- 1Institute of Space Physics and Applied Technology, Peking University, Beijing, China
- 2State Key Laboratory of Space Weather, National Space Science Center, Chinese Academy of Sciences, Beijing, China
- 3Space Plasma Physics Group, Department of Physics and Technology, University of Bergen, Bergen, Norway
- 4Department of Electromagnetism and Electronics, University of Murcia, Murcia, Spain
- 5Institut de Recherche en Astrophysique et Planétologie, Université de Toulouse, CNRS, UPS, CNES, Toulouse, France
- 6Swedish Institute of Space Physics, Uppsala, Sweden
- 7Physics and Astronomy Department, Vanderbilt University, Nashville, TN, United States
- 8Physics Department E. Fermi, University of Pisa, Pisa, Italy
- 9Southwest Research Institute, San Antonio, TX, United States
- 10Department of Physics and Astronomy, University of Texas at San Antonio, San Antonio, TX, United States
- 11NASA Goddard Space Flight Center, Greenbelt, MD, United States
- 12Max-Planck Institute for Solar Systems Research, Göttingen, Germany
- 13Birkeland Center for Space Science, University of Bergen, Bergen, Norway
- 14The University Centre in Svalbard, Longyearbyen, Svalbard
- 15Institute for the Study of Earth Oceans and Space, University of New Hampshire, Durham, NH, United States
- 16Laboratoire d’Astrophysique de Bordeaux, Univ. Bordeaux, Pessac, France
- 17Applied Physics Laboratory, Johns Hopkins University, Laurel, MD, United States
Cold (few eV) ions of ionospheric origin are widely observed in the lobe region of Earth’s magnetotail and can enter the ion jet region after magnetic reconnection is triggered in the magnetotail. Here, we investigate a magnetotail crossing with cold ions in one tailward and two earthward ion jets observed by the Magnetospheric Multiscale (MMS) constellation of spacecraft. Cold ions co-existing with hot plasma-sheet ions form types of ion velocity distribution functions (VDFs) in the three jets. In one earthward jet, MMS observe cold-ion beams with large velocities parallel to the magnetic fields, and we perform quantitative analysis on the ion VDFs in this jet. The cold ions, together with the hot ions, are reconnection outflow ions and are a minor population in terms of number density inside this jet. The average bulk speed of the cold-ion beams is approximately 38% larger than that of the hot plasma-sheet ions. The cold-ion beams inside the explored jet are about one order of magnitude colder than the hot plasma-sheet ions. These cold-ion beams could be accelerated by the Hall electric field in the cold ion diffusion region and the shrinking magnetic field lines through the Fermi effect.
1 Introduction
Cold (few eV) ions of ionospheric origin are widely present in the Earth’s magnetosphere (Chappell et al., 1980; Moore et al., 1997; Cully et al., 2003). In the lobes of the magnetosphere, spacecraft can be positively charged to several tens of volts due to photoelectron emissions. The positive potential prevents cold ions from reaching onboard ion instrument. With large-scale convective motion, for example, magnetopause fluctuation or plasma-sheet flapping, cold ions can be accelerated via the E×B drift to overcome the positive spacecraft potential (Sauvaud et al., 2004), and be detected by an ion instrument. Besides direct measurement with particle instruments, cold ions can also be inferred by a technique based on the wake signal of a charged spacecraft in a supersonic ion flow (Engwall et al., 2009; Li et al., 2013; André et al., 2015). Observational statistics show that cold ions dominate the plasma density in large regions of the nightside magnetosphere (e.g., lobe region) and polar regions (André and Cully, 2012). At times, the cold ions can also dominate in the dayside magnetosphere. Cold ions can play an important role in the solar wind-magnetosphere-ionosphere coupling system, including the terrestrial plasma escape and circulation and the magnetotail dynamics (Welling et al., 2015; Kistler and Mouikis, 2016; Yamauchi, 2019).
Magnetic reconnection is a fundamental and universal process to convert energy stored in the magnetic field into kinetic and thermal energies of charged particles (Priest and Forbes, 2007; Yamada et al., 2010). Magnetic reconnection is the major transport mechanism of mass, energy, and magnetic flux in the solar wind-magnetosphere coupling system, and is the predominant cause of the geomagnetic activity. In the past decade, the effects of cold ions on magnetic reconnection at the dayside magnetopause and in the magnetotail have been extensively analyzed via using in-situ measurements from multiple spacecraft missions (Wang et al., 2014; Toledo-Redondo et al., 2015; André et al., 2016; Li et al., 2017; Toledo-Redondo et al., 2021) and types of numerical simulation models (Aunai et al., 2011; Divin et al., 2016; Tenfjord et al., 2019; Dargent et al., 2019, 2020). At the dayside magnetopause, magnetic reconnection is typically asymmetric due to the large differences in plasma and magnetic field conditions between the magnetosheath and the magnetosphere. The gyroradii and the inertial lengths of the magnetosheath plasmas usually determine the two kinetic scales of the diffusion region, namely, ion and electron diffusion regions (Yamada et al., 2010). With the presence of cold-ion inflow from the magnetospheric side, cold ions bring a new diffusion region between the usual ion and electron diffusion regions (Toledo-Redondo et al., 2016a; Divin et al., 2016), and can push the reconnection jet towards the magnetosheath side (Cassak and Shay, 2007; Walsh et al., 2014). Li et al. (2017) present the first observation of the cold-ion outflows throughout the entire reconnection region via investigating the three-dimensional (3D) ion velocity distribution functions (VDFs). Cold-ion jets with high parallel velocities are formed on the magnetosheath side of the reconnection exhaust, and those cold ions remain relatively cold compared with the magnetosheath ions. Li et al. (2017) suggest that those fast cold-ion jets originate from the cold-ion inflow close to the X line, while the acceleration mechanism lacks detailed analysis.
In the magnetotail, the magnetic fields are oppositely directed on each side of the plasma sheet, and the plasma-sheet plasmas are hot with ion temperature of several to tens of keV. Magnetic reconnection is symmetric with homogeneous hot ion inflows from both sides. The plasma sheet boundary layer (PSBL) and lobe region always contain a certain amount of cold ions of ionospheric origin, which can affect the reconnection process in the magnetotail. One prominent feature is that cold ions create highly structured ion VDFs far from the Maxwellian shape. Among these distributions, counter-streaming cold-ion beams along the direction normal to the current sheet are frequently observed in the ion diffusion region both in observations and simulations (Fujimoto et al., 1996; Hoshino et al., 1998; Nagai et al., 1998; Shay et al., 1998; Wygant et al., 2005; Divin et al., 2016; Dai et al., 2021). The counter-streaming cold-ion beams are suggested to be accelerated by the Hall electric field along the normal direction at the separatrix, which is mostly along Z direction of Geocentric Solar Ecliptic (GSE) coordinates. The electric potential well across the separatrix is
Besides the acceleration by the Hall electric field in the ion diffusion region, the Fermi mechanism (slingshot effect) and the reconnection electric field can also be important for cold-ion acceleration in the reconnection exhaust. Several studies report counter-streaming cold-ion beams observed in bursty bulk flows (BBFs) and analyze acceleration mechanisms and ion anisotropic instabilities associated with these beams (Eastwood et al., 2015; Hietala et al., 2015; Birn et al., 2017; Runov et al., 2017; Xu et al., 2019). BBFs are widely interpreted as magnetic reconnection jets (Angelopoulos et al., 1992; Nagai et al., 1998; Birn et al., 2011) and can transport significant amount of magnetic fluxes and plasmas towards Earth (Baumjohann et al., 1990; Angelopoulos et al., 1992, 1994). Eastwood et al. (2015) find that counter-streaming beams are sourced from the thermal population in the preexisting plasma sheet and are accelerated by the reconnection electric field. Birn et al. (2017) show that the beam ions are from the low-energy lobe regions. These beams are firstly accelerated by the E×B drift motion and then by the slingshot effect of the earthward convecting BBF. A similar process is also described by Xu et al. (2019). In the previous studies, the counter-streaming cold-ion beams are mostly observed near the front edge of the reconnection jet, and quantitative studies analyzing the acceleration are primarily via numerical simulations. The existence and dynamics of the cold ions in an entire reconnection jet still lack quantitative analysis using in-situ observations. In this study, we perform a more comprehensive survey of ion VDFs in a magnetotail crossing by the Magnetospheric Multiscale (MMS) spacecraft. Various types of ion VDFs with cold-ion beams are observed inside a magnetic reconnection jet, and plasma partial moments of the cold ions are computed to investigate their dynamics through the reconnection process.
