- 1Departament de Química, Universitat Autònoma de Barcelona, Bellaterra, Spain
- 2Univ. Grenoble Alpes, CNRS, Institut de Planétologie et d'Astrophysique de Grenoble (IPAG), Grenoble, France
- 3Dipartimento di Chimica, Biologia e Biotecnologie, Università degli Studi di Perugia, Perugia, Italy
- 4Dipartimento di Chimica and Nanostructured Interfaces and Surfaces (NIS) Centre, Università degli Studi di Torino, Torino, Italy
In cold galactic molecular clouds, dust grains are coated by icy mantles and are prevalently charged negatively, because of the capture of the electrons in the gas. The interaction of the charged grains with gaseous cations is known to neutralize them. In this work, we focus on the chemical consequences of the neutralization process of HCO+, often the most abundant cation in molecular clouds. More specifically, by means of electronic structure calculations, we have characterized the energy and the structure of all possible product species once the HCO+ ion adsorbs on water clusters holding an extra electron. Two processes are possible: (i) electron transfer from the negative water cluster to the HCO+ ion or (ii) a proton transfer from HCO+ to the negative water cluster. Energetic considerations favor electron transfer. Assuming this scenario, two limiting cases have been considered in astrochemical models: (a) all the neutralized HCO+ is retained as neutral HCO adsorbed on the ice and (b) all the neutralized HCO+ gets desorbed to the gas phase as HCO. None of the two limiting cases appreciably contribute to the HCO abundance on the grain surfaces or in the gas.
Introduction
The identification of about 200 molecular species in the interstellar medium (ISM) through their rotational (millimeter), rovibrational (infrared), and/or electronic (ultraviolet or visible) transitions is the proof of the existence of a rich molecular universe in which a multitude of chemical processes take place (Caselli and Ceccarelli, 2012; Tielens, 2013; Van Dishoeck, 2014). Understanding the chemical and physical bases that drive the formation of these molecules in the harsh conditions of the ISM is a current challenge to the Astrochemistry community. Many of these species are formed in reaction chains taking place in the gas phase (for some recent examples, see, e.g., Shannon et al., 2013; Vasyunin and Herbst, 2013; Balucani et al., 2015; Skouteris et al., 2017, 2018, 2019; Vazart et al., 2020), but in some cases their production occurs in or on the icy mantle of interstellar dust grains. Interstellar grains, indeed, can act as a catalyzer or simply as a concentrator of the reactants, by impeding them to fly apart after non-reactive encounters. Several models have been developed to account for the chemistry on interstellar ice (e.g., Garrod and Herbst, 2006; Taquet et al., 2012; Agúndez and Wakelam, 2013; Rimola et al., 2014; Penteado et al., 2017; Loison et al., 2019; Grassi et al., 2020). The role of interstellar grains is especially important for the synthesis of those molecules that cannot be formed in gas-phase reactions, such as the most important interstellar molecule H2 (Vidali, 2013; Wakelam et al., 2017).
Remarkably, ice-assisted chemical synthesis can be activated by UV and high-energy radiation (e.g., X-rays and energetic particles like cosmic rays). The interaction of energetic particles with matter generates many non-thermal secondary low-energy (<20 eV) electrons (LEE), which may themselves induce chemical reactions (Arumainayagam et al., 2010; Alizadeh and Sanche, 2012). In an astrochemical context, LEE may result from the interaction of cosmic rays with gaseous H2 in dense clouds (Oka, 2013) and inelastic collisions of cosmic rays when traversing through the ices (Pimblott and Laverne, 2007). The number density of free electrons in molecular clouds is estimated to be ca. ne = 10−4 cm−3 (Evans, 1993; Rae et al., 2004; Mason and Field, 2019). As previously argued, the probability that interstellar dust grains can capture free electrons is high. It has been long recognized, indeed, that negatively charged grains are present in the interstellar medium (Draine and Sutin, 1987) and that their recombination with positive ions dominates their neutralization (Walmsley et al., 2004; Ceccarelli and Dominik, 2005). Primary electron attachment can also induce chemical transformations because of the production of reactive species (Boamah et al., 2014; Mason et al., 2014).
According to the work by Hatano et al. (2011), LEE are thermalized within approximately 1 ps via inelastic collisions. When the ice is predominantly formed by water molecules, the captured electrons can be treated as metastable solvated electrons. The plausibility of this scenario is demonstrated by experiments where molecules embedded in water ice irradiated by electrons at 100–400 eV yield the desorption of the molecular species followed by the formation of solvated electrons (Thrower et al., 2011).
In the present work, we aim to characterize the role of fully equilibrated solvated electrons in the chemistry of interstellar icy grains by means of quantum chemical calculations complemented by astrochemical modeling. To this end, we have described, as a test case, the interaction of a negatively charged icy grain with the HCO+ ion, the most abundant molecular ion in molecular clouds (average abundance with respect to H2 of about 10−8). Specifically, we have modeled the negative icy grain with a cluster of water molecules containing an extra electron (so-called solvated electron) and its possible interactions with the ion. We have also scrutinized the possible outcomes of such interactions, which are the following:
1) formation of the neutral HCO radical adsorbed on the water ice grain surfaces through an electron transfer from W− (i.e., the water cluster with the extra electron) to HCO+:
where W⋯HCO represents the radical HCO interacting with water ice W;
2) formation of CO adsorbed on the water ice grain surfaces through proton transfer from HCO+ to W−:
where HW⋯CO represents CO interacting with the water ice with an extra, covalently bound H atom;
3) formation of CO and H, both adsorbed on the water ice grain surfaces, due to dissociative electron transfer from W− to HCO+:
where H⋯W⋯CO represents CO and H simultaneously adsorbed on the water ice.
Finally, we have tried to address the possible astrophysical implications of the present quantum calculations. We remind the readers that HCO is an important intermediate species in the formation of H2CO and, subsequently, CH3OH by CO hydrogenation on ice. In addition, gaseous formyl radical has been widely detected in interstellar objects and its formation routes are poorly constrained (see below). New observational results on the gaseous HCO radical have recently been reported, including an analysis of its abundance to elucidate its origin (Bacmann and Faure, 2016). Since the electron–ion recombination liberates a large amount energy (as large as the ionization potential of the HCO radical, namely, 8.12 ± 0.04 eV), HCO or its moieties H and CO, once formed on ice, can chemically desorb into the gas phase. We will therefore tackle the possible effects of processes (1)–(3) either in the chemistry of ice or in the chemistry of the gas phase.
