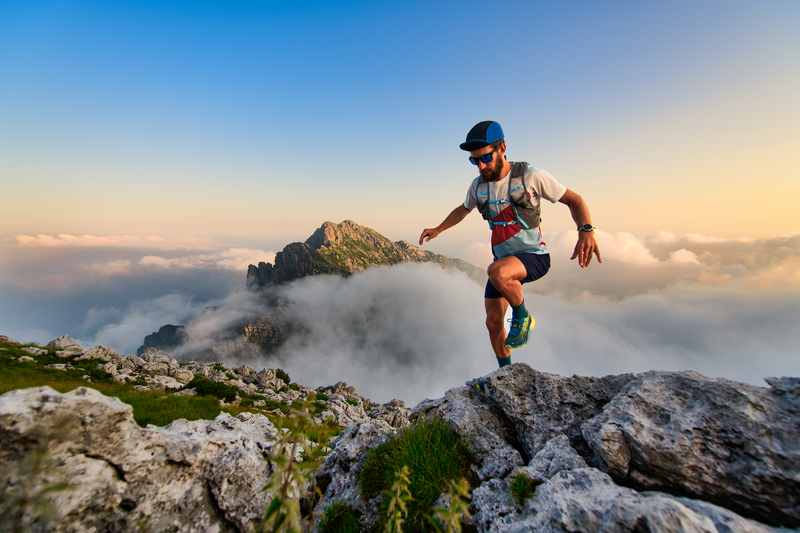
95% of researchers rate our articles as excellent or good
Learn more about the work of our research integrity team to safeguard the quality of each article we publish.
Find out more
REVIEW article
Front. Astron. Space Sci. , 15 October 2021
Sec. Astrochemistry
Volume 8 - 2021 | https://doi.org/10.3389/fspas.2021.672893
This article is part of the Research Topic Exploring the Chemical Universe View all 10 articles
The Orion molecular cloud (OMC) complex is the nearest and perhaps the best-studied giant molecular cloud complex within which low-mass and massive star formation occur. A variety of molecular species, from diatomic molecules to complex organic molecules (COMs), have been observed in the OMC regions. Different chemical species are found at different scales—from giant molecular clouds at parsec scales to cloud cores around young stellar objects at hundreds of au scales, and they act as tracers of different physical and chemical conditions of the sources. The OMC, therefore, is an ideal laboratory for studying astrochemistry over a broad spectrum of molecular cloud structures and masses. In this review, we discuss the usage of astrochemistry/molecular tracers and (sub) millimeter observations to understand the physical and chemical conditions of large-scale molecular clouds, filaments, and clumps down to cores and protostars in the OMC complex as a demonstration case.
The Orion molecular cloud (OMC) complex is a vast region consisting of giant molecular clouds (GMCs) with a variety of physical conditions. It is named after the “Orion” constellation, and the term “complex” is the collective name indicating different kinds of astronomical environments residing within. These regions are among the most vigorous star formation sites in our solar neighborhood. For more than 40 years, researchers have been actively working on the OMCs. With the advancement of astronomical facilities, our understanding of molecular clouds’ (MCs’) physical and chemical condition down to young-stellar objects (YSOs) in Orion is being improved. Using molecular line emission, we can probe the physical conditions, such as density, (kinetic) temperature, and velocity field, of the molecular gas. Emission from certain molecular species is useful in understanding the physical conditions; these species are often called “tracers.” The emission from molecular species depends on the local excitation conditions and the chemical formation mechanism in interstellar medium (ISM) environments. Though multi-wavelength observations are necessary for understanding the detail of star formations in OMC, gas-phase molecules and gas kinetic conditions are best studied using radio/(sub)millimeter facilities.
The spatial resolution of emission depends on the size of the telescope dish. With a typical single dish (of 10–30 m in diameter), we can investigate MC properties at a scale of 10,000 s astronomical units (au) at the distance of Orion. However, with interferometric facilities such as the Atacama Large (sub)millimeter Array (ALMA), one can achieve a resolution of 100-au or less for the same distance. MCs may host several cores, such as starless cores and YSOs, which are not spatially resolved by single-dish observations. Therefore, interferometric observations are necessary to understand the core properties down to YSO/protostellar scales. We recently performed survey observations toward the Orion molecular cores using ALMA at a resolution ∼ 120 au (Dutta et al., 2020; Hsu et al., 2020; Sahu et al., 2021). These sources were earlier studied using the single-dish James Clerk Maxwell Telescope (JCMT). Therefore, these consecutive observations help us understand physical and chemical evolution from large-scale core and clumps down to protostars. So, these observations enable us to understand the chemical evolution from GMCs down to protostars.
In our Milky way galactic disk, there are thousands of GMCs. The OMC or Orion complex, located at a distance of around 500 pc from the Earth in the direction of the Orion constellation, is a good example. OMC hosts the Orion-A, Orion-B, and λ-Orionis GMCs and is one of the most studied regions in astronomy. The Orion GMCs contain more than > 104 stars of age < 1–3 Myr and have older stars of 3–30 Myr in age. The older stars are no longer associated with the molecular gas; however, they are part of the Orion complex. The OB1s represent such populations of older stars. OB1s are stellar associations that consist of several dozens of hot giant stars of spectral types O and B. The Orion-OB1 has several subgroups, such as OB1a, OB1b, OB1c, and OB1d; Figure 1 marks two of these regions. The spatial extent of recent star formation activities in Orion is more than 60 degree2; however, many of the stars are still uncatalogued.
FIGURE 1. (A): Optical photograph of the Orion constellation. It shows the nebulas and major stars of “Orion the hunter.” Three OB stars of the stars of the Orion belt—Alnitak, Alnilam and Mintaka—can be seen along with the red supergiant Betelgeuse (top left). (B): The Giant molecular clouds that consist of cold molecular gas can be traced in CO emission; (adapted from Maddalena et al., 1986). Two major Orion GMC: Orion A and B marked in the figure.
Part of the OMC, the regions surrounding the hot O and B-type stars glow in visible wavelength due to radiation from stars, and star clusters are often called “Nebula.” The “Orion nebula” is a well-known and major nebula in the sky. To the south of Orion B and in the upper (north) region of Orion A, the high-density region situated within the clumpy distribution of MC is the Orion nebula, which is also designated as M42 or NGC 1972. In the Orion nebula, the radiation and winds from nebula’s cluster stars, Trapezium, create a bubble (a local cavity of number density ∼ 0.05 cm−3) of about 26 light-years (∼ 8 pc) across) and burst out one portion of the Orion-A cloud. The expanding gas near the hot stars is lit up mainly by the fluorescence of hydrogen gas and glows in visible wavelengths.
Stars and stellar populations are traditionally studied in the visible and ultraviolet (UV) wavelengths, especially the Hα emission. Molecular and atomic transitions in visible and UV are associated with electronic transitions. The required energy that drives the electronic transitions is high and typically seen only in stellar atmospheres or in extreme environments like HII regions. The bright “Orion nebula” is an HII region excited by the nearby “Trapezium” stars (θ1 OB stars). In addition to the OB clusters and high mass star-forming regions such as NGC 1977, NGC 2023, NGC 2024, NGC 2068, and NGC 2071 are part of OMC. Orion-BN/KL in M42 is an important high mass star-forming region, and violent mass outflow substantially affects the surrounding environment. High MC density, warm temperature, shocks, and grain mantle evaporation substantially change the chemistry, and this region hosts the Orion “hot core” (HC). The dynamically active outflowing gas created kinematically (i.e., based on velocity) different components, such as “expanding doughnut,” “plateau,” and “high-velocity shock”; the spatial distribution of these components are quite different (Genzel and Stutzki, 1989).
The gas content (99%) of GMCs is predominantly molecular hydrogen (H2), and about 1% dust is there. The total mass of Orion GMC is as high as 105 M⊙ (Stahler and Palla, 2004). As the typical size of dust particles is about 0.1 μm, the emission at a wavelength less than 0.1 μm is absorbed by the dust particles. Dense regions of MCs, where dust effectively blocks the emission from background stars, namely regions with high extinction, are known as “dark” clouds. Lynds 1962) (Gibb, 2008) cataloged these sources; naming starts with “L” are dark clouds. Many of the small dark/absorption nebulae are also named “Bok Globule.” Some typical dark regions are shown in Figure 2 based on optical and infrared observations of highly obscured regions. Though the cold molecular regions do not radiate either in visible or infrared radiation but can be easily detected in radio observations, molecular gas is traced from the molecular emission map in (sub)millimeter wavelength. Figure 1 shows the CO map of the entire OMC, and the presence of two prominent GMCs, Orion-A and Orion-B, can be identified from the map.