2 Observations
In-situ observations from NASA’s MMS mission (Burch et al., 2016) are utilized for this study. MMS was launched on March 13, 2015 and consists of four identical spacecraft designed to study the kinetic-scale physics of magnetic reconnection in the Earth’s magnetosphere. In this study, we use magnetic field data from the FluxGate Magnetometer (FGM) (Russell et al., 2016) and electric field data from the Electric Double Probe (EDP) (Ergun et al., 2016; Lindqvist et al., 2016). The ion data are from the Fast Plasma Investigation (FPI) (Pollock et al., 2016) sampled at 4.5 s for the fast mode and 0.15 s for the burst mode, and also, from the mass-resolved instrument Hot Plasma Composition Analyzer (HPCA) (Young et al., 2016) at 10 s resolution.
We investigate an MMS magnetotail crossing from 21:30:00 to 24:00:00 UT on July 06, 2017. The four spacecraft moved from [−24.6, −0.8, 5.2] RE (Earth radii) to [−24.5, −1.4, 5.4] RE (GSE) during this time interval. Owing to a small spacecraft separation ( ∼15 km), observations from the four spacecraft are nearly identical, and thus, we show results primarily from MMS1. An overview of the MMS1 observations is presented in Figure 1, and the data are in fast mode. The panels from top to bottom show 1) magnetic field B, 2) number densities of ions and electrons, 3) ion bulk velocities from FPI, 4) FPI ion omnidirectional differential energy flux, and 5) number densities of He++ and O+ ions from HPCA. In Figure 1B, the number densities of H+ from HPCA, all ions from FPI and electrons from FPI are shown in black, blue and red, respectively. The three curves almost overlap with each other, indicating reliable number density results. The MMS fleet is initially located in the plasma sheet, characterized by weak magnetic field (Figure 1A) and high-density ( ∼0.5 cm−3) and hot ( ∼4,500 eV) ions. From 21:49:00 to 22:02:00 UT, MMS1 observes a strong tailward ion flow, with a peak VX reaching -1,000 km s−1. Such a strong ion flow indicates ongoing reconnection and that the spacecraft are located tailward of the reconnection X line. In this tailward ion jet, the BX component is mostly positive, indicating that MMS cross the northern part of the ion jet.
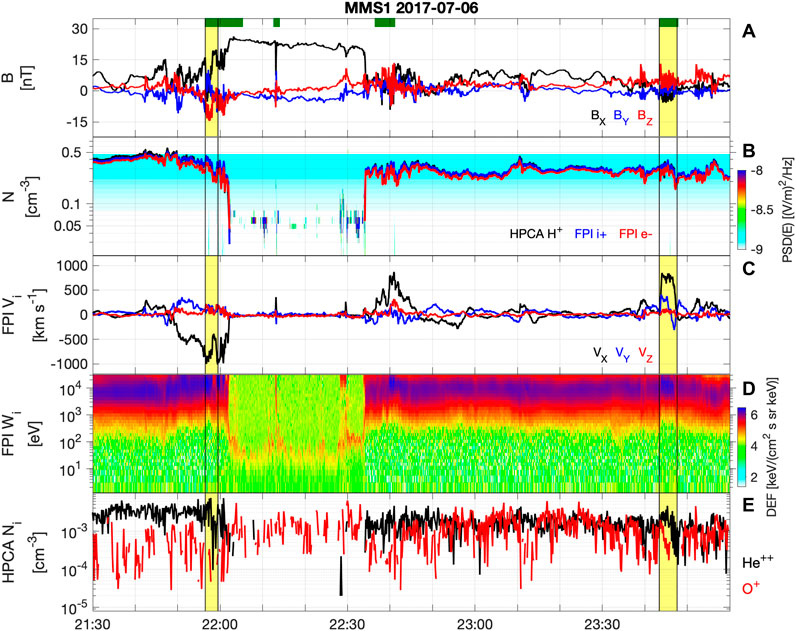
FIGURE 1. MMS observation of the Earth’s magnetotail on July 06, 2017. MMS1 data of (A) magnetic field B, (B) number densities of hydrogen (H+, black) from HPCA, all ions (blue) and electrons (red) from FPI and electron number density derived from the Langmuir waves observed by EDP, (C) ion bulk velocity Vi from FPI, (D) FPI ion omnidirectional differential energy flux, (E) number densities of He++ (black) and O+ (red) ions from HPCA. All vectors are presented in GSE coordinates, which are close to Geocentric Solar Magnetospheric (GSM) coordinates in this event. The horizontal green bars in the top of (A) denote the burst-mode time intervals, and detailed analysis of the ion VDFs in the two yellow-shaded intervals are presented in the following figures.
At 22:02:00 UT, we identify a separatrix of the reconnection exhaust, where B increases, plasma number density decreases, ion bulk velocity decreases, and energetic particles vanish (see Supplementary Figure S1 in Supplementary Material for more details). Then, MMS1 enters the lobe region until 22:34:00 UT. As shown by the ion energy flux in Figure 1D, cold ions of ionospheric origin, with ∼10 eV thermal energy, are present in the lobe region, while their total energy can reach 100 eV due to the E×B drift motion. In the lobe, the penetrating radiation leads to a nearly constant background from the lowest to the highest energy channels in the FPI ion measurement unit (Gershman et al., 2019), as seen by the green fluxes in Figure 1D. Thus, the FPI ion number density from the integral over the whole energy range is overestimated. Besides, the FPI electron measurement unit cannot provide reliable electron moment data in the lobe because of the low thermal energy and the photoelectron contamination (Gershman et al., 2019). So, we take the number density (0.03–0.05 cm−3) estimated from the waves at the electron plasma frequencies, as illustrated by the color-coded spectrum in Figure 1B. In the lobe, oxygen ions measured by HPCA have number densities about 0.0005–0.002 cm−3 (Figure 1E), and hydrogen is thus the primary ion species in terms of number density. From 22:13:04 to 22:13:15 UT, MMS cross a separatrix of the reconnection exhaust back and forth quickly (see Supplementary Figure S2 in Supplementary Material for details). Based on the observations during this time interval, Alm et al. (2018) analyzed the role of cold ions in the separatrix layer. They find that the cold ions account for ∼ 30% of the total ion number density and can significantly influence the Hall physics of reconnection. After 22:34:00 UT, MMS1 crosses back to the plasma sheet and sees two strong earthward ion jets, with aVX peak reaching 860 km s−1. O+ ions, as tracers of cold ionospheric ions, are continuously observed inside the plasma sheet shown in Figure 1, and have similar number densities with those in the lobe. Meanwhile, He++ ions can be used as tracers of solar wind ions, and are also continuously observed in the plasma sheet. Thus, both the ionospheric and solar-wind ions are the ion sources of the plasma sheet in our event, even through the two sources are indistinguishable from the FPI ion energy flux panel (Figure 1D). We explore the 3D ion VDFs in the tailward and earthward reconnection jets and characterize different types of ion VDFs with cold-ion beams. In the following, we present results from the two time intervals indicated by the yellow-shaded bars in Figure 1.