Methods
Quantum Chemical Calculations
As mentioned in the Introduction, the negatively charged icy grains have been modeled in this work as water clusters of different sizes containing one extra electron. In other words, we consider that the interaction of the additional electron occurs only with the water molecules of the icy mantles of grains. The electronic nature of the solvated electron has been extensively studied in physical and quantum chemistry (Turi and Rossky, 2012; Young and Neumark, 2012). Quantum chemical calculations based on large water clusters converge to the same description, that is, the extra electron can be either localized within the cavity of the water clusters, on the surface of the clusters in a localized fashion, or delocalized along the cluster surface. The preference for those forms depends on the structure and size of the water cluster (Kim et al., 1996, 1997; Lee et al., 1997; Khan, 2003, 2004, 2005, 2006; Herbert and Head-Gordon, 2005, 2006). To contemplate all the possible arrangements, in this work we have considered three well-described water clusters with one solvated electron (see Figure 1): (i) a 14 H2O-molecule cluster (Khan, 2006), in which the extra electron is found inside the cavity or localized on the surface cluster (-cav and -loc, respectively); (ii) a 20 H2O-molecule cluster (Khan, 2003, 2005; Herbert and Head-Gordon, 2005, 2006), in which the extra electron is localized or delocalized on the cluster surfaces (-loc and -deloc, respectively); and (iii) a 24 H2O-molecule cluster (Khan, 2004; Herbert and Head-Gordon, 2005, 2006), which can present the extra electron within the cavity, localized on the surface, or delocalized on the surface (-cav, -loc, and -deloc, respectively). For the sake of clarity, along this work we will use the “-xy” notation for the water cluster systems, in which W− will refer to water clusters with the extra electron, N refers to the number of water molecules composing the water cluster, and the xy term indicates if the extra electron is inside the cavity (xy=cav), is localized on the cluster surface (xy=loc), or is delocalized on the cluster surface (xy=deloc).
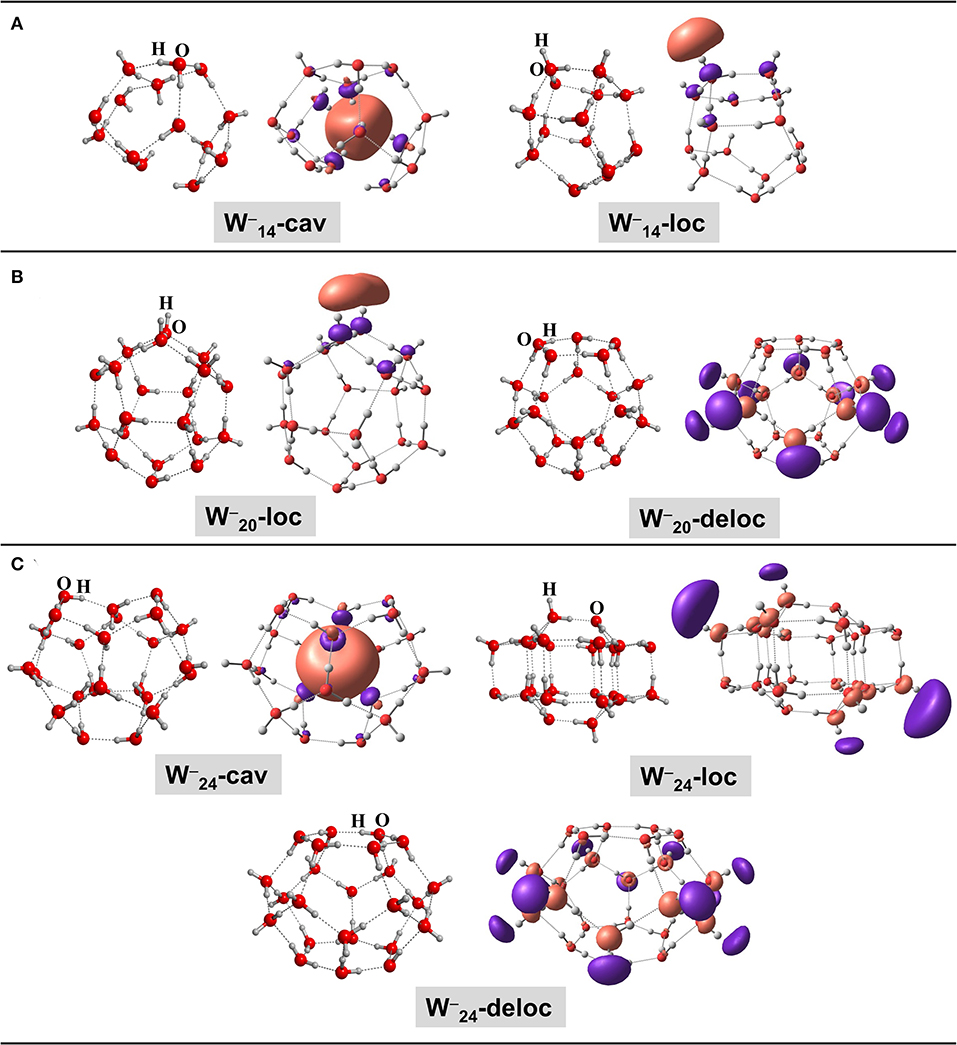
Figure 1. BHYLP/6-31(1+,3+)G(d,p)-optimized geometries of the water clusters with 14 (A), 20 (B), and 24 (C) H2O molecules with the extra electron (, , and , respectively) inside the cavity (cav), localized at the surface of the water clusters (loc) and delocalized at the surface of the clusters (deloc). The singly occupied molecular orbitals (SOMO), which represent the localization of the extra electron, are also shown.
Concerning the calculations including H/C/O, the initial guess structures were manually built: (i) for the W⋯HCO complexes, the initial guess features HCO+ interacting through its O atom end with one H atom of the H2O cluster ices, (ii) for the HW⋯CO complexes, the CO molecule is interacting with one H2O molecule of the cluster ices, while the H atom is incorporated into another water molecule of the cluster, thus forming an H3O group, and (iii) for the H⋯W⋯CO complexes both CO and H are interacting with different water molecules of the cluster ices. Following the work by Herbert and Head-Gordon (Herbert and Head-Gordon, 2005, 2006), geometry optimization has been carried out by using the BHLYP hybrid density functional method with a 6-31(1+,3+)G(d,p) basis set, which is the 6-31++G(d,p) standard one with two more sets of diffuse s functions on each H atom (with exponents 1.0843373500 × 10−2 and 3.2660763500 × 10−3, and coefficients 1.00). Single-point energy calculations on the optimized geometries at the MP2 post-Hartree–Fock method employing a 6-311(2+,3+)G(d,p) basis set [namely, the 6-311++G(d,p) standard one including an additional set of diffuse sp functions on each O and C atom (for O, with exponent 2.5451807230 × 10−2 and coefficient 1.00; for C, with exponent 1.3192771080 × 10−2 and coefficient 1.00) and the two additional sets of diffuse s functions on each H atom] have been performed to calculate the reaction energies of processes (1), (2), and (3). It is worth mentioning that more sophisticated treatments on electron capture phenomena are possible, including quantum effects (Flower and Middleton, 2004). However, our systems are relatively large to be simulated by this methodology (with a significant increase of the computational cost) and, since the aim of the work is mainly on the energetics of the studied processes, we consider the used level of computation to be accurate enough.