FIGURE 2. (A): The combined map of OMCs based on IRAS 25.60, 100 μm observations and CO map from Dame et al. (2001). The labels can be compared with CO map of Maddalena et al. (1986) as shown in Figure 1. (B): Some prominent Orion dark clouds are marked in the figure (adpted from Stahler and Palla, 2004). The dark cloud named after “L” (e.g., L1630) are based on Lynds cloud catalogue. The cloud named after “NGC” and including the shaded regions are reflection nebulae, i.e., dusty clouds that scatters in optical wavelength.
Typical sizes of GMCs are around 30–200 pc, so are the Orion A and B GMCs, which are elongated and clumpy structures are present. The GMCs can be divided into further sub-regions or sub-structures, typically cataloged based on the size, mass, and hierarchy of shapes (see, e.g., Stahler and Palla, 2004). The substructures in GMCs/MCs are nested in a hierarchical pattern, e.g., the clumps are nested inside GMCs and cores within clumps. A typical representation of such hierarchy structure is shown in Figure 3. GMCs structures are highly irregular and not very well defined; other structures like filaments and bubbles are also present in GMCs. A large filamentary structure of 10 s of pc in size is seen along the north-south direction in the Orion-A GMC (Bally et al., 1987; Nagahama et al., 1998) and is commonly known as the integral-shaped filament. Herschel PACS 70/160 μ`m and SPIRE 250/350/500 μm observations of Orion dark cloud L 1641N identified filaments and dense cores. It was seen that most of the prestellar cores are located along the filaments. Also, surveys of Hα emission are often used to trace the low-mass star formation, and many low-mass stars are found to be distributed in the Orion ridge of MCs. High-velocity outflows are often created due to star formation activities, interact with the surrounding gas and dust, and result in luminous Herbig–Haro (HH) objects. OMCs host many such HH objects, e.g., HH 212 in the L1630 cloud.
FIGURE 3. The cartoon depicts how clumps to cores with hierarchical structures were observed in the ALMASOP survey. Red contours are cores of a PGCC, which were observed with JCMT (Yi et al., 2018) ∼ 6,000 au resolutions. The cores were observed in detail with ALMA and revealed embedded protostars with multiplet and dense prestellar cores (Dutta et al., 2020; Sahu et al., 2021).
In summary, the OMC hosts many stellar objects, stellar clusters, HH objects, HCs, HII regions, photo-dissociation regions, molecular clumps to star-forming cores, and diffuse to dense gas. The major focus of this article is to discuss the Orion complex from the viewpoint of molecular astronomy. Additionally, molecular emission and chemistry are interconnected, so, overall astrochemistry is a good tool to study the Orion GMC environment and its components. Based on this idea, we organize the article to discuss the “anatomy of Orion.” However, it is to be noted that this article is not an exhaustive summary of all the observational results toward Orion.
As the Orion complex is huge (10 s of pc), so recovering the full extent of the cloud complex is extremely rare from a single observational project. Also, visible or infrared observations cannot detect the full extent of the MC as the cold MCs ( ∼ 10 −−20 K) do not emit in infrared or visible wavelengths. To detect cold dust through their thermal continuum emission or molecules through their spectral line emission/absorption, we predominantly rely on (sub)millimeter observations. Single dish observations in this wavelength are beneficial to map MCs in parsec scales. However, the resolution (∼ wavelength/dish diameter) of single-dish observations is limited due to dish size of few meters; higher resolutions can be achieved using interferometric observations. Therefore, single dish studies are limited to large structures such as clumps, filaments, and unresolved cores. In Section 2.1, we mainly discuss the large-scale structures, clumps, and filaments of the OMC, and in the subsequent sections, we discuss cores properties.
Possibly the most extensive CO (1–0) line survey performed by Maddalena et al. (1986) using a 1.2 m single-dish telescope (beam size 8. ′7 at 115 GHz), which shows the entire molecular gas structure of Orion A and B traced by the CO emission. The molecular emission associated with Orion A and B extends over 29 and 19 deg2 (1 deg ∼ 7 pc) and are connected by low-level emission, signifying their physical connection. From the large-scale map of CO and its isotopes, Nishimura et al. (2015) find that stars from along the dense filaments and the formation efficiency are correlated with gas density, and found that star formation rate in Orion A is higher than Orion B. Both Orions A and B have high CO temperatures and a ridge of emission on the western side due to recent star formation activities and OB stellar clusters. Star-forming regions in those clouds are found to be coherent, i.e., low-turbulence environment, based on the CO line width and velocity profile. In λ-Orinis, a ring of clouds surrounding and expanding away from the star λ-Ori was traced by the CO emission. The CO emission also traces the dark clouds B30, B35, and B223; in these regions, the temperatures tend to be higher due to stellar heating or compression resulting from nearby stars. Ungerechts et al. (1997) surveyed a region of Orion-A, 6′ north and south of BN-KL with FCRAO 14 m telescope with a coarse resolution of 50′′ at 3 mm Figure 4. They surveyed the region using different molecular transitions of 20 different species including CO, 13CO, C18O, CS, C34S, HCO+, H13CO+, N2H+,CN, C2H, SO, 34SO, HCN, H13CH, HNC, HN13C, CH3OH, C3H2, HC3N, and CH3CCH. This list of molecules constitutes a good sample to understand the physical properties and structures in GMC environments. For example, Ungerechts et al. (1997) from their analysis found that CS, C34S, and CH3CCH are best at delineating the structure of dense molecular ridge. Though CH3CCH emission was weak due to its low abundance compared to CS, both of them arise from the same volume of gas as estimated from line velocities and widths. Also, kinematical signatures of molecules C18O, C34S, H13CO+, CN, SO, and C3H2 show that they are predominantly associated with the molecular bar. Molecules like HCN, CH3OH, HC3N, and SO are found to be enhanced toward the Orion KN-BL region e.g., Blake et al. (1987), Sutton et al. (1995) and a subset of these molecules are found to be enhanced in other star-forming regions too. It seems that these molecules trace star-forming regions and energetic activities (such as outflows) within them.
FIGURE 4. Maps from coarse resolution (50″ ∼ 20,000 au) observations of the OMC-1 region with the FCRAO telescope by Ungerechts et al. (1997). The maps show different emission features of the OMC-1 region including the ridge, bar, outflow, and radical emission region [maps are adapted from Ungerechts et al. (1997)]. High-resolution observations further revealed the components described in Figure 6.
CO and CS survey maps show many filaments and shells, and most prominently an Integral Shaped Filament (ISF) extending over ∼ 1° region (e.g., Bally et al., 1987; Dutrey et al., 1991; Heyer et al., 1992). The ridge includes two prominent molecular emission peaks: OMC-1 and OMC-2. The OMC-1, which lies behind the Orion Nebula (M42) and the Trapezium stars, contains massive star-forming regions around Orion-BN and KL regions. The ISF region contains a variety of sources, including photo-dissociation regions (PDRs) and shocks. Johnstone et al. (2003) used JCMT molecular line observations of several molecules to trace the physical properties of these regions. Eight different molecules from carbon-, oxygen-, nitrogen-, and sulfur-bearing species were chosen to understand the chemical differentiation.
The basic chemistry of MCs here is that hydrogen mainly exists in molecular form, H2 which forms via grain/ice phase recombination of H atoms. In the MCs, the typical density of H2 is in the range of 102–107 cm−3. CO easily forms in MC via ion-neutral reactions and is the most abundant molecule after H2. Rotational transitions of CO have a low Einstein A coefficient and results in low critical densities. Therefore, the rotational transitions of CO are used to trace a wide range of densities in the GMCs from its lower density periphery to dense molecular ridge. In the dense regions of an MC where CO is optically thick, the less abundant isotopologous, e.g., C17O are used to study the gas properties. Formaldehyde (H2CO) is a slightly asymmetric rotor and provides temperature and density diagnostics for a wide range of gas conditions. On the other hand, methanol can trace hot conditions of the cores where ice phase molecules thermally desorb to the gas phase. HCN is one of the most abundant nitrogen molecules, and its abundance increases with temperature; therefore, it is a useful evolutionary tracer. Also, HCN/HNC ratio depends on the density and kinetic temperature of the gas, so it acts well as a physical diagnostic.