Figures 2A–F present an overview of the tailward ion jet in the time interval indicated by the left yellow-shaded bar in Figure 1. Here, burst-mode data are used. In this jet, VX varies between −1,240 km s−1 and −430 km s−1, and ions with distinct energy fluxes are all above 500 eV in the spacecraft frame (Figure 2D). Figure 2E shows the phase space density of the reduced ion VDF projected on to the magnetic field directions, and the black curve represents the parallel bulk velocity
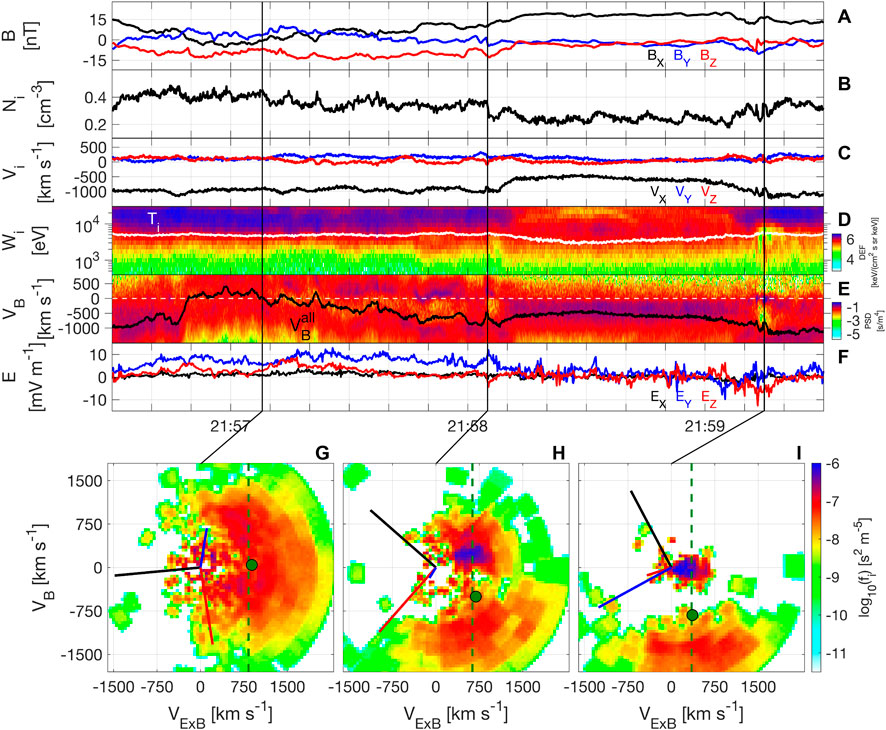
FIGURE 2. Cold ions inside the tailward ion jet. (A) B. (B) Ni. (C) Vi. (D) FPI ion omnidirectional differential energy flux with ion temperature (white curve). (E) Reduced ion VDF integrated over the directions perpendicular to the magnetic field. The black solid curve shows the parallel ion bulk speed
From 21:57:12 UT to 21:58:10 UT, MMS1 also observes two distinct populations in the reduced ion VDFs shown in Figure 2E, even though the two populations are not distinguishable from the FPI ion energy flux (Figure 2D). Figure 2H displays an example of the ion VDFs in this time interval. The properties of the hot population are similar to those shown in Figure 2I. The cold population follows E×B drift ( ∼630 km s−1) motion and has a small ( ∼200 km s−1) positive VB. Its bulk velocity is predominantly along the -Z direction in GSE. These cold-ion beams may originate from the northern lobe. A small bulk speed ( ∼660 km s−1) of this cold population suggests that those beams may be not from the cold-ion inflow close to the X line. They may experience an acceleration process as discussed previously by Birn et al. (2017). The cold ions are probably accelerated by the E×B drift motion when crossing a distant separatrix, and are further accelerated by the Fermi mechanism as the cold ions convect together with the shrinking magnetic field lines. Another prominent feature between the cold populations shown in Figures 2H,I is that the cold population in Figure 2H is clearly thermalized, and we discuss that in the following section. In the earthward ion jet between 22:36:00 UT and 22:47:00 UT, MMS observe similar features of the ion VDFs as those presented in Figure 2.
MMS see much more complicated features of cold-ion beams in the earthward ion jet between 23:43:23 UT and 23:47:53 UT (denoted by the right yellow-shaded bar in Figure 1). Figures 3A–F display a detailed overview of this ion jet using burst-mode data. MMS are originally located in the stagnant plasma sheet. When a dipolarization front (Nakamura et al., 2002; Runov et al., 2009; Fu et al., 2013) arrives at MMS at ∼23:43:34 UT, the plasma density drops from 0.31 cm−3 to 0.20 cm−3 and the magnetic field BZ component increases from 5 to 13 nT. The earthward flow speed subsequently increases to its maximum values, with aVX peak reaching 1,040 km s−1. This kind of fast ion flow is frequently interpreted as an earthward bursty bulk flow (BBF) driven by magnetic reconnection with an X line located tailward of the spacecraft. In Figure 3, we use a yellow-shaded bar to highlight the BBF or the jet interval. The average Ni of the jet is approximately 0.29 cm−3, which is similar to that of the nearby plasma sheet. The average Ti is 3.8 keV, with a small temperature anisotropy (Ti‖/Ti⊥ ∼0.92). After a detailed survey of all the ion VDFs, we find four types of ion VDFs inside the jet shown in Figure 3: Type I consists of only a single hot plasma-sheet population (see an example shown by Figure 3H), Type II consists of a hot population and a cold population with a positive VB (see Figure 3I), Type III consists of a hot population and a cold population with a negative VB (see Figure 3J), and Type IV consists of a hot population with counter-streaming cold populations (see Figure 3K). We categorize all the ion VDFs of this earthward ion jet, and the results are presented by colored dots in Figure 3G. In Figures 3H–K, the green dots denote the projected ion bulk velocities in the VE×B-VB plane, and the green vertical dashed lines represent the E×B drift speeds. The small discrepancy between the two speeds is likely from the errors (0.5–1.0 mV m−1) in electric field (Lindqvist et al., 2016) or could be caused by weak demagnetization of the ions. In GSE coordinates, all ions propagate earthward (illustrated by the black lines in Figures 3H–K). The large positive and negative VB components of the cold ions correspond to the large positive and negative VZ components (Figures 3I–K), respectively.
