, and the potential challenges in deploying them in life support systems.
The literature review showed that pure regolith simulants may be suitable media for plant growth (at least for limited periods) and function as a source of essential nutrients such as K, Ca, Mg, and Fe. However, they lack organic matter and key macronutrients such as N, P, and S. Furthermore, these simulants generally exhibit numerous features harmful for plant health, such as an alkaline pH, high availability of Na, low cohesion of mineral components, the predominance of macro-vs. micropores, and low water holding capacity. In addition, the Martian regolith sometimes contains toxic perchlorates. Hence, the configuration of a mineral-rich and fertile biological substrate for edible plant growth based on regolith simulants still presents a challenge in space biology research.
In many studies, nutrient deficiency in simulants was overcome by fertigation with nutrient-rich solutions (e.g., Hoagland). However, this agronomic technique is not feasible and sustainable in space agriculture, as the nutritional resources must be carried from the Earth and cannot be produced in BLSS. A promising strategy is the adding of in situ recycled organic matter to enrich regolith simulants. This is a sustainable and effective technique to enhance the chemical and biological fertility and physical and hydraulic properties of regolith-based substrates (e.g., permeability and water retention). The organic waste produced by BLSS crews can help recover compounds and allow their use as fertilizers or compost to support plant growth. Thus, future studies should evaluate the efficacy of these treatments. Moreover, consecutive cycles of plant cultivation on the same regolith-based substrate can allow prolonged root exudation and the release of organic acid molecules and CO2. This can lower the substrate pH, increase mineral weathering rates, enhance nutrient release/availability, promote the aggregation of particles of different sizes (to form a more efficient porous system), and overall contribute to soil improvement.
Many vital aspects of space farming have already been explored in the published experiments on plant growth in simulant-based substrates. Nevertheless, many other challenging factors still need to be considered to assess the true potential of extra-terrestrial farming based on the exploitation and development of in situ Lunar and Martian resources. For example, water management and recycling are paramount in sustainable BLSS modules developed and based on the ISRU strategy. Water is another limited resource in space, and we need a better understanding of water movement and fluxes in regolith-based substrate/plant systems under microgravity. In extra-terrestrial soil, water dynamics would regulate the extent of mineral weathering and the rate of organic matter decomposition, thus greatly affecting the biogeochemistry and bioavailability of nutrients and plant growth. Future studies simulating the potential environmental conditions of off-world bases are highly recommended.
Another aspect which needs further investigation is the effect of the space environment (such as different gravity and climatic conditions) on plant physiology (e.g., the biophysical limitations on gas exchange and transpiration), and how this affects plant growth and productivity and substrata properties. In sustainable scenarios of space farming, regolith-based substrates are required to sustain plant growth throughout the plant life cycle, including the complete seed maturation needed for reproduction. Although this may be feasible for microgreens or salad crops, it is more challenging for other candidate species such as potato (a source of carbohydrates) and soybean (a source of proteins), which require more nutrients and resources to produce a sufficiently edible yield than do salad crops.
The presence of—and interactions with—biota (including pathogens, cyanobacteria, plant growth-promoting bacteria, beneficial symbiotic fungi, and worms) and biostimulants will add further complexity to life support systems with extra-terrestrial soils. However, little is known about their effects on: 1) mineral weathering rates in the early stages of terraforming; 2) decomposition and recycling of organic plant residues and human excreta; 3) plant nutrition mediated by symbiotic fungi, compared with that mediated by entirely abiotic systems; and 4) protection of plants from environmental stresses. The occurrence of perchlorates in the Mars regolith provides a significant challenge to its use as an agricultural substrate. Thus, further steps—such as water rinses, phytoremediation, volatilization, and chemical reduction by using perchlorate-reducing bacteria—are necessary to make Mars regolith a viable growing substrate.
In conclusion, we encourage research that examines the effects of using in situ extra-terrestrial soils on several fundamental environmental functions (other than food security and biomass production) that constitute the primary functions of soil. On Earth, soils are the link between the air, water, rocks, and organisms. Soils are known to affect the climate, atmosphere composition, carbon and nutrient recycling, water quality and maintenance, biotic regulation, buffering, and the transformation of potentially harmful elements and compounds. In future, we should dedicate more attention to the development of pedogenesis on extra-terrestrial surface materials and explore the effects of regolith/simulant weathering on the soil-plant-atmosphere system. Providing ecosystem services by using a regolith-based substrate might be the key to a fruitful and sustainable Lunar or Martian BLSS.