Astrochemical Modeling
To assess the implications of our new computations on the chemical composition of the molecular cloud gas, we used the GRAINOBLE code (Taquet et al., 2012, 2013). Briefly, GRAINOBLE is a gas-grain time-dependent astrochemical model, which takes into account the grain-surface hydrogenation and oxidation plus the multilayered structure of the grain mantles. We assumed the standard elemental abundances (see, e.g., Agúndez and Wakelam, 2013), a cloud with a density of 104 cm−3, a temperature of 10 K, and a cosmic ray ionization rate of 3 × 10−17 s−1 and run the code up to 108 year.
Results and Discussion
Figures 2–4 show the optimized geometries for the relevant species in the (1)–(3) processes. In the same Figures, the reaction energies (bare values) and the relative energies (values in brackets) are also shown. Different optimized structures were obtained, but only the most stable ones are presented here for the sake of clarity and brevity.
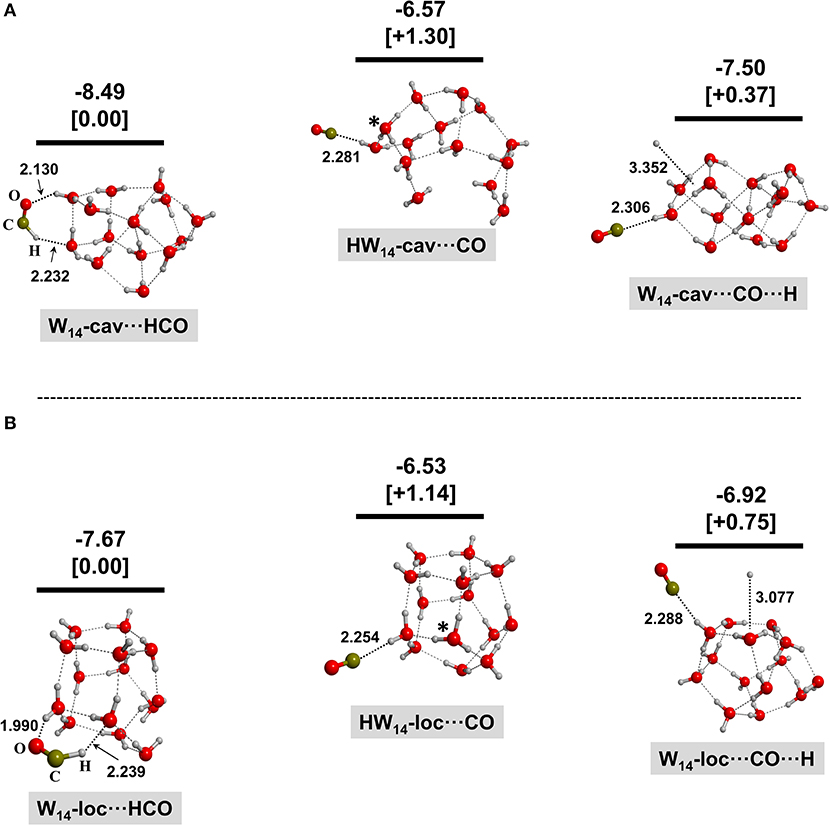
Figure 2. BHYLP/6-31(1+,3+)G(d,p)-optimized structures for the interaction of HCO+ with the 14-water-molecule clusters with an extra electron inside the cavity (A) and localized at the surface (B). Structures on the left correspond to the electron transfer from W−14 to HCO+; structures in the middle correspond to the H transfer from HCO+ to W−14 (the transferred H is labeled by an asterisk); and structures on the right correspond to the dissociation of HCO resulting with the adsorption of CO and H on the water ice clusters. Bare values are the calculated reaction energies taking as the zero energy reference the W−14 + HCO+ asymptote; values in brackets are the relative energies of the different complexes belonging to the same system. These energetics values are calculated at the MP2/6-311(2+,3+)G(d,p)//BHYLP/6-31(1+,3+)G(d,p) theory level. Energy units are in eV, and bond distances are in Å.
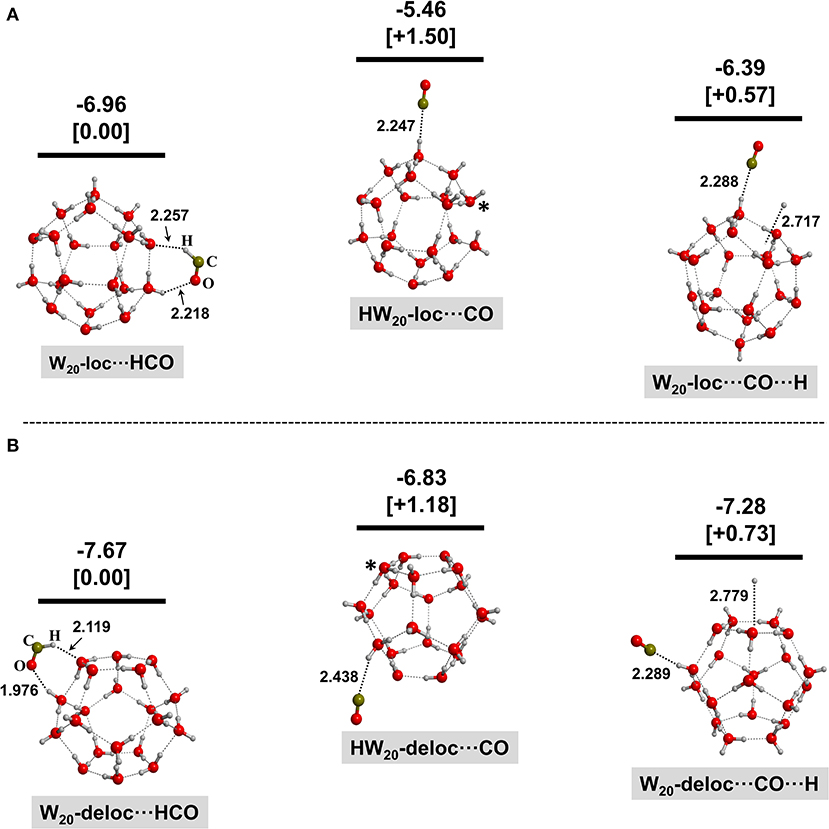
Figure 3. BHYLP/6-31(1+,3+)G(d,p)-optimized structures for the interaction of HCO+ with the 20-water-molecule clusters with an extra electron localized at the surface (A) and delocalized at the surface (B). Structures on the left correspond to the electron transfer from W−20 to HCO+; structures in the middle correspond to the H transfer from HCO+ to W−20 (the transferred H is labeled by an asterisk); and structures on the right correspond to the dissociation of HCO resulting in the adsorption of CO and H on the water ice clusters. Bare values are the calculated reaction energies taking as the zero energy reference the W−20 + HCO+ asymptote; values in brackets are the relative energies of the different complexes belonging to the same system. These energetics values are calculated at the MP2/6-311(2+,3+)G(d,p)//BHYLP/6-31(1+,3+)G(d,p) theory level. Energy units are in eV, and bond distances are in Å.