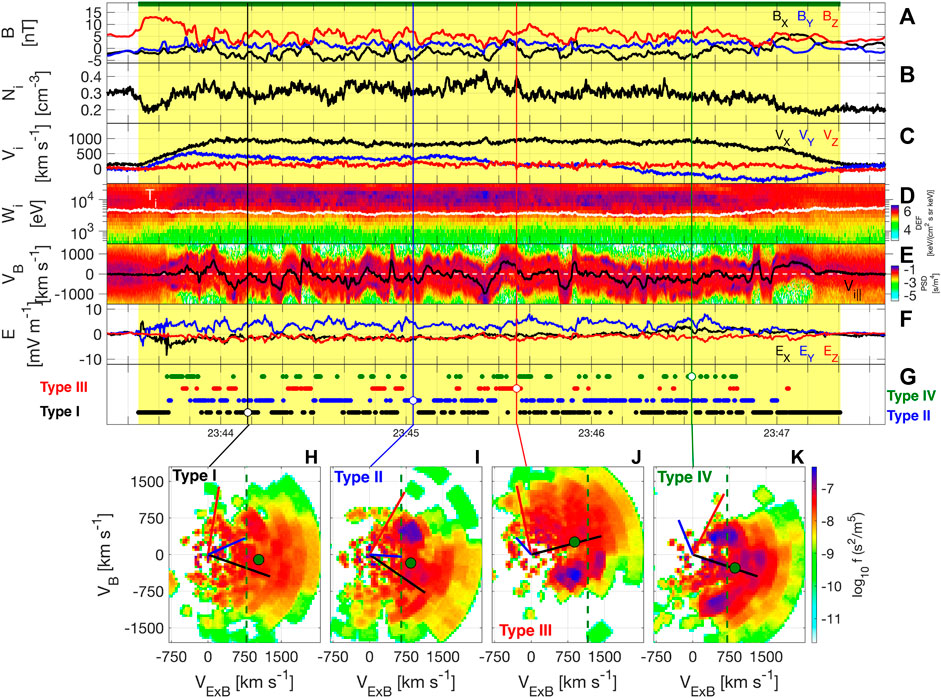
FIGURE 3. Four types of ion velocity distribution functions (VDFs) in the earthward ion jet. (A) B. The green horizontal bar denotes the ion jet interval. (B) Ni. (C) Vi. (D) FPI ion omnidirectional differential energy flux with ion temperature (white curve). (E) Reduced ion VDF integrated over the directions perpendicular to the magnetic field. The black solid curve shows the parallel ion bulk speed Vi‖, and the white dashed line denotes Vi‖ = 0. (F) Electric field E. (G) Ion VDF types inside the ion jet. (H)–(K) Examples of the four-type ion VDFs at times indicated by the four vertical lines in (A)–(G). (H)–(K) are in the same format with Figures 2G–I.
Previous studies like Eastwood et al. (2015) and Xu et al. (2019) explored the kinetic behavior of counter-streaming cold ions at the leading edges of the reconnection jets, with particular focus on the vicinity of dipolarization front. The cold-ion beams investigated in their works may originate from ions in the preexisting plasma sheet or the ionosphere and are swept up directly by a dipolarization front. In our study, we do not find the existence of isolated cold-ion beams in the region close to the dipolarization front, which could be due to the phase-space mixing by cold-ion thermalization. We find four types of ion VDFs widely distributed over the entire observed part of the jet. The cold-ion beams have total energies all above ∼3 keV and mix together with the plasma-sheet hot ions in the spectrogram of the differential energy flux (Figure 3D), and one can only distinguish cold ions in three-dimensional VDFs. This is different from the cold ions reported in Eastwood et al. (2015) and Xu et al. (2019), where the total energies of cold ions are lower than those of hot ions and one can easily see them in the spectrograms of the differential energy fluxes.
In the magnetotail, the BX component can be used as a guide to determine the normal distance to the plasma-sheet neutral line (BX = 0). Figure 4A shows the count profiles of the four types of ion VDFs along BX. MMS spend most of the time in the southern exhaust, and most of the counts are therefore in the negative BX region, where Type I and Type II VDFs clearly dominate. Figure 4B presents the occurrence rates of the four-type ion VDFs, and the gray shadow highlights the results with total counts over 50. Despite the large variation due to relatively low counts, one can conclude that the occurrence rate of Type I is nearly constant. The occurrence rate of Type II decreases gradually along BX or from the southern to the northern sides of the neutral line. Conversely, the occurrence rate of Type III increases gradually along BX. The occurrence rate of Type IV peaks at the neutral line. Therefore, the antiparallel beams are predominantly observed in the northern side of the neutral sheet, and the parallel beams are predominantly observed on the southern side. The majority of cold-ion beams were found to be approaching the neutral sheet, with a smaller subset of VDFs also showing cold ion populations moving away from the neutral sheet. This indicates that there is some mechanism that either prevents cold ions from crossing far into the opposite exhaust, that the cold-ion populations are no longer cold after the neutral sheet crossing (heating), or that they can not be distinguished from the hotter background.
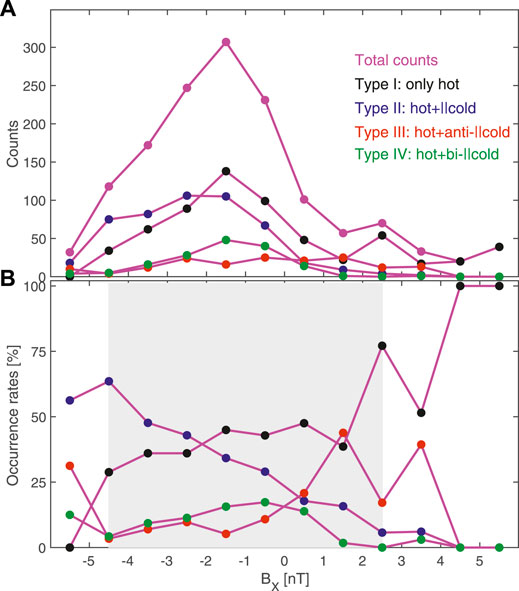
FIGURE 4. Statistics of the four types of ion VDFs along BX. (A) Counts profiles along BX. The magenta curve shows the total counts, and the rest of the curves show the four types of VDFs. (B) Occurrence rates. The gray shadow highlights the interval with total counts over 50.
The distribution of VDFs shown in Figure 4B may be generated by several processes. First, MMS observe the northern (see Figure 1) and southern lobes on July 6,2,017 and see cold ions in both lobes. As an example shown in Figure 1D, the energy fluxes of the cold ions in the lobe are intermittent, indicating that the cold ions inflowing into the reconnection region could be patchy. Secondly, when cold ions enter the separatrices close to the X line (e.g., the cold-ion diffusion region), numerical simulations (Aunai et al., 2011; Divin et al., 2016) show that the cold ions bounce within an electrostatic potential well between the separatrices. The distribution feature in Figure 4B could be a statistical result of the bouncing motions of the cold ions inflowing from both lobes. Finally, as shown in Figure 3A, MMS see large-amplitude fluctuations in magnetic field, which may be convected from the upstream exhaust region or be driven by the ion temperature anisotropy associated with cold-ion beams (Hietala et al., 2015). Besides, the minimum curvature radii of the magnetic field lines in this event are comparable to the gyroradii of the cold ions (as shown by Figure 5H). Plasma waves and the curved magnetic field lines with small curvature radii can scatter and thermalize the cold ions when moving from one side of the neutral line to the other side, and thus, can mix them with the plasma-sheet hot ions. The distributions in Figure 4B could be combinational results of those three effects.