LD, SV, MP, and AGC, contributed to the original draft of the manuscript. YR, SDP, and PA guided the editing process and the literature search.
This research was supported by the project In-situ REsource Bio-Utilization for life Support system (ReBUS), unique project code (CUP) F74I16000000005 financed by the Italian Space Agency.
The authors declare that the research was conducted in the absence of any commercial or financial relationships that could be construed as a potential conflict of interest.
All claims expressed in this article are solely those of the authors and do not necessarily represent those of their affiliated organizations, or those of the publisher, the editors and the reviewers. Any product that may be evaluated in this article, or claim that may be made by its manufacturer, is not guaranteed or endorsed by the publisher.
We would like to thank Editage (www.editage.com) for English language editing.
The Supplementary Material for this article can be found online at: https://www.frontiersin.org/articles/10.3389/fspas.2021.747821/full#supplementary-material
Adamo, P., Agrelli, D., and Zampella, M. (2018). “Chemical Speciation to Assess Bioavailability, Bioaccessibility and Geochemical Forms of Potentially Toxic Metals (PTMs) in Polluted Soils,” in Environmental Geochemistry: Site Characterization, Data Analysis and Case Histories (Amsterdam, AL: Elsevier), 153–194. doi:10.1016/B978-0-444-63763-5.00010-0
Aglan, H., Mortley, D., Trotman, A., Loretan, P., and Hill, W. (1998). Sweetpotato Growth Using a Microporous Tube System with Lunar Simulant Medium. SAE Trans. (Jstor) 107, 1012–1016. doi:10.4271/981806
Archer, P. D., Hogancamp, J. V., Gruener, J. E., and Ming, D. W. (2018). “Augmenting the Mojave Mars Simulant to More Closely Match the Volatile Content of Global Martian Soils Based on Mars Science Laboratory Results,” in 49th Lunar and Planetary Science Conference, The Woodlands, Texas, USA, March 19-23, 2018 (Woodlands, Texas: LPI Contribution), 2806.
Bandfield, J. L., Deanne Rogers, A., and Edwards, C. S. (2011). The Role of Aqueous Alteration in the Formation of Martian Soils. Icarus 211, 157–171. doi:10.1016/j.icarus.2010.08.028
Bates, S., Gushin, V., Bingham, G., Vinokhodova, A., Marquit, J., and Sychev, V. (2009). Plants as Countermeasures: A Review of the Literature and Application to Habitation Systems for Humans Living in Isolated or Extreme Environments. Habitation (elmsford) 12, 33–40. doi:10.3727/154296610X12686999887201
Battler, M. M., and Spray, J. G. (2009). The Shawmere Anorthosite and OB-1 as Lunar highland Regolith Simulants. Planet. Space Sci. 57, 2128–2131. doi:10.1016/j.pss.2009.09.003
Beegle, L. W., Peters, G. H., Mungas, G. S., Bearman, G. H., Smith, J. A., and Anderson, R. C. (2007). “Mojave Martian Simulant: a New Martian Soil Simulant,” in 38th Lunar and Planetary Science Conference 2005, League City, Texas, USA, March 14-18, 2005 (League City, Texas: LPI Contribution).
Benison, K. C., LaClair, D., and Walker, J. (2008). Physical Sedimentology Experiments with Sulfuric Acid Solutions: Implications for Mars? Earth Planet. Sci. Lett. 270, 330–337. doi:10.1016/j.epsl.2008.03.036
Brautigan, D. J., Rengasamy, P., and Chittleborough, D. J. (2012). Aluminium Speciation and Phytotoxicity in Alkaline Soils. Plant Soil 360, 187–196. doi:10.1007/s11104-012-1232-5
Britt, D. T., and Cannon, K. M. (2019). “Highlights of Science and Exploration Activities at the SSERVI CLASS Node,” in European Planetary Science Congress (EPSC-DPS), Copernicus GmbH (Copernicus Meetings), Geneva, CH, September 15-20, 2019 (Geneva, Switzerland: ESF – European Science Foundation).
Cannon, K. M., and Britt, D. T. (2019). “Mineralogically Accurate Simulants for Lunar ISRU, and Strategic Regolith Processing,” in Lunar ISRU 2019: Developing a New Space Economy through Lunar Resources and Their Utilization (Houston: Lunar and Planetary InstituteLPI Contribution). Abstract 5002.