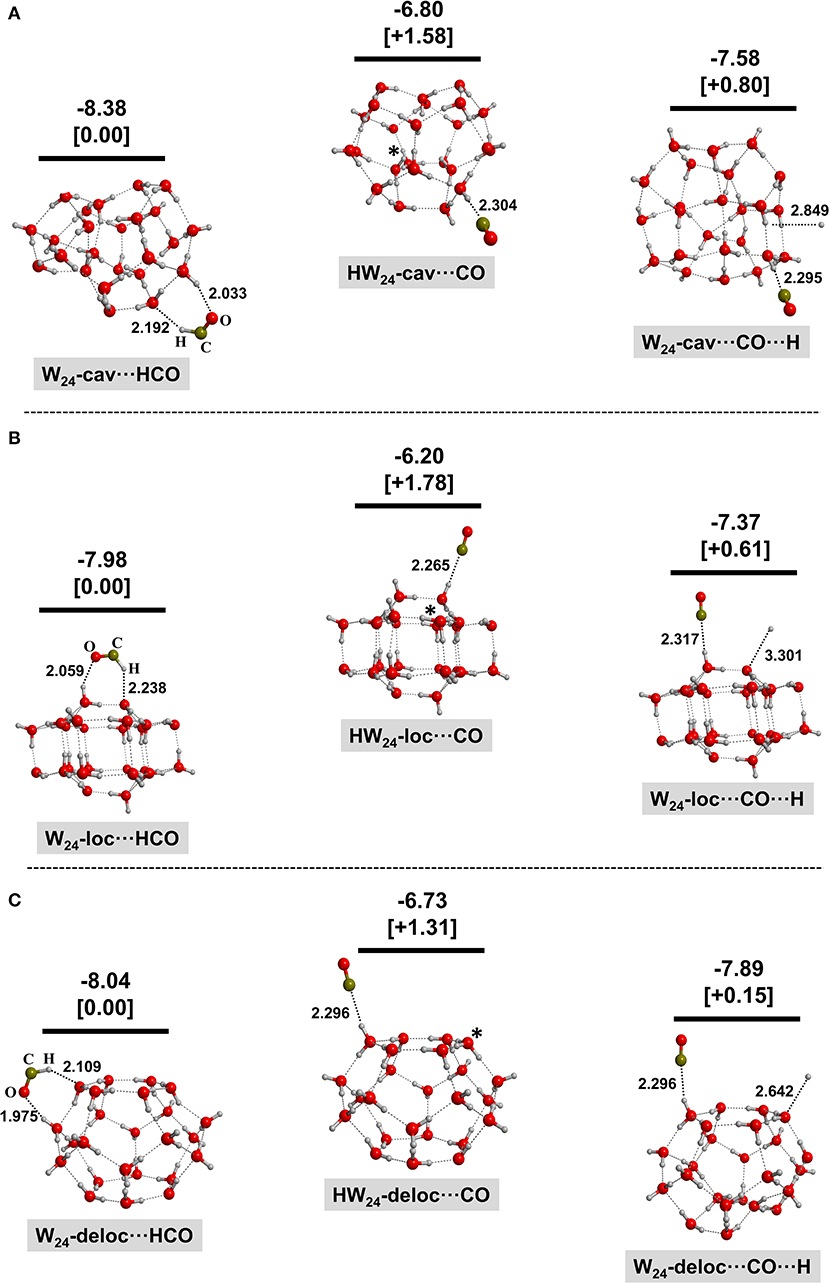
Figure 4. BHYLP/6-31(1+,3+)G(d,p)-optimized structures for the interaction of HCO+ with the 24-water-molecule clusters with an extra electron inside the cavity (A), localized at the surface (B), and delocalized at the surface (C). Structures on the left correspond to the electron transfer from W−24 to HCO+; structures in the middle correspond to the H transfer from HCO+ to W−24 (the transferred H is labeled by an asterisk); and structures on the right correspond to the dissociation of HCO resulting in the adsorption of CO and H on the water ice clusters. Bare values are the calculated reaction energies taking as the zero energy reference the W−24 + HCO+ asymptote; values in brackets are the relative energies of the different complexes belonging to the same system. These energetics values are calculated at the MP2/6-311(2+,3+)G(d,p)//BHYLP/6-31(1+,3+)G(d,p) theory level. Energy units are in eV, and bond distances are in Å.
For all the W⋯HCO cases, the initial HCO+ species (a linear molecule) becomes bent during the geometry optimization. Analysis of the spin density confirms that the unpaired electron is fully localized on the C atom, indicating that the extra electron is transferred to form the HCO radical (process 1). Moreover, in all the analyzed cases, the newly formed HCO group interacts with the water ice clusters via hydrogen bond (H-bond) interactions with both the H and O ends acting as donor and acceptor, respectively.
For the HW⋯CO complexes resulting after the proton transfer process (2), in all the considered cases, the incorporated H atom remains accommodated in the ice as a H3O radical species (labeled by an asterisk in the Figures), indicating that it is stable under these conditions.
Finally, in the case of the dissociative electron–ion recombination (3), the resulting H⋯W⋯CO complexes feature the CO moiety interacting with the water ice clusters similarly to the HW⋯CO systems, but the coproduct H atom is not covalently bound to a water molecule but simply physisorbed on water ice clusters.
By comparing the energy content of the possible final complexes, it is clear that the formation of W⋯HCO is more favorable than the formation of HW⋯CO and H⋯W⋯CO by ca. 1.15–1.78 eV and 0.15–0.80 eV, respectively. Even though the system will have enough energy to overcome those differences because of the large amount of energy liberated in all the three families of processes (1)–(3), these results imply that HCO+ neutralization on a negative grain is a possible route of HCO formation directly on the ice and without the need to surmount a potential energy barrier. This is at variance with HCO formation via H addition to CO for which a sizable barrier is present (Woon, 2002; Marenich and Boggs, 2003; Goumans et al., 2007, 2008; Andersson et al., 2011; Peters et al., 2013; Rimola et al., 2014). In other words, the interaction of HCO+ with a negatively charged grain readily leads to the formation of HCO directly adsorbed on the ice.