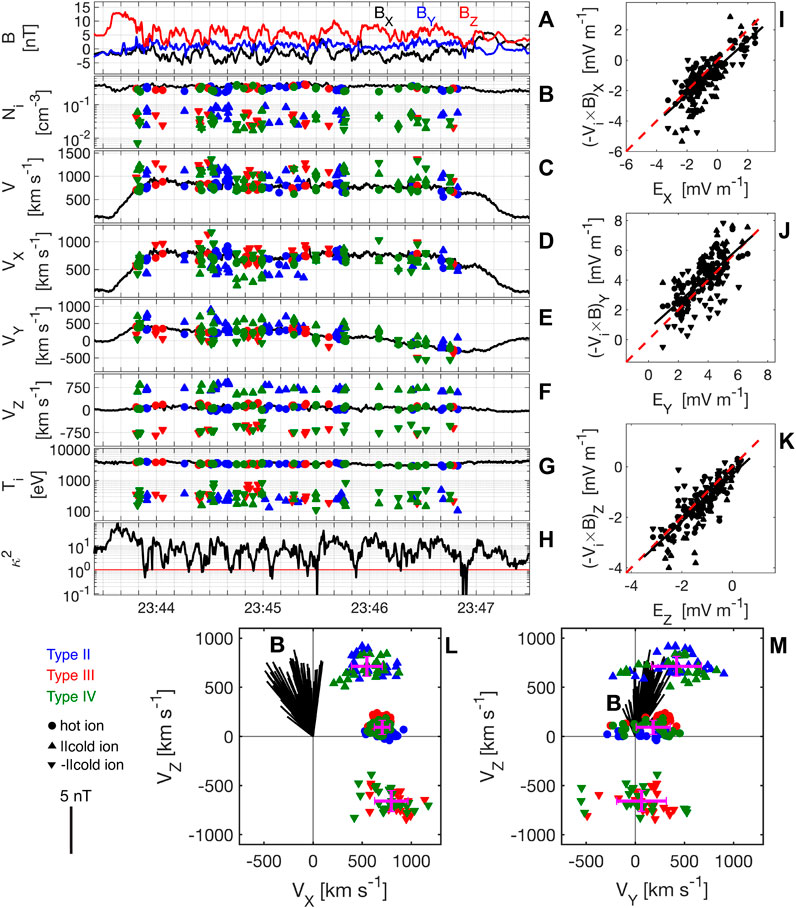
FIGURE 5. Partial moments of the cold-ion beams and the hot ions inside the earthward ion jet. Those ions are from the three types (Types II, III and IV) with cold ions. Types II, III and IV are colored in blue, red and green, respectively. The partial-moment results of the hot, parallel cold and anti-parallel cold ions are marked by circles, upward-pointing triangles and downward-pointing triangles, respectively. (A) B. (B) Ni of all ions (black curve) and cold and hot ions from partial moments. (C) Ion bulk speed V. (D)–(F) Three components of the ion bulk velocities Vi. The black curves are the average bulk velocities. (G) Ti. (H) Adiabatic parameter defined by κ2 =
3 Quantification of Cold-Ion Beams in the Earthward Jet
In order to study the dynamics of the cold-ion beams inside the jet, we utilize the same technique as used in Li et al. (2017) (see supporting information of that paper and Supplementary Figure S3 in Supplementary Material for more details) to compute partial moments of the hot and cold populations. First, we select data points of the three types of ion VDFs with cold ions with most coverage of the variant magnetic field conditions. Then, we separate the cold and hot populations for each ion VDF in three-dimensional velocity space and compute their partial moments, including number density, bulk velocity and ion temperature. The results are presented in Figure 5, and the average results with standard deviations are listed in Table 1. In Figures 5B–G, L,M, the partial-moment results of the three-type VDFs (Types II, III and IV) with cold ions are labeled in blue, red and green, respectively. The hot ions from all three types are marked by circles. The parallel cold-ion beams from Types II and IV VDFs are marked by the upward-pointing triangles, while the anti-parallel beams from Types III and IV are marked by the downward-pointing triangles. The black lines in Figures 5L,M denote the magnetic fields of the selected data points for the partial-moment calculations. Figures 5I–K show the comparison between the measured electric field from EDP and the convection electric field (-Vi×B) of the hot and cold ions from our partial-moment calculations. A good consistency of the three components means that the ions in the investigated jet are mainly frozen-in with the magnetic field lines. Also, it demonstrates a relatively good reliability of our partial-moment calculation via separating multiple populations from one VDF.
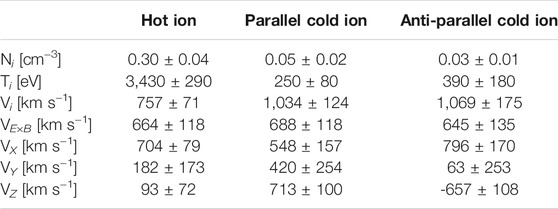
TABLE 1. Average partial moments of the hot and cold ions of the selected data points shown in Figure 5
As presented in Table 1, the average number density ( ∼0.04 cm−3) of the cold-ion beams is comparable to that of the cold ions in the lobe and approximately 13% of that of the hot ions inside the jet. Even though the cold ions have large peak VDFs in velocity space, they are still minor populations in this investigated event. As shown by the circles in Figure 5C, the bulk velocities of the hot ions from the partial-moment calculations are close to the average bulk velocities (black curve) of all ions. This is consistent with the partial-moment number density results. All of the hot ions convect earthward, and their speed
It is difficult to know the acceleration process from the observational aspect. Here, we find a similarity between the velocity patterns in Figures 5L,M and previous numerical results (Aunai et al., 2011; Divin et al., 2016) and suggest that the cold ions inside this investigated jet may come from the region close to the X line. Cold ions are demagnetized while crossing the separatrices close to the X line (Toledo-Redondo et al., 2016a; Divin et al., 2016), and are accelerated primarily by the Hall electric field, while the beams can remain relatively cold. The cold-ion beams may bounce for several times within the Hall electrostatic potential well, and get significant VZ and VX components. Then, the accelerated cold-ion beams could be further accelerated by the shrinking magnetic field lines due to the Fermi effect. One can find a characteristic example of such acceleration process from Figure 5 of Divin et al. (2016). This process could be responsible for the 38% extra speed at the MMS observation location.
Using Cluster data, Toledo-Redondo et al. (2016b) showed that cold ions can be heated by the large electric field gradient when crossing a separatrix boundary. Graham et al. (2017) analyzed a magnetopause reconnection event with cold ions and found lower-hybrid waves at the separatrix driven by the ion-ion streaming instability between the cold ions and the magnetosheath ions. The lower-hybrid waves can contribute to the cold-ion heating. Norgren et al. (2021) showed that the cold ions can be heated through a combination of thermalization at the separatrices and pitch angle scattering in the curved magnetic field around the neutral plane. Here, we calculate the temperature ( ∼320 eV) of the cold-ion beams inside the reconnection jet, which corresponds to about 6% of the total energy in the spacecraft frame. The cold-ion beams are approximately one order of magnitude colder than the hot ions, while the cold-ion beams are thermalized compared to the cold ions ( ∼10 eV) in the lobe. The large electric-field gradient at the separatrix and the scattering effect by the kinetic waves (see Supplementary Figure S4 in Supplementary Material for more details) and small magnetic-field curvature radii (Figure 5H) may all contribute to the heating of the observed cold ions from the lobe into the ion jet. However, one should note that the temperature results have a large uncertainty from our partial-moment calculation. As shown in Figures 3; Supplementary Figures S3, the thermal parts of the hot and cold populations usually overlap with each other. We cut major parts of the cold-ion VDFs empirically by eye. The results of the number density and bulk velocity are relatively reliable, but the ambient treatment of the VDF boundaries brings large errors to the temperature. The temperature results can be improved by fitting the cold-ion VDFs in the three-dimensional velocity space, which will be tried in our future works.