Cannon, K. M., Britt, D. T., Smith, T. M., Fritsche, R. F., and Batcheldor, D. (2019). Mars Global Simulant MGS-1: A Rocknest-Based Open Standard for Basaltic Martian Regolith Simulants. Icarus 317, 470–478. doi:10.1016/j.icarus.2018.08.019
Caporale, A. G., Vingiani, S., Palladino, M., El-Nakhel, C., Duri, L. G., Pannico, A., et al. (2020). Geo-mineralogical Characterisation of Mars Simulant MMS-1 and Appraisal of Substrate Physico-Chemical Properties and Crop Performance Obtained with Variable green Compost Amendment Rates. Sci. Total Environ. 720, 137543. doi:10.1016/j.scitotenv.2020.137543
Certini, G., Karunatillake, S., Zhao, Y.-Y. S., Meslin, P.-Y., Cousin, A., Hood, D. R., et al. (2020). Disambiguating the Soils of Mars. Planet. Space Sci. 186, 104922. doi:10.1016/j.pss.2020.104922
Certini, G., Scalenghe, R., and Amundson, R. (2009). A View of Extraterrestrial Soils. Eur. J. Soil Sci. 60, 1078–1092. doi:10.1111/j.1365-2389.2009.01173.x
Chamindu Deepagoda, T. K. K., Jones, S. B., Tuller, M., de Jonge, L. W., Kawamoto, K., Komatsu, T., et al. (2014). Modeling Gravity Effects on Water Retention and Gas Transport Characteristics in Plant Growth Substrates. Adv. Space Res. 54, 797–808. doi:10.1016/j.asr.2014.04.018
Chow, B. J., Chen, T., Zhong, Y., and Qiao, Y. (2017). Direct Formation of Structural Components Using a Martian Soil Simulant. Sci. Rep. 7, 1151. doi:10.1038/s41598-017-01157-w
Clark, J. V., Archer, P. D., Gruener, J. E., Ming, D. W., Tu, V. M., Niles, P. B., et al. (2020). JSC-rocknest: A Large-Scale Mojave Mars Simulant (MMS) Based Soil Simulant for In-Situ Resource Utilization Water-Extraction Studies. Icarus 351, 113936. doi:10.1016/j.icarus.2020.113936
Davila, A. F., Willson, D., Coates, J. D., and McKay, C. P. (2013). Perchlorate on Mars: a Chemical hazard and a Resource for Humans. Int. J. Astrobiology 12, 321–325. doi:10.1017/S1473550413000189
de Vera, J.-P., Horneck, G., Rettberg, P., and Ott, S. (2004). The Potential of the Lichen Symbiosis to Cope with the Extreme Conditions of Outer Space II: Germination Capacity of Lichen Ascospores in Response to Simulated Space Conditions. Adv. Space Res. 33, 1236–1243. doi:10.1016/j.asr.2003.10.035
Duri, L. G., El-Nakhel, C., Caporale, A. G., Ciriello, M., Graziani, G., Pannico, A., et al. (2020). Mars Regolith Simulant Ameliorated by Compost as In Situ Cultivation Substrate Improves Lettuce Growth and Nutritional Aspects. Plants 9, 628. doi:10.3390/plants9050628
Eichler, A., Hadland, N., Pickett, D., Masaitis, D., Handy, D., Perez, A., et al. (2021). Challenging the Agricultural Viability of Martian Regolith Simulants. Icarus 354, 114022. doi:10.1016/j.icarus.2020.114022
Eigenbrode, J. L., Summons, R. E., Steele, A., Freissinet, C., Millan, M., Navarro-González, R., et al. (2018). Organic Matter Preserved in 3-Billion-Year-Old Mudstones at Gale Crater, Mars. Science 360, 1096–1101. doi:10.1126/science.aas9185
Fackrell, L. E., Schroeder, P. A., Thompson, A., Stockstill-Cahill, K., and Hibbitts, C. A. (2021). Development of Martian Regolith and Bedrock Simulants: Potential and Limitations of Martian Regolith as an In-Situ Resource. Icarus 354, 114055. doi:10.1016/j.icarus.2020.114055
Fageria, N. K. (2009). The Use of Nutrients in Crop Plants. Boca Raton, FL, USA: CRC Press-Taylor & Francis Group. doi:10.1201/9781420075113
Ferl, R. J., and Paul, A.-L. (2010). Lunar Plant Biology-A Review of the Apollo Era. Astrobiology 10, 261–274. doi:10.1089/ast.2009.0417
Filiberto, J. (2017). Geochemistry of Martian Basalts with Constraints on Magma Genesis. Chem. Geology. 466, 1–14. doi:10.1016/j.chemgeo.2017.06.009
Foing, B. H., Racca, G., Marini, A., Koschny, D., Frew, D., Grieger, B., et al. (2018). SMART-1 Technology, Scientific Results and Heritage for Future Space Missions. Planet. Space Sci. 151, 141–148. doi:10.1016/j.pss.2017.09.002
Gaillard, F., Michalski, J., Berger, G., McLennan, S. M., and Scaillet, B. (2013). Geochemical Reservoirs and Timing of Sulfur Cycling on Mars. Space Sci. Rev. 174, 251–300. doi:10.1007/s11214-012-9947-4
Gertsch, L. S., Rostami, J., and Gustafson, R. (2008). “Review of Lunar Regolith Properties for Design of Low Power Lunar Excavators,” in 6th International Conference on Case Histories in Geotechnical Engineering, Washington, DC, USA, August 4-9, 2008 (Missouri University of Science and Technology). Paper No.10.02.