The dense part of the molecular cloud where the gas density is higher than 104 is called “cores,” and dense cores are embedded within complex structures like clumps and filaments. CO freeze-out or depletes onto dust grain forming ice mantles in the cold denser part of the molecular cores and no longer acts as a good density tracer. Therefore, rotational transitions from species such as NH3, CS, CCS, H13CO+, and N2H+ are used to trace a variety of density structures due to their different values of critical densities (104 or higher). In addition to the line tracers, thermal emission from dust in (sub)millimeter wavelength, popularly known as “dust continuum,” is also a good tracer of dense regions. Dust continuum detected using (sub)millimeter emission detects cold objects, including core and clumps. Planck catalog of Galactic Cold Clumps (PGCCs) are such objects that were built using the observations of three Planck bands (857, 454, and 353 GHz) in combination with the IRAS data at 3 THz. The median temperature of the PGCCs lies between 13 and 14.5 K. Dense filaments and cold clumps are the ideal sites for the formation of stars. The cores embedded within Orion PGCCs are further discussed in Section 2.3.
Based on their evolutionary sequence, high mass star-forming regions (HMSFRs) qualitatively can be separated into four stages. At the first stage, quiescent infrared dark clouds (IRDCs) are formed; due to their inherent cold temperature, they are mainly detected at (sub)millimeter wavelengths. At the second stage, high mass protostellar objects (HMPOs) form, hosting an accreting protostar (massive >8 M⊙) that can be detected at (far) infrared wavelengths. The temperature rises and a much warmed hot molecular core (HMC) forms in the follow-up phase. The HMC phase is defined from a chemical point of view; in this phase, many complex organic molecules (COMs; six atom or higher) are detected. Finally, the energetic UV radiation from the embedded (proto) star ionizes the surrounding gas medium, creating an HII region. Orion-KL is a well-studied high-mass star-forming region and one of the most studied objects in OMC. As a template of the physical and chemical structure of a typical high mass region, we describe the Orion-KL regions.
The Orion-KL region is an exemplary high mass forming region located in the OMC-1 cloud. It was identified by Kleinmann and Low (1967) as the brightest infrared emitting region in the OMC, and is located close to the Trapezium cluster. The young massive stars in the Orion-KL are embedded inside dense gas and dust cocoons and remain undetected in the visible wavelength. The Becklin-Neugebauer object (BN) (Becklin and Neugebauer 1967) was the brightest source at 2 μm. At higher wavelength bright sources like IRc 2 and several other sources were detected. Some infrared sources are found to be manifestations of complex structures resulting from the surrounding molecular clouds. Radio sources like I and n are also present in the Orion-KL region, and the sources BN, I, n constitute three prominent runaway objects.
Based on single dish molecular line observations of the Orion-KL region, Blake et al. (1987) identified three components corresponding to distinct spatial/velocity signatures. These three components are named as the HC, compact ridge, and plateau. The molecular species that trace these components are labeled in Table 1. Simple molecules like CO and CN trace a range of density of molecular clouds. In the dense molecular clouds, these molecules are easily formed. On the other hand, the larger molecules such as NH3, CH3CCH, and HC3N also are produced in the gas phase of the cold dense regions, and are typically used as high-density tracers due to their higher critical densities. Meanwhile, these molecules, having symmetric structures, therefore act as good tracers of gas kinetic temperatures in dense cloud regions.
TABLE 1. Different components of the Orion-KL region as identified by Blake et al. (1987); the molecular line properties, kinetic temperature, density, source size, and tracer species are noted in the table.
High mass cores with gas-phase COMs and temperatures higher than >100 K are often termed as hot molecular cores (HMC). COMs are believed to form via surface recombinations of atoms and radicals on grains and then come to the gas phase mainly via thermal desorption; subsequently, they can be detected from line/spectroscopic observations. The “plateau” is created due to high-velocity outflow activity around the Orion-KL HMC. SiO is a good tracer of outflow activities. The main reason is that silicon is mainly trapped in dust grain (silicate) and ice-mantles. When heavy impact due to outflow shatter dust grains via gas-grain collision and sputtering, silicon (Si) comes to the gas phase. In the gas phase, “Si” reacts with “O” and form SiO. Additionally, SiO emission suffers minimal contamination from the infalling envelope and well traces jet emission. Sulfur-related species are also produced under high-temperature conditions and often trace well energetic environments like outflows.
One remarkable feature in the Orion-KL region is that in the central region an explosive event occurred about 550 years ago (Pagani et al., 2019). Figure 5 shows the three runaway objects Orion-K, l, and n, which are affected by the explosive effects along with different molecular emission regions. Based on ALMA observations, Zapata et al. (2011) and Orozco-Aguilera et al. (2017) proposed that the Orion HC is externally heated due to the effect of the explosion. In addition, Blake et al. (1987), Wang et al. (2011), and Favre et al. (2011) suggested that the compact regions are also affected by the external heating, though the heating source may not be the explosion but outflows from the source I hitting the compact ridge (Liu et al., 2002). The explosion affects the chemistry of the Orion-KL region and its components. High excitation emission lines are primarily associated with the explosion center and the distribution of oxygen-rich COMs and CN-rich COMs are different. For example, ethylene glycol peak is found to be associated with hollow spheres of material, which originate from the impact of “bullet” originating from the explosion center (Favre et al., 2017; Wright and Plambeck, 2017). On the other side, the compact ridge is not affected by the explosion as studied from the line-width profiles. Overall, this Orion-KL region is a unique source and the 550-year-old explosion is comparable to the “time of flight” experiment Pagani et al. (2019, and references therein) and helpful to understand how chemistry evolves in the cloud. Numerous molecules and their emission profiles can be studied both spatially and in velocity, which is particularly helpful in discerning the temporal evolution of molecules.
FIGURE 5. Adapted from Pagani et al. (2017), the figure shows different regions of the Orion-KL observed at 1.3 mm with ALMA. HC and CR represent hot core and compact ridge, MFxx (Favre et al. (2011) represents regions identified from methyl formate emission, IRCxx represents sources detected in infrared (Rieke et al., 1973). EGP represents the ethylene glycol peak as reported by (Brouillet et al., 2015). Sources have different naming conventions, such as mmxx and Cxx based on the observation by Feng et al. (2015) and Friedel and Widicus Weaver (2011) and references mentioned therein. The BN, I, and n, marked by yellow crosses, represent the three runaway sources which are moving away from the center of the explosion. Detailed observations with ALMA is performed by Pagani et al. in series of papers.
The Orion “hot core” is very particularly interesting for studying COMs. Many surveys have been conducted by single-dish telescopes, e.g., the earliest works by Sutton et al. (1985), Blake et al. (1986) to recent observations by Tercero et al. (2010), Tercero et al. (2011), Crockett et al. (2014), Rizzo et al. (2017) to detect new COMs. The range of molecules from simple to COMs including HCOOH (formic acid), CH3OH (methanol), HCOOCH3 (methyl formate), CH3OCH3 (dimethyl ether), CH3CH2OH (ethanol), CH3COCH3 (acetone), CH3CHO (acetaldehyde), (CH2OH)2 (ethylene glycol), and CH2OHCHO (glycolaldehyde) were detected. The high sensitivity of modern single dish spectrometers, though helpful for the spectral survey and COMs detection, has reached the confusion limit toward Orion-KL. Single dish observations, being poor in spatial resolution, include emission from different sources associated with different velocities, results in increasing overlap of lines and leads to spectral confusion. As an example in Table 2, we have mentioned a list of molecules that are detected from a few single dish surveys. Though the COMs are coming from Orion-KL, detailed physical and chemical conditions associated with the COMs could not be studied. Therefore, interferometric arrays with high angular resolutions are used to separate the velocity components and diminishing confusion (e.g., Favre et al., 2011; Peng et al., 2012; Brouillet et al., 2013; Feng et al., 2015; Tercero et al., 2015; Peng et al., 2017). It should be remembered that the list of COMs that are mentioned are not exhaustive. Additionally, ALMA observations make it possible to detect notable complex species like gGg′ isomer of ethylene glycol isomer (
The extreme ultraviolet photons (>13.6 eV, i.e., the ionization potential of hydrogen) from the massive star cluster Trapezium fully ionize the gas surrounding it and creates the so-called HII regions where hydrogen is in the form of H+. High energetic photoelectrons can collisionally heat the gas as high as ∼ 104 K, and the electron density of this region ranges from 1–105 cm−3 (Hollenbach and Tielens, 1999). The hydrogen number density of this region is ∼ 1 cm−3 or less, whereas in the dense molecular cloud the density is > 104 cm−3. In the distant region from the star, H + ion starts recombining with electrons and forms hydrogen atoms (known as HI regions). The size of the HII region near a star depends on the energy distribution and electron number density for the recombination of ionized hydrogen. The radius (the distance from the star) at which the recombination and ionization maintain equilibrium is called Strömgren radius, beyond which hydrogen remains mainly in the atomic form (HI region). The properties of the spectrum help us to understand these regions. Based on quantum mechanical probabilities, the dominant photon that escapes the HII region results from n = 3-2 transitions of electron and denoted as Hα. In the HI regions, atomic hydrogen (H0) shields most of the extreme ultraviolet emission (EUV) 100 eV > hν > 13.6 eV, however far-ultraviolet emission (FUV <13.6 eV) can penetrate deep inside the cloud. These FUV photons ionize other elements such as C, Mg, S, and Fe (with their ionization potentials below 13.6 eV) and other molecules become photo-dissociated. The regions near the OB stars dominated by FUV emission of the stars are called PDRs (see, e.g., Hollenbach & Tielens 1997). The PDR located at ∼ 2 arcmin southwest to the Trapezium cluster in Orion-A is popularly known as the “Orion Bar.”