4 Conclusion
We investigated an MMS magnetotail crossing from 21:30:00 UT to 24:00:00 UT on July 06, 2017 with cold ions and ongoing magnetic reconnection and present quantitative analysis of the cold ions inside a reconnection ion jet. In this event, MMS cross one tailward and two earthward ion jets, and we explored the three-dimensional ion VDFs and find types of ion VDFs with cold-ion beams. MMS observe hot ions of plasma-sheet origin throughout the tailward jet and the first earthward ion jet, and cold ions with small VB show up in part of the jet crossing. At the separatrix, MMS observe E×B drifting cold ions with negligible VB. The second earthward ion jet is lead by a dipolarization front, and we find four types of ion VDFs inside: Type I consists of only a single hot plasma-sheet population, Type II consists of a hot population and a cold population with a positive VB, Type III consists of a hot population and a cold population with a negative VB, and Type IV consists of a hot population with counter-streaming cold populations. Our analysis focuses on those VDFs with cold-ion beams. The occurrence rate of Type II VDFs decreases gradually from the southern to the northern sides of the neutral line. Conversely, the occurrence rate of Type III VDFs increase gradually from south to the north. The occurrence rate of Type IV peaks at the neutral line. This distribution of VDFs could be formed by the patchy cold-ion inflow, the bouncing motions within the divergent Hall electrostatic potential well, and the scattering effect due to plasma waves and the curved magnetic field lines with small curvature radii.
We adopted the same technique as used in Li et al. (2017) to compute the partial moments of the hot and cold populations of Types II, III and IV VDFs. The average number density of the cold-ion beams is approximately 13% of that of the hot ions, meaning that the cold ions are a minor population in this investigated event. All the hot and cold ions are outflow ions towards Earth. The average bulk speed of the cold-ion beams is approximately 38% larger than that of the hot plasma-sheet ions. This extra speed is predominantly along the magnetic field direction. Those cold-ion beams could come from the cold-ion diffusion region and be initially accelerated by the Hall electric field. Then, the accelerated cold-ion beams could be further accelerated by the shrinking field lines through the Fermi effect. The cold-ion beams are still one order of magnitude colder than the hot ions, while the electric field with large gradient at the separatrix and the scattering effect by the waves and highly curved magnetic field lines may thermalize those cold ions from the lobe into the ion jet.
Data Availability Statement
All data used in this work are available at the MMS Data Center (https://lasp.colorado.edu/mms/sdc/public/). Data analysis was performed using the IRFU-MATLAB package (https://github.com/irfu/irfu-matlab).
Author Contributions
WL, YL, and BT carried out the data analysis and interpretation. YL wrote the first-version article, and WL revised it. CN, JH, CW, and QZ contributed to the data interpretation and article preparation. JB led the design and operation of the MMS mission. The remaining co-authors contributed to the data analysis under the framework of an ISSI international team.
Funding
This work was supported by NNSFC Grant No. 41731070, 41974170, and 41974196, the Chinese Academy of Sciences (QYZDJSSW-JSC028, XDA15052500, XDA17010301 and XDB41000000), and the Specialized Research Fund for State Key Laboratories of China. WYL was also supported by the Youth Innovation Promotion Association (2018177), and the Young Elite Scientists Sponsorship Program by CAST and the Open Research Program of Key Laboratory of Geospace Environment CAS. CN received support from the Research Council of Norway under contract 300865.
Conflict of Interest
The authors declare that the research was conducted in the absence of any commercial or financial relationships that could be construed as a potential conflict of interest.
Publisher’s Note
All claims expressed in this article are solely those of the authors and do not necessarily represent those of their affiliated organizations, or those of the publisher, the editors, and the reviewers. Any product that may be evaluated in this article, or claim that may be made by its manufacturer, is not guaranteed or endorsed by the publisher.
Acknowledgments
We thank the MMS team and instrument principal investigators for data access and support. We acknowledge support from the International Space Science Institute (ISSI) international team Cold plasma of ionospheric origin in the Earth’s magnetosphere.
Supplementary Material
The Supplementary Material for this article can be found online at: https://www.frontiersin.org/articles/10.3389/fspas.2021.745264/full#supplementary-material
References
Alm, L., André, M., Vaivads, A., Khotyaintsev, Y. V., Torbert, R. B., Burch, J. L., et al. (2018). Magnetotail hall physics in the presence of cold ions. Geophys. Res. Lett. 45 (10941–10), 950. doi:10.1029/2018GL079857
André, M., and Cully, C. M. (2012). Low-energy ions: A previously hidden solar system particle population. Geophys. Res. Lett. 39, L03101. doi:10.1029/2011GL05024210.1029/2011gl050242
André, M., Li, K., and Eriksson, A. I. (2015). Outflow of low-energy ions and the solar cycle. J. Geophys. Res. Space Phys. 120, 1072–1085. doi:10.1002/2014JA02071410.1002/2014ja020714
André, M., Li, W., Toledo-Redondo, S., Khotyaintsev, Y. V., Vaivads, A., Graham, D. B., et al. (2016). Magnetic reconnection and modification of the hall physics due to cold ions at the magnetopause. Geophys. Res. Lett. 43, 6705–6712. doi:10.1002/2016GL069665
Angelopoulos, V., Baumjohann, W., Kennel, C. F., Coroniti, F. V., Kivelson, M. G., Pellat, R., et al. (1992). Bursty bulk flows in the inner central plasma sheet. J. Geophys. Res. 97, 4027–4039. doi:10.1029/91ja02701
Angelopoulos, V., Kennel, C. F., Coroniti, F. V., Pellat, R., Kivelson, M. G., Walker, R. J., et al. (1994). Statistical characteristics of bursty bulk flow events. J. Geophys. Res. 99, 21257–21280. doi:10.1029/94ja01263
Aunai, N., Belmont, G., and Smets, R. (2011). Proton acceleration in antiparallel collisionless magnetic reconnection: Kinetic mechanisms behind the fluid dynamics. J. Geophys. Res. 116, a–n. doi:10.1029/2011ja016688
Baumjohann, W., Paschmann, G., and Lühr, H. (1990). Characteristics of high-speed ion flows in the plasma sheet. J. Geophys. Res. 95, 3801–3809. doi:10.1029/JA095iA04p03801
Birn, J., Nakamura, R., Panov, E. V., and Hesse, M. (2011). Bursty bulk flows and dipolarization in mhd simulations of magnetotail reconnection. J. Geophys. Res. 116, a–n. doi:10.1029/2010ja016083
Birn, J., Runov, A., and Zhou, X. Z. (2017). Ion velocity distributions in dipolarization events: Distributions in the central plasma sheet. J. Geophys. Res. Space Phys. 122, 8014–8025. doi:10.1002/2017ja024230