Gilrain, M. R., Hogan, J. A., Cowan, R. M., Finstein, M. S., and Logendra, L. S. (1999). Preliminary Study of Greenhouse Grown Swiss Chard in Mixtures of Compost and Mars Regolith Simulant. SAE. Technical Paper, (No. 1999-01-2021). doi:10.4271/1999-01-2021
Grotzinger, J. P., Sumner, D. Y., Kah, L. C., Stack, K., Gupta, S., Edgar, L., et al. (2014). A Habitable Fluvio-Lacustrine Environment at Yellowknife Bay, Gale Crater, Mars. Science 343, 1242777. doi:10.1126/science.1242777
He, X. X., Xiao, L., Huang, J., Wan, C. H., Wu, T., Gao, R., et al. (2010). “Lunar Regolith Simulant CUG-1A,” in 41st Lunar and Planetary Science Conference, Woodlands, Texas, USA, March 1-5, 2010 (The Woodlands, Texas: LPI Contribution). Abstr. #1183.
Heiken, G. H., Vaniman, D. T., and French, B. M. (1991). Lunar Sourcebook. Cambridge, United Kingdom: Cambridge Univ. Press. ISBN 0-521--33444-6.
Hopkins, W. G., and Huner, N. P. A. (2008). Introduction to Plant Physiology, 111 River Street, Hoboken, NJ: John Wiley & Sons.
Hoson, T., Kamisaka, S., Wakabayashi, K., Soga, K., Tabuchi, A., Tokumoto, H., et al. (2000). Growth Regulation Mechanisms in Higher Plants under Microgravity Conditions. Changes in Cell Wall Metabolism. Biol. Sci. Space 14, 75–96. doi:10.2187/bss.14.75
Hurowitz, J. A., Grotzinger, J. P., Fischer, W. W., McLennan, S. M., Milliken, R. E., Stein, N., et al. (2017). Redox Stratification of an Ancient lake in Gale Crater, Mars. Science 356, eaah6849. doi:10.1126/science.aah6849
Ivanova, T. N., Sapunova, S. M., Kostov, P. T., and Ilieva, I. I. (2005). “Recent Advances in the Development of the SVET Space Greenhouse Equipment,” in Proceedings of 2nd International Conference on Recent Advances in Space Technologies, 2005. RAST 2005, Istanbul, TR, June 9-11, 2005 (IEEE), 722–727. doi:10.1109/RAST.2005.1512662
Jones, S. B., and Or, D. (1999). Microgravity Effects on Water Flow and Distribution in Unsaturated Porous media: Analyses of Flight Experiments. Water Resour. Res. 35, 929–942. doi:10.1029/1998WR900091
Juilleret, J., Dondeyne, S., Vancampenhout, K., Deckers, J., and Hissler, C. (2016). Mind the gap: A Classification System for Integrating the Subsolum into Soil Surveys. Geoderma 264, 332–339. doi:10.1016/j.geoderma.2015.08.031
Kanamori, H., Udagawa, S., Yoshida, T., Matsumoto, S., and Takagi, K. (1998). “Properties of Lunar Soil Simulant Manufactured in Japan,” in Space 98. Reston, VA: American Society of Civil Engineers, 462–468. doi:10.1061/40339(206)53
Karl, D., Kamutzki, F., Zocca, A., Goerke, O., Guenster, J., and Gurlo, A. (2018). Towards the Colonization of Mars by In-Situ Resource Utilization: Slip Cast Ceramics from Martian Soil Simulant. PLoS One 13, e0204025. doi:10.1371/journal.pone.0204025
Kölbl, D., Pignitter, M., Somoza, V., Schimak, M. P., Strbak, O., Blazevic, A., et al. (2017). Exploring Fingerprints of the Extreme Thermoacidophile Metallosphaera Sedula Grown on Synthetic Martian Regolith Materials as the Sole Energy Sources. Front. Microbiol. 8, 1978. doi:10.3389/fmicb.2017.01918