A significant molecular column density builds up when FUV emission is heavily attenuated by dust. For the typical condition of molecular clouds, extinction must exceed ∼ 0.1 mag to observe a significant amount of molecular hydrogen (H2). As the CO abundance is much less than H2, CO can only be observed in higher density clouds where Av is much higher. van Dishoeck and Black (1988) showed that a GMC has a layer of C+ and C0 for a hydrogen column density (corresponding to total hydrogen nH = 2nH2 + nH) column density: NH = 1.4 × 1021 cm−2. Extinction increases with the increased hydrogen number density; the relation between extinction and hydrogen often estimated by
So, interstellar clouds with mean extinction significantly higher than 1.4 mag (0.7 corresponds to NH = 1.4 × 1021 cm−2, therefore extinction through the whole cloud = 2 × 0.7) are expected to have a thin layer of H0 and a thick layer of H2, C0, and C+, and the interior regions would be fully molecular.
The λ−Orionis region (Figure 6B) is a good example, understanding the evolution of the HII region to PDR and dense clouds. This region is affected by a supernova explosion that occurred about 1 Myr ago, which may lead to the formation of a large HII region. The figure shows a three-color composite map including emission in IR, millimeter, and Hα emission. Hα traces the diffuse and warm HII regions. The 353 GHz (0.84 mm) dust emission as detected using Planck telescope traces the dust continuum emission from dense and cold molecular clumps and cores. Some of these clumps and cores also host protostars (YSOs), emission from infrared due to star formation activities are traced by IRAS 100 μm continuum emission. We discuss the Orion Bar PDR region and chemistry in dense molecular clumps and cores of OMCs in the subsequent section.
FIGURE 6. (A): Left panel: Overlay of the HCO+ J = 3–2 (in red, traces extended OMC), SII (in green, traces hot ionized gas surrounding the Trapezium stars), OI (in blue, traces interface between the ionized and the neutral gas) around Orion; BN/KL, star-forming region. Right panel: A close-up, ALMA HCO+ J = 4–3 emission (red) of the Orion molecular bar region; the black region is the atomic layer. Image taken from Goicoechea et al. (2016). (B): Three-color composite image taken from Yi et al., 2018 (red: Planck 353 GHz; green: IRAS 100 μm; blue: Hα) of the λ Orionis cloud. The white contours show the dust continuum of molecular clumps and cores detected in Planck 857 GHz emission. The yellow star indicates the position of the “λ-Ori” OB binary.
Due to the high energetic UV emission from the Trapezium cluster an HII region, ionized hydrogen is created near the Orion nebula. Orion Bar is an interface region between the HII region and the Orion A, and it is a good example of a strongly illuminated photodissociation region (see Figure 6B). Major chemical reactions in the PDR regions are related to the reactions between atomic ions, electrons, H, and H2. As an example, hydride formation starts via the reaction between C+ and H2. At first, CH2+ form via radiative association reaction between C+ and H2: C+ + H2 = CH2+ + hν. Now, CH2+ can undergo number of reactions to form ionic species like CH3+ and CH5+. Since the radiative association reactions are slow, the dissociation of the ionic species (e.g., CH3+) produces neutral hydrides CH, CH2. The reaction pathways of hydrides also allow formation of simple molecules like CO. Ionic oxygen (O+) produce in reaction with O and H+, and following O+, oxygenated species OH+, H2O+, H3O+, OH, etc., are produced. Like oxygen, nitrogen remains predominantly in the atomic form in diffuse PDR and a series of neutral-neutral reactions with hydrides and other molecular species lead to species such as CN and HCN.
A line survey of the Orion Bar edge was performed by Cuadrado et al. (2015), Cuadrado et al. (2016), Cuadrado et al. (2017). The PDR regions host chemical features of reactive ions such as SH+,CH+, CO+, and HOC+ (Fuente et al., 2003; Goicoechea et al., 2017), and hydrocarbon ions, e.g., C3H+ (Pety et al., 2012; Cuadrado et al., 2015). Jansen et al. (1995) found that radicals such as CN and C2H are abundant in the low extinction region of PDR. Due to enhanced abundance of the radical species, it was named as the “radical” region. Using ALMA-ACA Goicoechea et al. (2017) observed reactive ions SH+ and HOC+ in high angular resolution (5″ × 3″). The observed emission traces a narrow layer ∼ 800–4,000 au, and possibly follows the vibrationally excited H2∗. Both ions close trace the H/H2 transition region of Orion bar (Av < 1 mag), and in this region C+, S+, and H2∗ coexist. Complex organic molecules do not form efficiently in PDR regions. Interestingly, a number of COMs—trans-HCOOH, CH2CO, CH3CN, CH3OH, CH3CHO, and CH3CCH—are detected at the edge of the Horsehead nebula (dark nebular near the east star of Orion belt) (Guzmán et al., 2014). The COMs mainly originate from a cold condensation region, shielded from the UV field, due to ice-mantle photodesorption, and gas-phase reactions in UV-illuminated neutral gas. On the other side, in a lower density translucent clouds only H2CO is detected (Liszt et al., 2006), therefore, the detection of larger molecular species suggests that COMs may not form efficiently in strongly irradiated regions. Cuadrado et al. (2017) from their spectral survey toward the Orion bar edge found molecules including H2CO,CH3OH, HCO, H2CCO, CH3CHO, H2CS, HCOOH, CH3CN, CH2NH, HNCO, and H13CO in a decreasing order of abundance. Other than the ever prevailing ionic species/tracers in PDR, the COMs represent at most the possible complex chemistry in regions close to PDR environments.
In addition to the chemical complexity and molecular line tracers, PDRs emit IR radiation, which hints at star formation activities in GMCs. H2 rovibrational lines, CII 158μm, OI 63μm fine-structure lines, etc., and heated grain/dust after absorbing FUV photon from nearby stars re-emitted in mid-IR and far-IR bands (see Figure 6A). Therefore, using molecular lines typical of PDRs, IR dust emission together helpful for studying chemical evolution as well as star formation activities.
It is accepted that stars form by the gravitational collapse of cold, dense, molecular cores. Molecular cores are not distributed randomly in the GMCs; instead, they are aligned along the gas filaments. Figure 7 shows positions of a few PGCCs along the Orion GMCs, which are the site of low mass star formations. Cores are embedded inside the clumps. Not all molecular cores necessarily form protostars. Only the prestellar cores, which are gravitationally bound and collapsing, can form protostars. Early stages of protostars are (e.g., Class 0, I) constitute of a young disk, envelope and outflow-jet system. A Solar-like system may form in later evolutionary stages (e.g., Class II, III) of low-mass cores. To study different components of a protostar, a resolution of 100 au (0.′′25) or higher is necessary, which can only be achieved using interferometers like ALMA. HH212 is a low-mass protostar at the Class 0/I stage with disk, envelope, and outflow components. HH 212 is one of the most well-studied protostars using high angular resolution interferometric observations. This section describes the studies of the Orion cores and chemistry in a typical protostar, HH 212.
FIGURE 7. Three-color composite image (red: Planck 353 GHz; green: IRAS 100 μm; blue: Hα) similar to Figure 4 (right panel) but for Orion-A and -B GMCs (Yi et al., 2018). PGCCs that marked in red were analyzed by Yi et al. (2018), and 72 PGCCs in Orion-A, -B, and λ Orionis were further observed in detail with ALMA.
The red circles and orange triangles are the location of some 50 PGCCs with N(H2) > 5 × 1020 cm−2. This type of clumps and cores are further observed at high resolution with ALMA (see Section 4).