Büchner, J., and Zelenyi, L. M. (1989). Regular and chaotic charged particle motion in magnetotaillike field reversals: 1. basic theory of trapped motion. J. Geophys. Res. 94, 11821–11842. doi:10.1029/JA094iA09p11821
Burch, J. L., Moore, T. E., Torbert, R. B., and Giles, B. L. (2016). Magnetospheric Multiscale overview and science objectives. Space Sci. Rev. 199, 5–21. doi:10.1007/s11214-015-0164-910.1007/s11214-015-0164-9
Cassak, P. A., and Shay, M. A. (2007). Scaling of asymmetric magnetic reconnection: General theory and collisional simulations. Phys. Plasmas 14, 102114. doi:10.1063/1.279563010.1063/1.2795630
Chappell, C. R., Baugher, C. R., and Horwitz, J. L. (1980). New advances in thermal plasma research. Rev. Geophys. 18, 853–861. doi:10.1029/RG018i004p00853
Cully, C. M., Donovan, E. F., Yau, A. W., and Arkos, G. G. (2003). Akebono/Suprathermal mass spectrometer observations of low-energy ion outflow: Dependence on magnetic activity and solar wind conditions. J. Geophys. Res. 108, 1093. doi:10.1029/2001JA009200
Dai, L., Wang, C., and Lavraud, B. (2021). Kinetic imprints of ion acceleration in collisionless magnetic reconnection. ApJ 919, 15. doi:10.3847/1538-4357/ac0fde10.3847/1538-4357/ac0fde
Dargent, J., Aunai, N., Lavraud, B., Toledo‐Redondo, S., and Califano, F. (2019). Signatures of cold ions in a kinetic simulation of the reconnecting magnetopause. J. Geophys. Res. Space Phys. 124, 2497–2514. doi:10.1029/2018JA026343
Dargent, J., Aunai, N., Lavraud, B., Toledo‐Redondo, S., and Califano, F. (2020). Simulation of plasmaspheric plume impact on dayside magnetic reconnection. Geophys. Res. Lett. 47, e2019GL086546. doi:10.1029/2019gl086546
Dargent, J., Aunai, N., Lavraud, B., Toledo-Redondo, S., Shay, M. A., Cassak, P. A., et al. (2017). Kinetic simulation of asymmetric magnetic reconnection with cold ions. J. Geophys. Res. Space Phys. 122, 5290–5306. doi:10.1002/2016JA023831
Divin, A., Khotyaintsev, Y. V., Vaivads, A., André, M., Toledo-Redondo, S., Markidis, S., et al. (2016). Three-scale structure of diffusion region in the presence of cold ions. J. Geophys. Res. Space Phys. 121, 12001–12013. doi:10.1002/2016JA02360610.1002/2016ja023606
Eastwood, J. P., Goldman, M. V., Hietala, H., Newman, D. L., Mistry, R., and Lapenta, G. (2015). Ion reflection and acceleration near magnetotail dipolarization fronts associated with magnetic reconnection. J. Geophys. Res. Space Phys. 120, 511–525. doi:10.1002/2014ja020516
Eastwood, J. P., Phan, T.-D., Mozer, F. S., Shay, M. A., Fujimoto, M., Retinò, A., et al. (2007). Multi-point observations of the hall electromagnetic field and secondary island formation during magnetic reconnection. J. Geophys. Res. 112, a–n. doi:10.1029/2006ja012158
Engwall, E., Eriksson, A. I., Cully, C. M., André, M., Torbert, R., and Vaith, H. (2009). Earth's ionospheric outflow dominated by hidden cold plasma. Nat. Geosci 2, 24–27. doi:10.1038/ngeo387
Ergun, R. E., Tucker, S., Westfall, J., Goodrich, K. A., Malaspina, D. M., Summers, D., et al. (2016). The axial double probe and fields signal processing for the MMS mission. Space Sci. Rev. 199, 167–188. doi:10.1007/s11214-014-0115-x
Fu, H. S., Cao, J. B., Khotyaintsev, Y. V., Sitnov, M. I., Runov, A., Fu, S. Y., et al. (2013). Dipolarization fronts as a consequence of transient reconnection: In situ evidence. Geophys. Res. Lett. 40, 6023–6027. doi:10.1002/2013GL058620
Fujimoto, M., Nakamura, M. S., Nagai, T., Mukai, T., Yamamoto, T., and Kokubun, S. (1996). New kinetic evidence for the near-earth reconnection. Geophys. Res. Lett. 23, 2533–2536. doi:10.1029/96GL02429
Gershman, D. J., Dorelli, J. C., Avanov, L. A., Gliese, U., Barrie, A., Schiff, C., et al. (2019). Systematic Uncertainties in Plasma Parameters Reported by the Fast Plasma Investigation on NASA's Magnetospheric Multiscale Mission. J. Geophys. Res. Space Phys. 124, 10345–10359. doi:10.1029/2019JA026980
Graham, D. B., Khotyaintsev, Y. V., Norgren, C., Vaivads, A., André, M., Toledo‐Redondo, S., et al. (2017). Lower hybrid waves in the ion diffusion and magnetospheric inflow regions. J. Geophys. Res. Space Phys. 122, 517–533. doi:10.1002/2016JA02357210.1002/2016ja023572
Hietala, H., Drake, J. F., Phan, T. D., Eastwood, J. P., and McFadden, J. P. (2015). Ion temperature anisotropy across a magnetotail reconnection jet. Geophys. Res. Lett. 42, 7239–7247. doi:10.1002/2015gl065168
Hoshino, M., Mukai, T., Yamamoto, T., and Kokubun, S. (1998). Ion dynamics in magnetic reconnection: Comparison between numerical simulation and geotail observations. J. Geophys. Res. 103, 4509–4530. doi:10.1029/97ja01785
Kistler, L. M., and Mouikis, C. G. (2016). The inner magnetosphere ion composition and local time distribution over a solar cycle. J. Geophys. Res. Space Phys. 121, 2009–2032. doi:10.1002/2015JA021883
Li, K., Haaland, S., Eriksson, A., André, M., Engwall, E., Wei, Y., et al. (2013). Transport of cold ions from the polar ionosphere to the plasma sheet. J. Geophys. Res. Space Phys. 118, 5467–5477. doi:10.1002/jgra.50518
Li, W. Y., André, M., Khotyaintsev, Y. V., Vaivads, A., Fuselier, S. A., Graham, D. B., et al. (2017). Cold ionospheric ions in the magnetic reconnection outflow region. J. Geophys. Res. Space Phys. 122 (10), 10194–10202. doi:10.1002/2017ja024287
Lindqvist, P.-A., Olsson, G., Torbert, R. B., King, B., Granoff, M., Rau, D., et al. (2016). The spin-plane double probe electric field instrument for MMS. Space Sci. Rev. 199, 137–165. doi:10.1007/s11214-014-0116-9
Moore, T. E., Chappell, C. R., Chandler, M. O., Craven, P. D., Giles, B. L., Pollock, C. J., et al. (1997). High-altitude observations of the polar wind. Science 277, 349–351. doi:10.1126/science.277.5324.349
Nagai, T., Fujimoto, M., Saito, Y., Machida, S., Terasawa, T., Nakamura, R., et al. (1998). Structure and dynamics of magnetic reconnection for substorm onsets with geotail observations. J. Geophys. Res. 103, 4419–4440. doi:10.1029/97ja0219010.1029/97ja02190
Nakamura, R., Baumjohann, W., Klecker, B., Bogdanova, Y., Balogh, A., Rème, H., et al. (2002). Motion of the dipolarization front during a flow burst event observed by cluster. Geophys. Res. Lett. 29, 3–1. –3–4. doi:10.1029/2002GL015763
Norgren, C., Tenfjord, P., Hesse, M., Toledo-Redondo, S., Li, W.-Y., Xu, Y., et al. (2021). On the presence and thermalization of cold ions in the exhaust of antiparallel symmetric reconnection. Front. Astron. Space Sci. 8, 149. doi:10.3389/fspas.2021.730061
Pollock, C., Moore, T., Jacques, A., Burch, J., Gliese, U., Saito, Y., et al. (2016). Fast Plasma Investigation for Magnetospheric Multiscale. Space Sci. Rev. 199, 331–406. doi:10.1007/s11214-016-0245-4
Priest, E., and Forbes, T. (2007). Magnetic reconnection. Magnetic Reconnection. Cambridge, UK: Cambridge University Press.