Kozyrovska, N. O., Lutvynenko, T. L., Korniichuk, O. S., Kovalchuk, M. V., Voznyuk, T. M., Kononuchenko, O., et al. (2006). Growing pioneer Plants for a Lunar Base. Adv. Space Res. 37, 93–99. doi:10.1016/j.asr.2005.03.005
Lee, T. S. (2017). “In-Situ Resource Utilization (ISRU) Construction Technology for Moon and Mars,” in International MoonBase Summit (Mauna Lani Hotel and Bungalows Kohala Coast – Hawaiʻi’s Big Island: Sponsored by the (International Moonbase Alliance, LLC). Honolulu, Hawaii: International Moonbase Alliance, LLC.
Li, Y., Liu, J., and Yue, Z. (2009). NAO-1: Lunar Highland Soil Simulant Developed in China. J. Aerosp. Eng. 22, 53–57. doi:10.1061/(asce)0893-1321(2009)22:1(53)
Llorente, B., Williams, T., and Goold, H. (2018). The Multiplanetary Future of Plant Synthetic Biology. Genes 9, 348. doi:10.3390/genes9070348
Maggi, F., and Pallud, C. (2010). Martian Base Agriculture: The Effect of Low Gravity on Water Flow, Nutrient Cycles, and Microbial Biomass Dynamics. Adv. Space Res. 46, 1257–1265. doi:10.1016/j.asr.2010.07.012
Malla, R. B., Binienda, W. K., and Maji, A. K. (2006). Earth & Space 2006. League City/Houston, Texas: American Society of Civil Engineers. doi:10.1061/9780784408308
Marquit, J. D., Bates, S. C., Gushin, V. I., Synchev, V. N., Levinskikh, M. A., Podolsky, I. G., et al. (2008). “Testing Crew Responses to Varied Higher Plant Presentations in the MARS-500 Day Mission Simulation,” in Life in Space for Life on Earth. Editors L. Ouwehand, and F Angers (Cambridge, United Kingdom: ESA-SP). id. 33.
Matsushima, T., Katagiri, J., Uesugi, K., Tsuchiyama, A., and Nakano, T. (2009). 3D Shape Characterization and Image-Based DEM Simulation of the Lunar Soil Simulant FJS-1. J. Aerosp. Eng. 22, 15–23. doi:10.1061/(asce)0893-1321(2009)22:1(15)
McCollom, T. M., Robbins, M., Moskowitz, B., Berquó, T. S., Jöns, N., and Hynek, B. M. (2013). Experimental Study of Acid-Sulfate Alteration of Basalt and Implications for Sulfate Deposits on Mars. J. Geophys. Res. Planets 118, 577–614. doi:10.1002/jgre.20044
McFee, W. W., and VanScoyoc, G. E. (1975). The Nature and Properties of Soils, 8th Edition. Soil Sci. Soc. America J. 39, iv. doi:10.2136/sssaj1975.03615995003900050003x
McKay, D. S., Carter, J. L., Boles, W. W., Allen, C. C., and Allton, J. H. (1994). JSC-1: A New Lunar Soil Simulant. Eng. Constr. Oper. Sp. IV 2, 857–866.
McSween, H. Y., and Keil, K. (2000). Mixing Relationships in the Martian Regolith and the Composition of Globally Homogeneous Dust. Geochimica et Cosmochimica Acta 64, 2155–2166. doi:10.1016/S0016-7037(99)00401-9
Menezes, A. A., Cumbers, J., Hogan, J. A., and Arkin, A. P. (2015). Towards Synthetic Biological Approaches to Resource Utilization on Space Missions. J. R. Soc. Interf. 12, 20140715. doi:10.1098/rsif.2014.0715
Ming, D. W., Golden, D. C., and Henninger, D. L. (1993). Utilization of On-Site Resources for Regenerative Life Support Systems at Lunar and Martian Outposts. Angers, FR: SAE. Technical Paper, No. 932091. doi:10.4271/932091
Ming, D. W., and Henninger, D. L. (1989). Lunar Base Agriculture : Soils for Plant Growth. American Society of Agronomy, 255. ISBN 0-89118-100-8.