Ikeda et al. (2009) carried out a core survey of 1 deg2 region in Orion B using H13CO+ (J = 1–0) transition with the NRO-45m single dish at a resolution of ∼ 27.′′, which was better than 1. ′8 and of 3. ′8 of previous studies of the cloud in CS (2–1; Lada et al., 1991a) and H13CO+ (1–0; Aoyama et al., 2001). The survey detected low mass core (of about 10 M⊙ or less) with an average size of 0.1 pc (∼ 20,000 au). A comparison with the 0.85 mm dust continuum showed that the H13CO+ cores are evolved cores and possibly star-formation is going on. In a similar type of survey, using H13CO+ line observations, Ikeda et al. (2007) detected 236 cores in Orion-A. HCO+ generally depletes inside extremely dense cores where no hot central region due to star formation is present. Also, the high critical density of H13CO+ (1–0) > 104–105cm−3 is helpful for detecting dense cores. These kinds of molecular line studies along the dense filaments of GMCs are an important tool to understand core properties and low mass star formations along the filaments.
Many PGCCs were identified along the filaments of Orion clouds (see Figure 5) using various single dish telescopes (e.g., JCMT, IRAM, PMO 14 m, APEX, Mopra Effelsberg, CSO, SMA, TRAO), Tatematsu et al. (2014) studied the chemical properties of Orion PGCCs and tried understanding the core evolution using chemical properties of the cores. CCS and HC3N tend to be abundant in early starless cores, while nitrogen-related molecules like NH3, NH2+, and cyclic molecules like c–C3H2 trace star-forming cores. As the density increases during the core-collapse and due to the inherent cold temperature of the dense cores (∼10–20 K), CO starts depleting, and the abundance of CO-related molecules (e.g., HCO+) slowly decreases from the gas phase. Therefore, CO and related molecules no longer act as a good tracer of high-density cores. However, nitrogen-related species are less affected by depletion, and molecules like NH3 and N2H+ trace denser regions of cores. Tatematsu et al. (2014) finds that N(N2H+/)N(CCS) is a good evolutionary indicator of molecular cores. For starless cores they found N(N2H+/)N(CCS)) < 2–3 and in star-forming cores it is >2–3; the molecular ratios are different because in evolved cores, CCS decreases and N2H+ increases. CCS emission appears different from N2H+ emission, and in starless cores it is found to surround the N2H+ emission (Lai and Crutcher, 2000; Aikawa et al., 2001), additionally CCS emission may appears from clumpy structures too. Based on the molecular tracers, Tatematsu et al. (2017) defined a chemical evolution factor (CEF), and the value of CEFs ∼ -100–0 and 0–100 were estimated, respectively, for starless and star-forming cores.
The deuterium fractionation (D/H ratio) of chemical species are also found to trace the evolution of molecular cores. Deuteration mainly happens in the cold starless phase, and the deuterium fractionation increases with the evolution of starless cores; however, as the gas becomes warm during the formation of protostars, the fractionation starts decreasing. Though these are not strict forward rules to understand the core evolution based on deuterium fractionation, one must consider various factors like the temperature, density, chemical properties of related molecules. For example, N (DNC)/N(HNC) decreases with core evolution due to an increase in kinetic temperature and partly due to a larger reaction time scale (104 years). N ((N2D+)/N(N2H+) ratio increases with core evolution, and decreases during the evolved warm-up (>20 K) phase; as the reaction time scale (∼ 100 days) is shorter during the collapse phase, N ((N2D+)/N(N2H+) traces the current temperature more directly Sakai et al. (2012). In another observation, Hirota and Yamamoto (2006) found that N (DNC)/N(HN13C) increases with core evolution within a range of 0.66–3 for their sampled cores.
When observed at a high angular resolution, a protostar system has more specific chemistries related to protostar structure. For example, in class 0 sources, H2CO and CO ratio are of the order of 10–5, it increases by a factor 100 or so, in the inner region of warm envelopes (Johnstone et al., 2003). H2CO can form in icy mantle because of surface hydrogenation of CO, so the jump in H2CO abundances indicates the ice evaporation in the inner warmer region of the envelope. The observation of CH3OH, in association with H2CO, also suggests the evaporation of CH3OH from ice-grain surfaces. CS traces more energetic regions and implies that warm or evolved sources provide more heating to the outer envelope. The paragraph below describes an exemplary protostar, HH 212, observed using interferometry (e.g., ALMA) at 100 au or higher resolution.
HH 212 is a star-forming system located in the L1630 cloud of Orion-B. The extended (0.47 pc) bipolar jet from this object was first discovered by Zinnecker et al. (1998) from H2 rotational-vibrational lines. The narrow jet of partially ionized gas from the young central star collides with nearby gas and dust and creates patches of nebulosity, which are typical for HH objects.
HH 212 is a class 0 YSO. Typically, at this early stage, YSOs are composed of four major components: 1) an infalling envelope, 2) a circumstellar disk, 3) a jet-outflow system, and 4) the central stellar object. During the initial gravitational collapse, the envelope is massive enough and cold (about 10 K) and obscures emission at UV, optical, and near-infrared (NIR) wavelengths, so the cold envelope only can be observed in (sub)millimeter continuum and molecular lines. With time the envelope gets removed, and the central region (around 100 au in scale) becomes hot and hosts a disk-jet system. The temperatures of the envelope range from 10 K in the outer regions to 100 K at the inner (100 au) regions, and the density varies from 104 cm−3–108 cm−3 in these regions. The inner dense and hot region, which is enriched with COMs, is called a hot-corino (Codella et al., 2016).
The envelope of HH 212 is well traced by 17CO emission (Lee et al., 2017), and CO also traces the wide-scale outflow and cavity regions. The inner region of the envelope can be viewed in the optically thin C17O emission. Due to the lower abundances of the CO isotopologues, the C17O emission allows one to look deep into the envelope and study the innermost infalling regions with higher rotational velocities. HH 212 hosts an infalling envelope and rotating keplerian disk as inferred from the HCO+ and C17O observations (Lee et al., 2014). The bipolar outflow from the source is traced by SiO (8–7) (Codella et al., 2007) line emission, and in general, it is a good tracer of shocks.
Due to the outflow activities, materials blow up along with the outflow and create cavities due to emptied gas and dust. Such a cavity wall in HH 212 is traced by C34S (7–6) emission (Tabone et al., 2017). The critical density of C34S (7–6) emission is quite high (around 109 cm−3), so the dense gas in the cavity wall is traced by C34S. Another molecule, HDCO, is found to trace a similar region (Sahu et al., 2018). HDCO is mainly produced in the grain phase during the cold starless phase of the molecular cloud. So, the HDCO tracing cavity wall suggests that shocked material may be present in the cavity wall, and HDCO injected in the gas phase due to sputtering during outflow activities.
The transitional region between the innermost infalling and rotating envelope and the keplerian disk is called the centrifugal barrier. In the centrifugal barrier, a ring of shock material is expected due to the excess energy and angular momentum of infalling material dispersed before moving into the keplerian disk. However, the low velocity of accretion shock in the centrifugal barrier cannot destroy the grain’s refractory material, but some chemical changes happen possibly due to sudden heat-up. It was found that c–C3H2 and SO trace the centrifugal barrier in HH 212 system.
Though researchers have been studying OMC for several decades, it is still an exciting region from both star formation and astrochemistry aspects. Here, we mention a few aspects on which new studies are performed and the future scope for high-resolution studies.
Filaments and star formation: Filaments are an important structural element of star-forming regions in Orion. Some observational results are already mentioned in Section 2.1. Far-infrared and (sub)millimeter observations made with Herschel further reveal that, in general, interstellar filaments and star formation in them are correlated. Therefore, there have been renewed interests in the science community to study the filaments. As an example, Orkisz et al. surveyed 1.9 deg2 regions in Orion-B using C18O (1–0) at a resolution of 23.5″ with the IRAM-30m single-dish telescope. The density and velocity structure was studied from the CO emission. Based on the results, the filaments in Orion-B are found to be stable against gravitational collapse because of the lukewarm temperature, which is one of the main reasons for the low star formation rate in Orion-B.
Effect of environments: The typical chemical properties in the Orion cores are described in earlier sections. It is, however, important to know the role of environments to the cores located in different regions of Orion. New survey observations are being performed to understand the effect of environments within the Orion GMCs. Recently, Yi et al. (2021) surveyed the Orion cores located in Orion-A (39 cores), B (26 cores), and λ Orionis (15 cores). Molecular tracers like N2H+, HCO+, H13CO+, C2H, HCN, and H2CO were included in the survey to understand the effect of stellar feedback in the OMC. The cores in λ Orionis are found to be less dense; also the deuterium fractionation estimation indicates the λ Orionis cores are less evolved. Combining the analysis of multiple lines, overall, it was found that λ-Orionis cores are mostly affected by nearby photodissociation and heating from nearby HII regions, and possibly stay in the starless stage for a longer timescale. These kinds of studies are necessary to understand star formation rates in the Orion GMCs and the effect of stellar feedback.