Interestingly, all the three situations are below the “W− + HCO+(gas)” asymptote and accordingly the three products arising from process (1)–(3) can be populated. Nonetheless, the energy liberated in the formation of W⋯HCO by (1) is very large (between −7.0 and −8.0 eV) and it is reasonable to consider that part of this large nascent energy can be used to break the H–C bond of the HCO radical yielding the formation of the H⋯W⋯CO complexes, which is process (3). We have calculated the energy barriers for the H–C fission in the W24-cav⋯HCO, W24-loc⋯HCO, and W24-deloc⋯HCO cases. Their values (1.75, 1.35, and 2.01 eV, respectively) are still largely below the asymptote, thus supporting this idea. On the other hand, the energetics involved in the formation of W⋯HCO, process (1), is dictated by the difference in the electron affinity of HCO+ and of neutral water ice, while in HW⋯CO, process (2) is dictated by the proton affinity of CO and W-, respectively. The value of the electron affinity for HCO+ and the W24-cav water ice cluster (here W24-cav is used here as a test case of the water ice) is −7.67 and −0.52 eV, respectively, in our calculations (see reactions entitled “Electron affinity” of Table 1), while the calculated proton affinity of CO and -cav is, instead, −6.51 and −13.01 eV, respectively (see reactions entitled “Proton affinity” of Table 1). The larger electron affinity of HCO+ compared to W24-cav indicates that the solvated electron prefers to be transferred to HCO+ to form HCO, whereas the larger proton affinity of -cav compared to CO indicates that proton transfer to -cav to form HW24-cav is also energetically favorable. Thus, it seems there is a competition between processes (1) + (3) and (2), namely, between electron–ion recombination (dictated by electron transfer) and proton transfer. Additionally, for process (2), an energy barrier associated with the H transfer from HCO+ to form the H3O radical is expected, since the formed H3O radical is a metastable species in the clusters. Such a stability of the solvated H3O radical has already been reported by Chulkov et al. (2009).
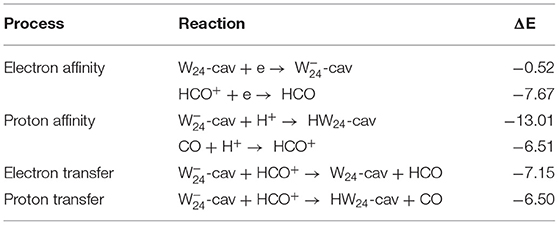
Table 1. Reaction energies (ΔE, in eV) calculated at the MP2/6-311(2+,3+)G(d,p)//BHYLP/6-31(1+,3+)G(d,p) theory level corresponding to (i) the electron affinity of W24-cav and HCO+, (ii) the proton affinity of -cav and CO, (iii) the electron transfer from -cav to the HCO+ cations, and (iv) the H+ transfer from the HCO+ cation to -cav.
By considering the involved energy, the competition between these processes seems to be favored by the electron–ion recombination (the calculated reaction energies for these processes are shown in Table 1 indicating that for the electron transfer is −7.15 eV whereas for the proton transfer is −6.50 eV). In addition to that, from a dynamical point of view, electron transfer is naturally favored by the much smaller mass of the electron compared to the proton. We can also expect that the interaction between HCO+ and the additional electron of the water clusters will start at a much longer distance, as the impact parameters and cross sections are usually much larger in the case of electron transfer rather than proton transfer (Prigogine and Rice, 1973). In other words, both energetics and the dynamical considerations favor processes (1) + (3) as dominant over process (2).
It is worth mentioning that these reaction energies are less negative by some amount than the calculated reaction energies for the formation of W24-cav⋯HCO and HW24-cav⋯CO from the interaction of HCO+ with -cav (−8.38 and −6.80 eV, respectively), because the W24-cav⋯HCO and HW24-cav⋯CO structures account for the stabilizing H-bond interaction between the two partners.
Astrophysical Implications
The present calculations only offer a qualitative picture of the title process, as they do not provide reaction rate coefficients to be introduced in astrochemical models. The generation of those rate coefficients would require a rigorous treatment of the energy dissipation between all the degrees of freedom of the cluster molecules (or better, of the icy mantle of interstellar grain) once the energy associated with the electron–ion recombination (or proton transfer) is liberated. Such a treatment is a daunting task adopting state-of-the-art theoretical treatments, even though the first studies are appearing in the literature (Fredon et al., 2017; Fredon and Cuppen, 2018; Pantaleone et al., 2020). This is probably one of the main challenges in the characterization of chemistry occurring on interstellar ice. However, based on the two cited works, a large fraction (≥70%) of the reaction energy is absorbed very quickly, in <1 ps, by the water molecules of the ice. Relevant to this study, Pantaleone et al. (2020) studied the fate of the energy released by the H + CO reaction on the icy surface and concluded that the 137 kJ mol−1 released by the reaction is largely absorbed by the icy molecules and that the newly formed HCO only possesses <20 kJ mol−1, a too low energy for it to break the bonds with the ice and be liberated into the gas.
However, missing specific simulations for the case studied here, namely, HCO+ + e−, we consider the two possible scenarios. If most of reaction energy is quickly redistributed along all the degrees of freedom of the ice-connected molecules, then HCO can remain adsorbed on the ice as such; in this same scenario, we might have the breaking up of the H–C bond, but with both moieties still adsorbed on the ice. Alternatively, the large amount of energy liberated by the electron–ion recombination will induce the chemical desorption of the whole HCO or even of its two moieties H and CO.
Trying to assess the possible effects of the electron–ion recombination process on interstellar ice without knowing the relative importance of the different scenarios, we will consider two limiting cases: case (a) HCO remains in the grain as such, thus becoming available to further hydrogenation up to H2CO and, eventually, CH3OH; case (b) HCO survives as such, but it is immediately released in the gas-phase by chemisorption.
Case (a)
By employing the GRAINOBLE code (Taquet et al., 2012, 2013), the present authors have demonstrated that, when considering the energy barriers determined by quantum calculations of the same kind as those presented here to characterize CO hydrogenation (Rimola et al., 2014), the amount of H2CO that can easily be accounted for (in spite of the barriers and thanks to the tunnel effect) is n(H2CO)/n(H) = 10−6-10−5. Now, in the most favorable case, n(HCO+)/n(H) can be as high as the HCO+ abundance, namely, about 10−8 (see section Introduction). Therefore, even in the limiting case that all HCO+ is systematically converted into HCO adsorbed on water ice, this process can only account up to about 1% of the total H2CO produced on ice. In other words, the title process has a negligible effect on the amount of H2CO produced on interstellar grains.
Case (b)
The formyl HCO radical is one of the first molecules detected in space (Snyder et al., 1976) and a rather ubiquitous molecule found relatively easily in molecular clouds (e.g., Snyder et al., 1985; Schenewerk et al., 1986, 1988; Caux et al., 2011; Frau et al., 2012; Bacmann and Faure, 2016; Spezzano et al., 2017; Rivilla et al., 2019). Its abundance in cold objects is about 10−11 (with respect to H2; e.g., Bacmann and Faure, 2016; Rivilla et al., 2019), where the gaseous HCO+ abundance is lower than about 10−9.