Runov, A., Angelopoulos, V., Artemyev, A., Birn, J., Pritchett, P. L., and Zhou, X. Z. (2017). Characteristics of ion distribution functions in dipolarizing flux bundles: Event studies. J. Geophys. Res. Space Phys. 122, 5965–5978. doi:10.1002/2017ja02401010.1002/2017ja024010
Runov, A., Angelopoulos, V., Sitnov, M. I., Sergeev, V. A., Bonnell, J., McFadden, J. P., et al. (2009). Themis observations of an earthward-propagating dipolarization front. Geophys. Res. Lett. 36. doi:10.1029/2009GL038980
Russell, C. T., Anderson, B. J., Baumjohann, W., Bromund, K. R., Dearborn, D., Fischer, D., et al. (2016). The Magnetospheric Multiscale Magnetometers. Space Sci. Rev. 199, 189–256. doi:10.1007/s11214-014-0057-310.1007/978-94-024-0861-4_8
Sauvaud, J.-A., Louarn, P., Fruit, G., Stenuit, H., Vallat, C., Dandouras, J., et al. (2004). Case studies of the dynamics of ionospheric ions in the Earth's magnetotail. J. Geophys. Res. 109. doi:10.1029/2003JA009996
Shay, M. A., Drake, J. F., Denton, R. E., and Biskamp, D. (1998). Structure of the dissipation region during collisionless magnetic reconnection. J. Geophys. Res. 103, 9165–9176. doi:10.1029/97ja03528
Tenfjord, P., Hesse, M., Norgren, C., Spinnangr, S. F., and Kolstø, H. (2019). The impact of oxygen on the reconnection rate. Geophys. Res. Lett. 46, 6195–6203. doi:10.1029/2019gl082175
Toledo‐Redondo, S., André, M., Vaivads, A., Khotyaintsev, Y. V., Lavraud, B., Graham, D. B., et al. (2016b). Cold ion heating at the dayside magnetopause during magnetic reconnection. Geophys. Res. Lett. 43, 58–66. doi:10.1002/2015GL067187
Toledo-Redondo, S., André, M., Aunai, N., Chappell, C. R., Dargent, J., Fuselier, S. A., et al. (2021). Impacts of ionospheric ions on magnetic reconnection and earth’s magnetosphere dynamics. Rev. Geophys. n/a, e2020RG000707. doi:10.1029/2020RG000707
Toledo-Redondo, S., André, M., Khotyaintsev, Y. V., Vaivads, A., Walsh, A., Li, W., et al. (2016a). Cold ion demagnetization near the x-line of magnetic reconnection. Geophys. Res. Lett. 43, 6759–6767. doi:10.1002/2016GL069877
Toledo-Redondo, S., Dargent, J., Aunai, N., Lavraud, B., André, M., Li, W., et al. (2018). Perpendicular current reduction caused by cold ions of ionospheric origin in magnetic reconnection at the magnetopause: Particle-in-cell simulations and spacecraft observations. Geophys. Res. Lett. 45, 10,033–10,042. doi:10.1029/2018GL079051
Toledo-Redondo, S., Vaivads, A., André, M., and Khotyaintsev, Y. V. (2015). Modification of the Hall physics in magnetic reconnection due to cold ions at the Earth's magnetopause. Geophys. Res. Lett. 42, 6146–6154. doi:10.1002/2015GL06512910.1002/2015gl065129
Walsh, B. M., Phan, T. D., Sibeck, D. G., and Souza, V. M. (2014). The plasmaspheric plume and magnetopause reconnection. Geophys. Res. Lett. 41, 223–228. doi:10.1002/2013GL058802
Wang, S., Kistler, L. M., Mouikis, C. G., Liu, Y., and Genestreti, K. J. (2014). Hot magnetospheric O + and cold ion behavior in magnetopause reconnection: Cluster observations. J. Geophys. Res. Space Phys. 119, 9601–9623. doi:10.1002/2014JA02040210.1002/2014ja020402
Welling, D. T., André, M., Dandouras, I., Delcourt, D., Fazakerley, A., Fontaine, D., et al. (2015). The earth: Plasma sources, losses, and transport processes. Space Sci. Rev. 192, 145–208. doi:10.1007/s11214-015-0187-2
Wygant, J. R., Cattell, C. A., Lysak, R., Song, Y., Dombeck, J., McFadden, J., et al. (2005). Cluster observations of an intense normal component of the electric field at a thin reconnecting current sheet in the tail and its role in the shock-like acceleration of the ion fluid into the separatrix region. J. Geophys. Res. 110. doi:10.1029/2004ja010708
Xu, Y., Fu, H. S., Norgren, C., Toledo‐Redondo, S., Liu, C. M., and Dong, X. C. (2019). Ionospheric cold ions detected by mms behind dipolarization fronts. Geophys. Res. Lett. 46, 7883–7892. doi:10.1029/2019gl083885
Yamada, M., Kulsrud, R., and Ji, H. (2010). Magnetic reconnection. Rev. Mod. Phys. 82, 603–664. doi:10.1103/RevModPhys.82.603
Yamauchi, M. (2019). Terrestrial ion escape and relevant circulation in space. Ann. Geophys. 37, 1197–1222. doi:10.5194/angeo-37-1197-2019
Young, D. T., Burch, J. L., Gomez, R. G., De Los Santos, A., Miller, G. P., Wilson, P., et al. (2016). Hot plasma composition analyzer for the magnetospheric multiscale mission. Space Sci. Rev. 199, 407–470. doi:10.1007/s11214-014-0119-6
Keywords: cold ions, plasma moments, acceleration, magnetic reconnection, Earth’s magnetotail
Citation: Li Y-X, Li W-Y, Tang B-B, Norgren C, He J-S, Wang C, Zong Q-G, Toledo-Redondo S, André M, Chappell C, Dargent J, Fuselier SA, Glocer A, Graham DB, Haaland S, Kistler L, Lavraud B, Moore TE, Tenfjord P, Vines SK and Burch J (2021) Quantification of Cold-Ion Beams in a Magnetic Reconnection Jet. Front. Astron. Space Sci. 8:745264. doi: 10.3389/fspas.2021.745264
Received: 21 July 2021; Accepted: 11 October 2021;
Published: 29 October 2021.
Edited by:
Olga V. Khabarova, Institute of Terrestrial Magnetism Ionosphere and Radio Wave Propagation (RAS), RussiaReviewed by:
Elena E. Grigorenko, Space Research Institute (RAS), RussiaTieyan Wang, Rutherford Appleton Laboratory, United Kingdom
Copyright © 2021 Li, Li, Tang, Norgren, He, Wang, Zong, Toledo-Redondo, André, Chappell, Dargent, Fuselier, Glocer, Graham, Haaland, Kistler, Lavraud, Moore, Tenfjord, Vines and Burch. This is an open-access article distributed under the terms of the Creative Commons Attribution License (CC BY). The use, distribution or reproduction in other forums is permitted, provided the original author(s) and the copyright owner(s) are credited and that the original publication in this journal is cited, in accordance with accepted academic practice. No use, distribution or reproduction is permitted which does not comply with these terms.
*Correspondence: Wen-Ya Li, d3lsaUBzcGFjZXdlYXRoZXIuYWMuY24=; Bin-Bin Tang, YmJ0YW5nQHNwYWNld2VhdGhlci5hYy5jbg==