The areas mentioned above are typical new trends based on recent single-dish observations. Single dish studies were also performed to study starless/prestellar cores, as discussed in Section 2.1. However, it was unclear how the cores collapse to form protostars and what happens inside them. To answer these questions, one needs to survey many Orion cores at different stages of their evolutionary states using high-resolution observations. A recent observational study, ALMASOP, fills in the gap; details are described in the next section. In the ALMASOP observation, the resolution was ∼ 140 au, sufficient enough identifying the embedded protostars within dense cores. However, the resolution was not enough for studying the protostellar disks and subsequent evolution to the protoplanetary disks. Therefore, one of the primary goals in the future will be to uncover the disks in OMC and to study the kinematical properties, temperature structures, and chemistry with high-resolution molecular line observations.
Typically molecular core sizes are ∼ 10000s au (∼ 0.05 pc). Many embedded cores are found within Orion-PGCCs. Yi et al. (2018) observed PGCC sources using JCMT (SCUBA-2) 0.84 mm observations. Figures 4, 5 show the locations of cores, which were surveyed at a resolution of about ∼ 6,000 au (14″) (Yi et al., 2018). In this survey, 119 dense cores with masses in the range of 0.2–14 M⊙ with an average radius of 0.05 pc were revealed. These Orion cores were further studied in molecule lines with the NRO-45m telescope (Kim et al., 2020). The spectral line observations show that nearly half of the JCMT objects exhibit strong emission from N2D+, DCO+, and DNC, which are tracers of cold, and dense molecular gas and the core may be chemically evolved (Kim et al., 2020; Tatematsu et al., 2020).
Chemically evolved cores are highly probable to host gravitationally bound prestellar cores and embedded faint protostars, which are not detected in infrared emission. Therefore, to study low mass star formation and chemical evolution at different evolutionary stages, 72 cores in different stages—from starless, prestellar to protostar, were studied in our recent survey observation—the ALMA Survey of Orion Planck Galactic Cold Clumps (ALMASOP) (Dutta et al., 2020; Hsu et al., 2020; Sahu et al., 2021). A few starless cores are found to be well traced by N2D+ emission and more compact in nature than other starless cores. Additionally, these cores do not show outflow in CO and SiO emission, which are characteristic of young protostars. Therefore, these cores are suggested to be chemically evolved and represent a class of starless cores on the verge of collapse, which is termed as “prestellar” (Sahu et al., 2021).
High-resolution ( ∼140 au) observations of the ALMASOP detected compact protostars with outflow signatures in the CO and SiO emission. Figure 3 shows one such core with CO outflow (bottom right corner). Though the protostellar temperatures are typically high ( ∼ 100 K), but only few protostars show hot-corino signatures. Low-mass protostars that typically host a hot environment and show the presence of COMs in the gas phase are called hot corinos; this naming separates it from the HCs associated with high mass star-forming regions. Based on the ALMASOP survey, Hsu et al. (2020) reported the detection of five protostars with signatures of COMs like CH3OH, CH3CHO, CH3OCHO, C2H5OH, and NH2CHO. The formation of these molecules mainly occur via ice grain, and during protostellar warm-up, these COMs come into the gas phase. However, it is not clear at which evolutionary stage these hot-corino signatures are observed. Before the ALMASOP observations, there was only one known hot corino in Orion, which is HH 212. In the future, additional high-resolution survey observations of Orion cores using multiple line tracers are necessary to understand the detailed physical and chemical processes related to a hot-corino stage.
The OMC is a well-studied GMC within 500 pc from the Solar system. There are thousands of research articles about the OMC. In this article, we tried to present a concise summary of the physical structures of Orion and their associated chemical properties. In particular, the role of molecular tracer and astrochemistry is vital for understanding molecular clouds’ physical conditions from clumps to core scale. Single dish (e.g., JCMT) studies of Orion were focused on probing clumps, (pc scales) filaments, and large structure of molecular cores (at a scale of ∼ 0.05 pc). To study protostars and various physical processes associated with them, high-resolution interferometric observations are necessary. As an example, the role of chemical tracers in studying the protostar system, HH 212 with an envelope, a disk, and a bipolar outflow, is described. Also, a recent ALMA survey of Orion cores is presented, which offers a zoomed view and reveals embedded protostar with outflows, chemically evolved prestellar cores, hot corinos, and COMs. Future high-resolution studies of Orion regions/cores will further enrich our understanding of molecular cores’ physical and chemical evolution.
DS led the writing of the review and S-YL and TL provided valuable comments and suggestions.
The authors declare that the research was conducted in the absence of any commercial or financial relationships that could be construed as a potential conflict of interest.
All claims expressed in this article are solely those of the authors and do not necessarily represent those of their affiliated organizations, or those of the publisher, the editors and the reviewers. Any product that may be evaluated in this article, or claim that may be made by its manufacturer, is not guaranteed or endorsed by the publisher.
DS and SY-L acknowledge support from the Ministry of Science and Technology, Taiwan (MoST) with Grants 109-2112-M-001-026 and 110-2112-M-001-056. Tie Liu acknowledges the supports by National Natural Science Foundation of China (NSFC) through Grants Nos. 12073061 and 12122307, the international partnership program of Chinese academy of sciences through Grant No. 114231KYSB20200009, and Shanghai Pujiang Program 20PJ1415500.
Aikawa, Y., Ohashi, N., Inutsuka, S. i., Herbst, E., and Takakuwa, S. (2001). Molecular Evolution in Collapsing Prestellar Cores. ApJ 552, 639–653. doi:10.1086/320551
Bally, J., Stark, A. A., Wilson, R. W., and Langer, W. D. (1987). Filamentary Structure in the Orion Molecular Cloud. ApJ 312, L45. doi:10.1086/184817
Blake, G. A., Masson, C. R., Phillips, T. G., and Sutton, E. C. (1986). The Rotational Emission-Line Spectrum of Orion A between 247 and 263 GHz. ApJS 60, 357. doi:10.1086/191090
Blake, G. A., Sutton, E. C., Masson, C. R., and Phillips, T. G. (1987). Molecular Abundances in OMC-1 - the Chemical Composition of Interstellar Molecular Clouds and the Influence of Massive star Formation. ApJ 315, 621. doi:10.1086/165165
Brouillet, N., Despois, D., Baudry, A., Peng, T.-C., Favre, C., Wootten, A., et al. (2013). CH3OCH3 in Orion-KL: a Striking Similarity with HCOOCH3. Astron. Astrophys. 550, A46. doi:10.1051/0004-6361/201219983
Brouillet, N., Despois, D., Lu, X. H., Baudry, A., Cernicharo, J., Bockelée-Morvan, D., et al. (2015). Antifreeze in the Hot Core of Orion. First Detection of Ethylene Glycol in ¡ASTROBJ¿Orion-KL¡/ASTROBJ¿. Astron. Astrophys. 576, A129. doi:10.1051/0004-6361/201424588
Codella, C., Cabrit, S., Gueth, F., Cesaroni, R., Bacciotti, F., Lefloch, B., et al. (2007). A Highly-Collimated SiO Jet in the HH212 Protostellar Outflow. Astron. Astrophys. 462, L53–L56. doi:10.1051/0004-6361:20066800
Codella, C., Ceccarelli, C., Cabrit, S., Gueth, F., Podio, L., Bachiller, R., et al. (2016). Water and Acetaldehyde in HH212: The First Hot Corino in Orion. Astron. Astrophys. 586, L3. doi:10.1051/0004-6361/201527424
Crockett, N. R., Bergin, E. A., Neill, J. L., Favre, C., Schilke, P., Lis, D. C., et al. (2014). Herschel Observations of Extraordinary Sources: Analysis of the HIFI 1.2 THz Wide Spectral Survey toward Orion KL. I. Methods. Astrophys. J. 787, 112. doi:10.1088/0004-637X/787/2/112
Cuadrado, S., Goicoechea, J. R., Pilleri, P., Cernicharo, J., Fuente, A., and Joblin, C. (2015). The Chemistry and Spatial Distribution of Small Hydrocarbons in UV-Irradiated Molecular Clouds: the Orion Bar PDR. Astron. Astrophys. 575, A82. doi:10.1051/0004-6361/201424568
Cuadrado, S., Goicoechea, J. R., Roncero, O., Aguado, A., Tercero, B., and Cernicharo, J. (2016). Trans-cis Molecular Photoswitching in Interstellar Space. Astron. Astrophys. 596, L1. doi:10.1051/0004-6361/201629913
Cuadrado, S., Goicoechea, J. R., Cernicharo, J., Fuente, A., Pety, J., and Tercero, B. (2017). Complex Organic Molecules in Strongly UV-Irradiated Gas. Astron. Astrophys. 603, A124. doi:10.1051/0004-6361/201730459
Dame, T. M., Hartmann, D., and Thaddeus, P. (2001). The Milky Way in Molecular Clouds: A New Complete CO Survey. Astrophys. J. 547, 792–813. doi:10.1086/318388
Dutrey, A., Duvert, G., Castets, A., Langer, W. D., Bally, J., and Wilson, R. W. (1991). Periodically Spaced Fragmentation in Orion A. Astron. Astrophys. 247, L9.