The main formation routes of gaseous HCO are reported in the astrochemical databases KIDA (http://kida.astrophy.u-bordeaux.fr/) and UMIST (http://udfa.ajmarkwick.net/): neutral–neutral reactions, dominated by H2CO + OH (Ocaña et al., 2017), and ionic routes, dominated by the recombination of protonated formaldehyde H2COH+ + e− (Hamberg et al., 2007). When comparing the theoretical predictions of the HCO abundance based on these gas-phase reactions with those measured in cold objects, both Bacmann and Faure (2016) and Rivilla et al. (2019) concluded that the ionic route of the formation of HCO can account for the measured values.
Yet, given the uncertainty in the H2COH+ + e− reaction branching ratio, we verified whether the injection of HCO from the recombination on the grain surfaces could have a non-negligible role. The results of our GRAINOBLE simulations confirm that, even assuming that all the HCO+ recombined on the grain surfaces is released as HCO in the gas, this contribution is at most 30% with respect to that derived using the KIDA reaction rate coefficients. We, therefore, conclude that, in the specific case of HCO+ the neutralization when they meet the charged grains does not substantially affect the abundance of gaseous HCO.
Finally, we would like to mention that this work exclusively focused on an all-water icy grain model, with the aim to be a first step toward studying the influence of a captured electron by an interstellar grain on its chemical properties. Thus, accounting for dirty ices (i.e., including other volatile species such as CO, CO2, NH3, and CH3OH) and ice-processing effects (e.g., cosmic rays' impact or/and UV photon adsorption) on the attached electron fate is beyond the scope of the work. However, we find interesting to mention a plausible scenario not considered in the (1)–(3) processes. It envisages a possible transformation of the negatively charged ice grain, in which OH radicals are also present in the grain, as generated, for instance, by cosmic ray impacts. In this case, the OH radical can scavenge the attached electron to give OH−, as OH− would be more stable than the solvated electron. In this situation, the adsorption of HCO+ does not cause the electron transfer toward HCO+, as the OH− stabilized by the surrounding water environment will attach to HCO+ to give the neutral HCOOH. In the absence of direct neutralization between HCO+ and OH−, HCO+ will interact with a neutral H2O molecule, as investigated by Woon (2011), bringing to the barrierless formation of HCOOH and the transfer of the proton, formerly belonging to the reacted water, toward OH−via a proton-reliance mechanism within the grain water molecules. This scenario is obviously completely different to our (1)–(3) processes. Nevertheless, this alternative route has important consequences in the interstellar grain chemistry because it opens up the possibility of ion–molecule chemistry on grains, traditionally attributed to exclusively occur in the gas phase.
Conclusions
The present work provides reliable evidence based on quantum mechanical calculations that thermalized low-energy electrons can be easily transferred to cationic molecular species upon interaction to form the corresponding radical neutral ones. As a test case, this has been investigated for the interaction of the HCO+ ion with water clusters containing an extra electron. Among the possible situations considered, results indicate that spontaneous formation of the HCO radical by electron transfer is the most favorable one from an energetic point of view. Accordingly, interstellar grain particles can act as reservoir of electrons, which in turn can trigger electron transfer processes, which can be of relevance in the inventory of the interstellar molecules and in the molecular evolution of the chemical diversity of the Universe.
Data Availability Statement
The original contributions presented in the study are included in the article/supplementary material, further inquiries can be directed to the corresponding author.
Author Contributions
All authors participated in the initial conception of the research idea and in the discussion of the results. AR did the quantum chemical calculations, wrote the initial draft of the manuscript, and participated in the editing of the final manuscript. CC did the astrochemical modeling and participated in the writing and editing of the final manuscript. NB revised the initial draft and participated in the editing of the final manuscript. PU participated in the writing and editing of the final manuscript. All authors contributed to the article and approved the submitted version.
Funding
This project has received funding from the European Research Council (ERC) under the European Union's Horizon 2020 research and innovation program (Grant Agreement No. 865657) for the project Quantum Chemistry on Interstellar Grains (QUANTUMGRAIN). The authors acknowledge funding from the European Union's Horizon 2020 research and innovation program, the European Research Council (ERC) Project the Dawn of Organic Chemistry, Grant Agreement No. 741002, and the Marie Skłodowska-Curie project Astro-Chemical Origins (ACO), Grant Agreement No. 811312. MINECO (project CTQ2017-89132-P) and DIUE (project 2017SGR1323). The Italian Space Agency which cofunded the Life in Space Project (ASI N. 2019-3-U.O) is acknowledged for financial support.
Conflict of Interest
The authors declare that the research was conducted in the absence of any commercial or financial relationships that could be construed as a potential conflict of interest.
Acknowledgments
AR is indebted to the Ramón y Cajal program.