Ming, D. W., and Henninger, D. L. (1994). Use of Lunar Regolith as a Substrate for Plant Growth. Adv. Space Res. 14, 435–443. doi:10.1016/0273-1177(94)90333-6
Moore, H. J., Bickler, D. B., Crisp, J. A., Eisen, H. J., Gensler, J. A., Haldemann, A. F. C., et al. (1999). Soil-like Deposits Observed by Sojourner, the Pathfinder Rover. J. Geophys. Res. 104, 8729–8746. doi:10.1029/1998JE900005
Moore, H. J., and Jakosky, B. M. (1989). Viking landing Sites, Remote-Sensing Observations, and Physical Properties of Martian Surface Materials. Icarus 81, 164–184. doi:10.1016/0019-1035(89)90132-2
Mortley, D. G., Aglan, H. A., Bonsi, C. K., and Hill, W. A. (2000). Growth of Sweetpotato in Lunar and Mars Simulants. SAE. Technical Paper, (No. 2000-01-2289). doi:10.4271/2000-01-2289
NASA (2018). Pursuant to Section 432(b) of the NASA Transition Authorization Act of 2017. Washington, DC: National Space Exploration Campaign Report, 115-10.
Nechitailo, G., and Mashinsky, A. (1993). Space Biology: Studies at Orbital Stations. Moscow: Mir Publishers, 324–330.
Oze, C., Beisel, J., Dabsys, E., Dall, J., North, G., Scott, A., et al. (2021). Perchlorate and Agriculture on Mars. Soil Syst. 5, 37. doi:10.3390/soilsystems5030037
Peters, G. H., Abbey, W., Bearman, G. H., Mungas, G. S., Smith, J. A., Anderson, R. C., et al. (2008). Mojave Mars Simulant-Characterization of a New Geologic Mars Analog. Icarus 197, 470–479. doi:10.1016/j.icarus.2008.05.004
Primm, K. M., Gough, R. V., Wong, J., Rivera-Valentin, E. G., Martinez, G. M., Hogancamp, J. V., et al. (2018). The Effect of Mars-Relevant Soil Analogs on the Water Uptake of Magnesium Perchlorate and Implications for the Near-Surface of Mars. J. Geophys. Res. Planets 123, 2076–2088. doi:10.1029/2018JE005540
Rahmatian, L. A., and Metzger, P. T. (2010). “Soil Test Apparatus for Lunar Surfaces,” in Earth and Space 2010: Engineering, Science, Construction, and Operations in Challenging Environments (Reston, VA: American Society of Civil Engineers), 239–253. doi:10.1061/41096(366)25
Ramírez, D. A., Kreuze, J., Amoros, W., Valdivia-Silva, J. E., Ranck, J., Garcia, S., et al. (2019). Extreme Salinity as a challenge to Grow Potatoes under Mars-like Soil Conditions: Targeting Promising Genotypes. Int. J. Astrobiology 18, 18–24. doi:10.1017/S1473550417000453
Rickman, D., Edmunson, J., and McLemore, C. (2013). Functional Comparison of Lunar Regoliths and Their Simulants. J. Aerosp. Eng. 26, 176–182. doi:10.1061/(ASCE)AS.1943-5525.0000223
Rikken, G. B., Kroon, A. G. M., and van Ginkel, C. G. (1996). Transformation of (Per)chlorate into Chloride by a Newly Isolated Bacterium: Reduction and Dismutation. Appl. Microbiol. Biotechnol. 45, 420–426. doi:10.1007/s002530050707
Seiferlin, K., Ehrenfreund, P., Garry, J., Gunderson, K., Hütter, E., Kargl, G., et al. (2008). Simulating Martian Regolith in the Laboratory. Planet. Space Sci. 56, 2009–2025. doi:10.1016/j.pss.2008.09.017
Sibille, L., Carpenter, P., Schlagheck, R., and French, R. A. (2006). Lunar Regolith Simulant Materials: Recommendations for Standardization, Production, and Usage. Marshall Space Flight Center. NASA Technical Report.