Dutta, S., Lee, C.-F., Liu, T., Hirano, N., Liu, S.-Y., Tatematsu, K., et al. (2020). ALMA Survey of Orion Planck Galactic Cold Clumps (ALMASOP). II. Survey Overview: A First Look at 1.3 Mm Continuum Maps and Molecular Outflows. Astrophys. J. Suppl. Ser. 251, 20. doi:10.3847/1538-4365/abba26
Favre, C., Despois, D., Brouillet, N., Baudry, A., Combes, F., Guélin, M., et al. (2011). HCOOCH3 as a Probe of Temperature and Structure in Orion-KL. Astron. Astrophys. 532, A32. doi:10.1051/0004-6361/201015345
Favre, C., Pagani, L., Goldsmith, P. F., Bergin, E. A., Carvajal, M., Kleiner, I., et al. (2017). The Complexity of Orion: an ALMA View. II. gGg’-Ethylene Glycol and Acetic Acid. Astron. Astrophys. 604, L2. doi:10.1051/0004-6361/201731327
Feng, S., Beuther, H., Henning, T., Semenov, D., Palau, A., and Mills, E. A. C. (2015). Resolving the Chemical Substructure of Orion-KL. Astron. Astrophys. 581, A71. doi:10.1051/0004-6361/201322725
Friedel, D. N., and Widicus Weaver, S. L. (2011). A High Spatial Resolution Study of the λ = 3 Mm Continuum of Orion-KL. Astrophys. J. 742, 64. doi:10.1088/0004-637X/742/2/64
Fuente, A., Rodrıguez-Franco, A., Garcıa-Burillo, S., Martın-Pintado, J., and Black, J. H. (2003). Observational Study of Reactive Ions and Radicals in PDRs. Astron. Astrophys. 406, 899–913. doi:10.1051/0004-6361:20030712
Genzel, R., and Stutzki, J. (1989). The Orion Molecular Cloud and star-forming Region. Annu. Rev. Astron. Astrophys. 27, 41–85. doi:10.1146/annurev.aa.27.090189.000353
Goicoechea, J. R., Pety, J., Cuadrado, S., Cernicharo, J., Chapillon, E., Fuente, A., et al. (2016). Compression and Ablation of the Photo-Irradiated Molecular Cloud the Orion Bar. Nature 537, 207–209. doi:10.1038/nature18957
Goicoechea, J. R., Cuadrado, S., Pety, J., Bron, E., Black, J. H., Cernicharo, J., et al. (2017). Spatially Resolved Images of Reactive Ions in the Orion Bar. Astron. Astrophys. 601, L9. doi:10.1051/0004-6361/201730716
Guzmán, V. V., Pety, J., Gratier, P., Goicoechea, J. R., Gerin, M., Roueff, E., et al. (2014). Chemical Complexity in the Horsehead Photodissociation Region. Faraday Discuss. 168, 103–127. doi:10.1039/C3FD00114H
Heyer, M. H., Morgan, J., Schloerb, F. P., Snell, R. L., and Goldsmith, P. F. (1992). Evidence for Large-Scale Expanding Motions within the Orion A Molecular Cloud. Astrophys. J. Lett. 395, L99. doi:10.1086/186497
Hirota, T., and Yamamoto, S. (2006). Molecular Line Observations of Carbon-Chain-Rich Core L492. Astrophys. J. 646, 258–268. doi:10.1086/504866
Hollenbach, D. J., and Tielens, A. G. G. M. (1999). Photodissociation Regions in the Interstellar Medium of Galaxies. Rev. Mod. Phys. 71, 173–230. doi:10.1103/RevModPhys.71.173
Hsu, S.-Y., Liu, S.-Y., Liu, T., Sahu, D., Hirano, N., Lee, C.-F., et al. (2020). ALMA Survey of Orion Planck Galactic Cold Clumps (ALMASOP). I. Detection of New Hot Corinos with the ACA. Astrophys. J. 898, 107. doi:10.3847/1538-4357/ab9f3a
Ikeda, N., Kitamura, Y., and Sunada, K. (2009). A Survey of Dense Cores in the Orion B Cloud. Astrophys. J. 691, 1560–1582. doi:10.1088/0004-637x/691/2/1560
Jansen, D. J., van Dishoeck, E. F., Black, J. H., Spaans, M., and Sosin, C. (1995). Physical and Chemical Structure of the IC 63 Nebula. II. Chemical Models. Astron. Astrophys. 302, 223.
Johansson, L. E. B., Andersson, C., Ellder, J., Friberg, P., Hjalmarson, A., Hoglund, B., et al. (1984). Spectral Scan of Orion A and IRC +10216 from 72 to 91 GHz. Astron. Astrophys. 130, 227–256.
Johnstone, D., Boonman, A. M. S., and van Dishoeck, E. F. (2003). Astrochemistry of Sub-millimeter Sources in Orion. Studying the Variations of Molecular Tracers with Changing Physical Conditions. Astron. Astrophys. 412, 157–174. doi:10.1051/0004-6361:20031370
Lai, S.-P., and Crutcher, R. M. (2000). 33.8 GHZ CCS Survey of Molecular Cores in Dark Clouds. Astrophys. J. Suppl. Ser. 128, 271–286. doi:10.1086/313372
Lee, C.-F., Hirano, N., Zhang, Q., Shang, H., Ho, P. T. P., and Krasnopolsky, R. (2014). ALMA Results of the Pseudodisk, Rotating Disk, and Jet in the Continuum and HCO+ in the Protostellar System HH 212. Astrophys. J. 786, 114. doi:10.1088/0004-637X/786/2/114
Lee, C.-F., Ho, P. T. P., Li, Z.-Y., Hirano, N., Zhang, Q., and Shang, H. (2017). A Rotating Protostellar Jet Launched from the Innermost Disk of HH 212. Nat. Astron. 1, 0152. doi:10.1038/s41550-017-0152
Liszt, H. S., Lucas, R., and Pety, J. (2006). Comparative Chemistry of Diffuse Clouds. V. Ammonia and Formaldehyde. Astron. Astrophys. 448, 253–259. doi:10.1051/0004-6361:20054071
Liu, S.-Y., Girart, J. M., Remijan, A., and Snyder, L. E. (2002). Formic Acid in Orion KL from 1 Millimeter Observations with the Berkeley-Illinois-Maryland Association Array. Astrophys. J. 576, 255–263. doi:10.1086/341620
Maddalena, R. J., Morris, M., Moscowitz, J., and Thaddeus, P. (1986). The Large System of Molecular Clouds in Orion and Monoceros. Astrophys. J. 303, 375. doi:10.1086/164083
Nishimura, A., Tokuda, K., Kimura, K., Muraoka, K., Maezawa, H., Ogawa, H., et al. (2015). Revealing the Physical Properties of Molecular Gas in Orion with a Large-Scale Survey in J = 2-1 Lines of 12CO, 13CO, and C18O. Astrophys. J. Suppl. Ser. 216, 18. doi:10.1088/0067-0049/216/1/18
Orozco-Aguilera, M. T., Zapata, L. A., Hirota, T., Qin, S.-L., and Masqué, J. M. (2017). ALMA Observations of the Archetypal “Hot Core” that Is Not: Orion-KL. Astrophys. J. 847, 66. doi:10.3847/1538-4357/aa88cd
Pagani, L., Favre, C., Goldsmith, P. F., Bergin, E. A., Snell, R., and Melnick, G. (2017). The Complexity of Orion: an ALMA View. I. Data and First Results. Astron. Astrophys. 604, A32. doi:10.1051/0004-6361/201730466
Pagani, L., Bergin, E., Goldsmith, P. F., Melnick, G., Snell, R., and Favre, C. (2019). The Complexity of Orion: an ALMA View. III. The Explosion Impact. Astron. Astrophys. 624, L5. doi:10.1051/0004-6361/201935267
Peng, T. C., Despois, D., Brouillet, N., Parise, B., and Baudry, A. (2012). Deuterated Methanol in Orion BN/KL. Astron. Astrophys. 543, A152. doi:10.1051/0004-6361/201118310
Peng, Y., Qin, S.-L., Schilke, P., Sánchez-Monge, Á., Wu, Y., Liu, T., et al. (2017). ALMA Observations of Vibrationally Excited HC3N Lines toward Orion KL. Astrophys. J. 837, 49. doi:10.3847/1538-4357/aa5c81