References
Agúndez, M., and Wakelam, V. (2013). Chemistry of dark clouds: databases, networks, and models. Chem. Rev. 113, 8710–8737. doi: 10.1021/cr4001176
Alizadeh, E., and Sanche, L. (2012). Precursors of solvated electrons in radiobiological physics and chemistry. Chem. Rev. 112, 5578–5602. doi: 10.1021/cr300063r
Andersson, S., Goumans, T. P. M., and Arnaldsson, A. (2011). Tunneling in hydrogen and deuterium atom addition to CO at low temperatures. Chem. Phys. Lett. 513, 31–36. doi: 10.1016/j.cplett.2011.07.073
Arumainayagam, C. R., Lee, H.-L., Nelson, R. B., Haines, D. R., and Gunawardane, R. P. (2010). Low-energy electron-induced reactions in condensed matter. Surf. Sci. Rep. 65, 1–44. doi: 10.1016/j.surfrep.2009.09.001
Bacmann, A., and Faure, A. (2016). The origin of gas-phase HCO and CH3O radicals in prestellar cores. Astron. Astrophys. 587:A130. doi: 10.1051/0004-6361/201526198
Balucani, N., Ceccarelli, C., and Taquet, V. (2015). Formation of complex organic molecules in cold objects: the role of gas-phase reactions. Mon. Not. R. Astron. Soc. 449, L16–L20. doi: 10.1093/mnrasl/slv009
Boamah, M. D., Sullivan, K. K., Shulenberger, K. E., Soe, C. M., Jacob, L. M., Yhee, F. C., et al. (2014). Low-energy electron-induced chemistry of condensed methanol: implications for the interstellar synthesis of prebiotic molecules. Faraday Discuss. 168, 249–266. doi: 10.1039/C3FD00158J
Caselli, P., and Ceccarelli, C. (2012). Our astrochemical heritage. Astron. Astrophys. Rev. 20, 1–68. doi: 10.1007/s00159-012-0056-x
Caux, E., Kahane, C., Castets, A., Coutens, A., Ceccarelli, C., Bacmann, A., et al. (2011). TIMASSS: the IRAS 16293-2422 millimeter and submillimeter spectral survey. Astron. Astrophys. 532:A23. doi: 10.1051/0004-6361/201015399
Ceccarelli, C., and Dominik, C. (2005). Deuterated in proto-planetary disks. Astron. Astrophys. 440, 583–593. doi: 10.1051/0004-6361:20052991
Chulkov, S. K., Stepanov, N. F., and Novakovskaya, Y. V. (2009). Stationary states and dissociation of H3O radical in water clusters. Russ. J. Phys. Chem. 83, 798–808. doi: 10.1134/S0036024409050203
Draine, B. T., and Sutin, B. (1987). Collisional charging of interstellar grains. Astrophys. J. 320, 803–817. doi: 10.1086/165596
Flower, D. R., and Middleton, M. J. (2004). Electron attachment to interstellar grains. Mon. Not. R. Astron. Soc. 352, 837–840. doi: 10.1111/j.1365-2966.2004.07969.x
Frau, P., Girart, J. M., and Beltrán, M. T. (2012). Chemical differentiation toward the Pipe nebula starless cores. Astron. Astrophys. 537:L9. doi: 10.1051/0004-6361/201118612
Fredon, A., and Cuppen, H. M. (2018). Molecular dynamics simulations of energy dissipation and non-thermal diffusion on amorphous solid water. Phys. Chem. Chem. Phys. 20, 5569–5577. doi: 10.1039/C7CP06136F
Fredon, A., Lamberts, T., and Cuppen, H. M. (2017). Energy dissipation and nonthermal diffusion on interstellar ice grains. Astrophys. J. 849:125. doi: 10.3847/1538-4357/aa8c05
Garrod, R. T., and Herbst, E. (2006). Formation of methyl formate and other organic species in the warm-up phase of hot molecular cores. Astron. Astrophys. 457, 927–936. doi: 10.1051/0004-6361:20065560
Goumans, T. P. M., Catlow, C. R. A., and Brown, W. A. (2008). Hydrogenation of CO on a silica surface: an embedded cluster approach. J. Chem. Phys. 128:134709. doi: 10.1063/1.2888933
Goumans, T. P. M., Wander, A., Catlow, C. R. A., and Brown, W. A. (2007). Silica grain catalysis of methanol formation. Mon. Not. R. Astron. Soc. 382, 1829–1832. doi: 10.1111/j.1365-2966.2007.12491.x
Grassi, T., Bovino, S., Caselli, P., Bovolenta, G., Vogt-Geisse, S., and Ercolano, B. (2020). A novel framework for studying the impact of binding energy distributions on the chemistry of dust grains. Astron. Astrophys. 643:A155. doi: 10.1051/0004-6361/202039087
Hamberg, M., Geppert, W. D., Thomas, R. D., Zhaunerchyk, V., Österdahl, F., Ehlerding, A., et al. (2007). Experimental determination of dissociative recombination reaction pathways and absolute reaction cross-sections of CH2OH+, CD2OD+ and CD2. Mol. Phys. 105, 899–906. doi: 10.1080/00268970701206642
Hatano, Y., Katsumura, Y., and Mozumder, A. (2011). Charged particle and photon interactions with matter. Boca Raton, FL: CRC Press. doi: 10.1201/b10389
Herbert, J. M., and Head-Gordon, M. (2005). Calculation of electron detachment energies for water cluster anions: an appraisal of electronic structure methods, with application to (H2O)20- and (H2O)24. J. Phys. Chem. A 109, 5217–5229. doi: 10.1021/jp051096s
Herbert, J. M., and Head-Gordon, M. (2006). Accuracy and limitations of second-order many-body perturbation theory for predicting vertical detachment energies of solvated-electron clusters. Phys. Chem. Chem. Phys. 8, 68–78. doi: 10.1039/B513098K
Khan, A. (2003). Solvated electron in and clusters: a theoretical study. J. Chem. Phys. 118, 1684–1687. doi: 10.1063/1.1531100
Khan, A. (2004). Extra electron in cluster isomers: a theoretical study. J. Chem. Phys. 121, 280–284. doi: 10.1063/1.1758692
Khan, A. (2005). Solvated electron in dodecahedral cavity: calculated stretch frequencies and vertical dissociation energy. Chem. Phys. Lett. 401, 85–88. doi: 10.1016/j.cplett.2004.11.035
Khan, A. (2006). Ab initio studies on clusters: existence of surface and interior-bound extra electrons. J. Chem. Phys. 125:024307. doi: 10.1063/1.2216705
Kim, J., Park, J. M., Oh, K. S., Lee, J. Y., Lee, S., and Kim, K. S. (1997). Structure, vertical electron-detachment energy, and O–H stretching frequencies of e+(H2O)12. J. Chem. Phys. 106, 10207–10214. doi: 10.1063/1.474106
Kim, K. S., Park, I., Lee, S., Cho, K., Lee, J. Y., Kim, J., et al. (1996). The nature of a wet electron. Phys. Rev. Lett. 76, 956–959. doi: 10.1103/PhysRevLett.76.956
Lee, S., Kim, J., Lee, S. J., and Kim, K. S. (1997). Novel structures for the excess electron state of the water hexamer and the interaction forces governing the structures. Phys. Rev. Lett. 79, 2038–2041. doi: 10.1103/PhysRevLett.79.2038
Loison, J.-C., Wakelam, V., Gratier, P., Hickson, K. M., Bacmann, A., Agùndez, M., et al. (2019). Oxygen fractionation in dense molecular clouds. Mon. Not. R. Astron. Soc. 485, 5777–5789. doi: 10.1093/mnras/stz560