Soil Survey Staff (2014). Keys to Soil Taxonomy. 12th ed. Washington, DC: U.S. Department of Agriculture, Natural Resources Conservation Service.
Stern, J. C., Sutter, B., Freissinet, C., Navarro-González, R., McKay, C. P., Archer, P. D., et al. (2015). Evidence for Indigenous Nitrogen in Sedimentary and Aeolian Deposits from the Curiosity Rover Investigations at Gale Crater, Mars. Proc. Natl. Acad. Sci. USA 112, 4245–4250. doi:10.1073/pnas.1420932112
Stevens, A. H., Steer, E., McDonald, A., Amador, E. S., and Cockell, C. S. (2018). Y-Mars: An Astrobiological Analogue of Martian Mudstone. Earth Space Sci. 5, 163–174. doi:10.1002/2017EA000318
Stoeser, D. B., Rickman, D. L., and Wilson, S. (2010a). “Design and Specifications for the highland Regolith Prototype Simulants NU-LHT-1m and-2M,” in NASA Technical Report Citeseer 21643. Huntsville, AL: National Aeronautics and Space Administration, Marshall Space Flight Center.
Stoeser, D. B., Wilson, S. A., and Rickman, D. L. (2010b). “Preliminary Geological Findings on the BP-1 Simulant,” in NASA Technical Report (Alabama, 35812: National Aeronautics and Space Administration IS20, George C. MarshNational Aeronautics and Space Administration IS20, George C. Marshall Space Flight Center, Marshall Space Flight Center), 3.
Suescun-Florez, E., Roslyakov, S., Iskander, M., and Baamer, M. (2015). Geotechnical Properties of BP-1 Lunar Regolith Simulant. J. Aerosp. Eng. 28, 04014124. doi:10.1061/(ASCE)AS.1943-5525.0000462
Sueyoshi, K., Watanabe, T., Nakano, Y., Kanamori, H., Aoki, S., Miyahara, A., et al. (2008). “Reaction Mechanism of Various Types of Lunar Soil Simulants by Hydrogen Reduction,” in Earth & Space 2008: Engineering, Science, Construction, and Operations in Challenging Environments (Reston, VA: American Society of Civil Engineers), 1–8. doi:10.1061/40988(323)134
Sun, H., Yi, M., Shen, Z., Zhang, X., and Ma, S. (2017). Developing a New Controllable Lunar Dust Simulant: BHLD20. Planet. Space Sci. 141, 17–24. doi:10.1016/j.pss.2017.04.010
Tang, H., Li, X., Zhang, S., Wang, S., Liu, J., Li, S., et al. (2017). A Lunar Dust Simulant: CLDS-I. Adv. Space Res. 59, 1156–1160. doi:10.1016/j.asr.2016.11.023
Taylor, L. A., Pieters, C., Keller, L. P., Morris, R. V., McKay, D. S., Patchen, A., et al. (2001). The Effects of Space Weathering on Apollo 17 Mare Soils: Petrographie and Chemical Characterization. Meteorit. Planet. Sci. 36, 285–299. doi:10.1111/j.1945-5100.2001.tb01871.x
Taylor, S. R. (2007). “The Moon,” in Encyclopedia Of the Solar System. Amsterdam, AL: Elsevier, 227–250. doi:10.1016/B978-012088589-3/50016-5
Thomas-Keprta, K. L., Clemett, S. J., Messenger, S., Ross, D. K., Le, L., Rahman, Z., et al. (2014). Organic Matter on the Earth's Moon. Geochimica et Cosmochimica Acta 134, 1–15. doi:10.1016/j.gca.2014.02.047
van Susante, P. J., and Dreyer, C. B. (2010). “Lunar and Planetary Excavation Prototype Development and Testing at the Colorado School of Mines,” in Earth and Space 2010: Engineering, Science, Construction, and Operations in Challenging Environments (Reston, VA: American Society of Civil Engineers, 1191–1200. doi:10.1061/41096(366)109
Verseux, C., Baqué, M., Lehto, K., de Vera, J.-P. P., Rothschild, L. J., and Billi, D. (2016). Sustainable Life Support on Mars - the Potential Roles of Cyanobacteria. Int. J. Astrobiology 15, 65–92. doi:10.1017/S147355041500021X
Walkinshaw, C. H., and Johnson, P. H. (1971). Analysis of Vegetable Seedlings Grown in Contact with Apollo 14 Lunar Surface Fines. HortScience 6, 532–535.