Pety, J., Gratier, P., Guzmán, V., Roueff, E., Gerin, M., Goicoechea, J. R., et al. (2012). The IRAM-30 M Line Survey of the Horsehead PDR. II. First Detection of the L-C3h+ Hydrocarbon Cation. Astron. Astrophys. 548, A68. doi:10.1051/0004-6361/201220062
Rieke, G. H., Low, F. J., and Kleinmann, D. E. (1973). High-Resolution Maps of the Kleinmann-Low Nebula in Orion. Astrophys. J. Lett. 186, L7. doi:10.1086/181346
Rizzo, J. R., Tercero, B., and Cernicharo, J. (2017). A Spectroscopic Survey of Orion KL between 41.5 and 50 GHz. Astron. Astrophys. 605, A76. doi:10.1051/0004-6361/201629936
Sahu, D., Minh, Y. C., Lee, C.-F., Liu, S.-Y., Das, A., Chakrabarti, S. K., et al. (2018). Deuterated Formaldehyde in the Low-Mass Protostar HH212. MNRAS 475, 5322–5329. doi:10.1093/mnras/sty190
Sahu, D., Liu, S.-Y., Liu, T., Evans, I., Neal, J., Hirano, N., et al. (2021). ALMA Survey of Orion Planck Galactic Cold Clumps (ALMASOP): Detection of Extremely High-Density Compact Structure of Prestellar Cores and Multiple Substructures within. Astrophys. J. Lett. 907, L15. doi:10.3847/2041-8213/abd3aa
Sakai, T., Sakai, N., Furuya, K., Aikawa, Y., Hirota, T., and Yamamoto, S. (2012). DNC/HNC Ratio of Massive Clumps in Early Evolutionary Stages of High-Mass Star Formation. Astrophys. J. 747, 140. doi:10.1088/0004-637X/747/2/140
Sutton, E. C., Blake, G. A., Masson, C. R., and Phillips, T. G. (1985). Molecular Line Survey of Orion A from 215 to 247 GHz. Astrophysical J. Suppl. Ser. 58, 341–378. doi:10.1086/191045
Sutton, E. C., Peng, R., Danchi, W. C., Jaminet, P. A., Sandell, G., and Russell, A. P. G. (1995). The Distribution of Molecules in the Core of OMC-1. Astrophys. J. Suppl. Ser. 97, 455. doi:10.1086/192147
Tabone, B., Cabrit, S., Bianchi, E., Ferreira, J., Pineau des Forêts, G., Codella, C., et al. (2017). ALMA Discovery of a Rotating SO/SO2 Flow in HH212. A Possible MHD Disk Wind? Astron. Astrophys. 607, L6. doi:10.1051/0004-6361/201731691
Tatematsu, K., Ohashi, S., Umemoto, T., Lee, J.-E., Hirota, T., Yamamoto, S., et al. (2014). Chemical Variation in Molecular Cloud Cores in the Orion A Cloud. II. Publ. Astron. Soc. Jpn. 66, 16. doi:10.1093/pasj/pst016
Tatematsu, K., Liu, T., Ohashi, S., Sanhueza, P., Nguyen Lu’o’ng, Q., Hirota, T., et al. (2017). Astrochemical Properties of Planck Cold Clumps. Astrophys. J. Suppl. Ser. 228, 12. doi:10.3847/1538-4365/228/2/12
Tercero, B., Cernicharo, J., Pardo, J. R., and Goicoechea, J. R. (2010). A Line Confusion Limited Millimeter Survey of Orion KL . I. Sulfur Carbon Chains. Astron. Astrophys. 517, A96. doi:10.1051/0004-6361/200913501
Tercero, B., Vincent, L., Cernicharo, J., Viti, S., and Marcelino, N. (2011). A Line-Confusion Limited Millimeter Survey of Orion KL. II. Silicon-Bearing Species. Astron. Astrophys. 528, A26. doi:10.1051/0004-6361/201015837
Tercero, B., Cernicharo, J., López, A., Brouillet, N., Kolesniková, L., Motiyenko, R. A., et al. (2015). Searching for Trans Ethyl Methyl Ether in Orion KL⋆. Astron. Astrophys. 582, L1. doi:10.1051/0004-6361/201526255
Turner, B. E. (1991). A Molecular Line Survey of Sagittarius B2 and Orion–KL from 70 to 115 GHz. II. Analysis of the Data. Astrophys. J. Suppl. Ser. 76, 617. doi:10.1086/191577
Ungerechts, H., Bergin, E. A., Goldsmith, P. F., Irvine, W. M., Schloerb, F. P., and Snell, R. L. (1997). Chemical and Physical Gradients along the OMC-1 Ridge. Astrophys. J. 482, 245–266. doi:10.1086/304110
van Dishoeck, E. F., and Black, J. H. (1988). The Photodissociation and Chemistry of Interstellar CO. Astrophys. J. 334, 771. doi:10.1086/166877
Wang, S., Bergin, E. A., Crockett, N. R., Goldsmith, P. F., Lis, D. C., Pearson, J. C., et al. (2011). Herschel Observations of EXtra-ordinary Sources (HEXOS): Methanol as a Probe of Physical Conditions in Orion KL. Astron. Astrophys. 527, A95. doi:10.1051/0004-6361/201015079
Wright, M. C. H., and Plambeck, R. L. (2017). ALMA Images of the Orion Hot Core at 349 GHz. Astrophys. J. 843, 83. doi:10.3847/1538-4357/aa72e6
Yi, H.-W., Lee, J.-E., Liu, T., Kim, K.-T., Choi, M., Eden, D., et al. (2018). Planck Cold Clumps in the λ Orionis Complex. II. Environmental Effects on Core Formation. Astrophys. J. Suppl. Ser. 236, 51. doi:10.3847/1538-4365/aac2e0
Yi, H.-W., Lee, J.-E., Kim, K.-T., Liu, T., Lim, B., and Tatematsu, K. (2021). Planck Cold Clumps in the Lambda Orionis Complex. III. A Chemical Probe of Stellar Feedback on Cores in the Lambda Orionis Cloud. arXiv e-prints. arXiv:2103.03499.
Zapata, L. A., Schmid-Burgk, J., and Menten, K. M. (2011). Orion KL: the Hot Core that Is Not a “Hot Core”. Astron. Astrophys. 529, A24. doi:10.1051/0004-6361/201014423
Keywords: orion molecular cloud, astrochemistry, star-formations, (sub)millimeter astronomy, single dish and interferometers
Citation: Sahu D, Liu S-Y and Liu T (2021) Anatomy of Orion Molecular Clouds—The Astrochemistry Perspective/Approach. Front. Astron. Space Sci. 8:672893. doi: 10.3389/fspas.2021.672893
Received: 26 February 2021; Accepted: 05 August 2021;
Published: 15 October 2021.
Edited by:
Luca Bizzocchi, Max Planck Institute for Extraterrestrial Physics, GermanyReviewed by:
Susanna L. Widicus Weaver, University of Wisconsin-Madison, United StatesCopyright © 2021 Sahu, Liu and Liu. This is an open-access article distributed under the terms of the Creative Commons Attribution License (CC BY). The use, distribution or reproduction in other forums is permitted, provided the original author(s) and the copyright owner(s) are credited and that the original publication in this journal is cited, in accordance with accepted academic practice. No use, distribution or reproduction is permitted which does not comply with these terms.
*Correspondence: Dipen Sahu, ZHNhaHVAYXNpYWEuc2luaWNhLmVkdS50dw==
Disclaimer: All claims expressed in this article are solely those of the authors and do not necessarily represent those of their affiliated organizations, or those of the publisher, the editors and the reviewers. Any product that may be evaluated in this article or claim that may be made by its manufacturer is not guaranteed or endorsed by the publisher.
Research integrity at Frontiers
Learn more about the work of our research integrity team to safeguard the quality of each article we publish.