Walkinshaw, C. H., Sweet, H. C., Venketeswaran, S., and Horne, W. H. (1970). Results of Apollo 11 and 12 Quarantine Studies on Plants. Bioscience 20, 1297–1302. doi:10.2307/1295365
Wallace, W. T., Taylor, L. A., Liu, Y., Cooper, B. L., McKay, D. S., Chen, B., et al. (2009). Lunar Dust and Lunar Simulant Activation and Monitoring. Meteorit. Planet. Sci. 44, 961–970. doi:10.1111/j.1945-5100.2009.tb00781.x
Wamelink, G. W. W., Frissel, J. Y., Krijnen, W. H. J., and Verwoert, M. R. (2019). Crop Growth and Viability of Seeds on Mars and Moon Soil Simulants. Open Agric. 4, 509–516. doi:10.1515/opag-2019-0051
Wamelink, G. W. W., Frissel, J. Y., Krijnen, W. H. J., Verwoert, M. R., and Goedhart, P. W. (2014). Can Plants Grow on Mars and the Moon: A Growth Experiment on Mars and Moon Soil Simulants. PLoS One 9, e103138. doi:10.1371/journal.pone.0103138
Weete, J. D., and Walkinshaw, C. H. (1972). Apollo 12 Lunar Material: Effects on Plant Pigments. Can. J. Bot. 50, 101–104. doi:10.1139/b72-015
Weete, J. D., Walkinshaw, C. H., and Laseter, J. L. (1972). Apollo 12 Lunar Material: Effects on Lipid Levels of Tobacco Tissue Cultures. Science 175, 623–624. doi:10.1126/science.175.4022.623
Zaets, I., Burlak, O., Rogutskyy, I., Vasilenko, A., Mytrokhyn, O., Lukashov, D., et al. (2011). Bioaugmentation in Growing Plants for Lunar Bases. Adv. Space Res. 47, 1071–1078. doi:10.1016/j.asr.2010.11.014
Zeng, X., He, C., and Wilkinson, A. (2010). Geotechnical Properties of NT-LHT-2m Lunar Highland Simulant. J. Aerosp. Eng. 23, 213–218. doi:10.1061/(ASCE)AS.1943-5525.0000026
Zeng, X., Li, X., Wang, S., Li, S., Spring, N., Tang, H., et al. (2015). JMSS-1: a New Martian Soil Simulant. Earth Planet. Sp 67, 72. doi:10.1186/s40623-015-0248-5
Zheng, Y., Wang, S., Ouyang, Z., Zou, Y., Liu, J., Li, C., et al. (2009). CAS-1 Lunar Soil Simulant. Adv. Space Res. 43, 448–454. doi:10.1016/j.asr.2008.07.006
Keywords: in situ resource utilization, regolith simulants, space exploration, Moon, Mars, extra-terrestrial farming, bioregenerative life support systems, food production
Citation: Duri LG, Caporale AG, Rouphael Y, Vingiani S, Palladino M, De Pascale S and Adamo P (2022) The Potential for Lunar and Martian Regolith Simulants to Sustain Plant Growth: A Multidisciplinary Overview. Front. Astron. Space Sci. 8:747821. doi: 10.3389/fspas.2021.747821
Received: 26 July 2021; Accepted: 29 November 2021;
Published: 04 January 2022.
Edited by:
Lucie Poulet, NASA Postdoctoral Program, United StatesReviewed by:
Andrew George Palmer, Florida Institute of Technology, United StatesCopyright © 2022 Duri, Caporale, Rouphael, Vingiani, Palladino, De Pascale and Adamo. This is an open-access article distributed under the terms of the Creative Commons Attribution License (CC BY). The use, distribution or reproduction in other forums is permitted, provided the original author(s) and the copyright owner(s) are credited and that the original publication in this journal is cited, in accordance with accepted academic practice. No use, distribution or reproduction is permitted which does not comply with these terms.
*Correspondence: Antonio Giandonato Caporale, YWcuY2Fwb3JhbGVAdW5pbmEuaXQ=
†These authors have contributed equally to this work and share first authorship
Disclaimer: All claims expressed in this article are solely those of the authors and do not necessarily represent those of their affiliated organizations, or those of the publisher, the editors and the reviewers. Any product that may be evaluated in this article or claim that may be made by its manufacturer is not guaranteed or endorsed by the publisher.
Research integrity at Frontiers
Learn more about the work of our research integrity team to safeguard the quality of each article we publish.