Marenich, A. V., and Boggs, J. E. (2003). Coupled cluster CCSD(T) calculations of equilibrium geometries, anharmonic force fields, and thermodynamic properties of the formyl (HCO) and isoformyl (COH) radical species. J. Phys. Chem. A 107, 2343–2350. doi: 10.1021/jp0223298
Mason, N. J., and Field, D. (2019). “Electrons in space: the final (and first) frontier,” in Low-Energy Electrons. Fundamentals and Applications, Chapter 8, ed O. Ingólfsson (Boca Raton, FL: Jenny Stanford Publishing). 371–402. doi: 10.1201/9780429058820-8
Mason, N. J., Nair, B., Jheeta, S., and Szymanska, E. (2014). Electron induced chemistry: a new frontier in astrochemistry. Faraday Discuss. 168, 235–247. doi: 10.1039/C4FD00004H
Ocaña, A. J., Jiménez, E., Ballesteros, B., Canosa, A., Antiñolo, M., Albaladejo, J., et al. (2017). Is the gas-phase OH+H2CO reaction a source of HCO in interstellar cold dark clouds? A kinetic, dynamic, and modeling study. Astrophys. J. 850:28. doi: 10.3847/1538-4357/aa93d9
Pantaleone, S., Enrique-Romero, J., Ceccarelli, C., Ugliengo, P., Balucani, N., and Rimola, A. (2020). Chemical desorption versus energy dissipation: insights from ab initio molecular dynamics of HCO· formation. Astrophys. J. 897:56. doi: 10.3847/1538-4357/ab8a4b
Penteado, E. M., Walsh, C., and Cuppen, H. M. (2017). Sensitivity analysis of grain surface chemistry to binding energies of ice species. Astrophys. J. 844:71. doi: 10.3847/1538-4357/aa78f9
Peters, P. S., Duflot, D., Wiesenfeld, L., and Toubin, C. (2013). The H + CO ⇌ HCO reaction studied by ab initio benchmark calculations. J. Chem. Phys. 139:164310. doi: 10.1063/1.4826171
Pimblott, S. M., and Laverne, J. A. (2007). Production of low-energy electrons by ionizing radiation. Radiat. Phys. Chem. 76, 1244–1247. doi: 10.1016/j.radphyschem.2007.02.012
Prigogine, I., and Rice, S. A. (1973). Advances in chemical physics. New York, NY: Wiley. doi: 10.1002/9780470143735
Rae, J. G. L., Hartquist, T. W., Lepp, S. H., O'neill, P. T., and Williams, D. A. (2004). Grain photoelectric ionisation rates, heating rates, and charge as functions of visual extinction. Astron. Astrophys. 413, 1–5. doi: 10.1051/0004-6361:20031505
Rimola, A., Taquet, V., Ugliengo, P., Balucani, N., and Ceccarelli, C. (2014). Combined quantum chemical and modeling study of CO hydrogenation on water ice. Astron. Astrophys. 572:A70. doi: 10.1051/0004-6361/201424046
Rivilla, V. M., Beltrán, M. T., Vasyunin, A., Caselli, P., Viti, S., Fontani, F., et al. (2019). First ALMA maps of HCO, an important precursor of complex organic molecules, towards IRAS 16293–2422. Mon. Not. R. Astron. Soc. 483, 806–823. doi: 10.1093/mnras/sty3078
Schenewerk, M. S., Snyder, L. E., and Hjalmarson, A. (1986). Interstellar HCO - Detection of the missing 3 millimeter quartet. Astrophys. J. 303, L71–L74. doi: 10.1086/184655
Schenewerk, M. S., Snyder, L. E., Hollis, J. M., Jewell, P. R., and Ziurys, L. M. (1988). HCO emission from H ii–molecular cloud interface regions. Astrophys. J. 328:785. doi: 10.1086/166337
Shannon, R. J., Blitz, M. A., Goddard, A., and Heard, D. E. (2013). Accelerated chemistry in the reaction between the hydroxyl radical and methanol at interstellar temperatures facilitated by tunnelling. Nat. Chem. 5, 745–749. doi: 10.1038/nchem.1692
Skouteris, D., Balucani, N., Ceccarelli, C., Faginas lago, N., Codella, C., Falcinelli, S., et al. (2019). Interstellar dimethyl ether gas-phase formation: a quantum chemistry and kinetics study. Mon. Not. R. Astron. Soc. 482, 3567–3575. doi: 10.1093/mnras/sty2903
Skouteris, D., Balucani, N., Ceccarelli, C., Vazart, F., Puzzarini, C., Barone, V., et al. (2018). The genealogical tree of ethanol: gas-phase formation of glycolaldehyde, acetic acid, and formic acid. Astrophys. J. 854:135. doi: 10.3847/1538-4357/aaa41e
Skouteris, D., Vazart, F., Ceccarelli, C., Balucani, N., Puzzarini, C., and Barone, V. (2017). New quantum chemical computations of formamide deuteration support gas-phase formation of this prebiotic molecule. Mon. Not. R. Astron. Soc. 468, L1–L5. doi: 10.1093/mnrasl/slx012
Snyder, L. E., Hollis, J. M., and Ulich, B. L. (1976). Radio detection of the interstellar formyl radical. Astrophys. J. 208, L91–L94. doi: 10.1086/182239
Snyder, L. E., Schenewerk, M. S., and Hollis, J. M. (1985). Observations of several new transitions of interstellar HCO. Astrophys. J. 298, 360–368. doi: 10.1086/163618
Spezzano, S., Caselli, P., Bizzocchi, L., Giuliano, B. M., and Lattanzi, V. (2017). The observed chemical structure of L1544. Astron. Astrophys. 606:A82. doi: 10.1051/0004-6361/201731262
Taquet, V., Ceccarelli, C., and Kahane, C. (2012). Multilayer modeling of porous grain surface chemistry. Astron. Astrophys. 538:A42. doi: 10.1051/0004-6361/201117802
Taquet, V., Peters, P. S., Kahane, C., Ceccarelli, C., López-Sepulcre, A., Toubin, C., et al. (2013). Water ice deuteration: a tracer of the chemical history of protostars. Astron. Astrophys. 550:A127. doi: 10.1051/0004-6361/201220084
Thrower, J. D., Collings, M. P., Rutten, F. J. M., and Mccoustra, M. R. S. (2011). Highly efficient electron-stimulated desorption of benzene from amorphous solid water ice. Chem. Phys. Lett. 505, 106–111. doi: 10.1016/j.cplett.2011.02.029
Tielens, A. G. G. M. (2013). The molecular universe. Rev. Mod. Phys. 85, 1021–1081. doi: 10.1103/RevModPhys.85.1021
Turi, L., and Rossky, P. J. (2012). Theoretical studies of spectroscopy and dynamics of hydrated electrons. Chem. Rev. 112, 5641–5674. doi: 10.1021/cr300144z
Van Dishoeck, E. F. (2014). Astrochemistry of dust, ice and gas: introduction and overview. Faraday Discuss. 168, 9–47. doi: 10.1039/C4FD00140K
Vasyunin, A. I., and Herbst, E. (2013). Reactive desorption and radiative association as possible drivers of complex molecule formation in the cold interstellar medium. Astrophys. J. 769:34. doi: 10.1088/0004-637X/769/1/34
Vazart, F., Ceccarelli, C., Balucani, N., Bianchi, E., and Skouteris, D. (2020). Gas-phase formation of acetaldehyde: review and new theoretical computations. Mon. Not. R. Astron. Soc. 499, 5547–5561. doi: 10.1093/mnras/staa3060
Vidali, G. (2013). H2 formation on interstellar grains. Chem. Rev. 113, 8762–8782. doi: 10.1021/cr400156b
Wakelam, V., Bron, E., Cazaux, S., Dulieu, F., Gry, C., Guillard, P., et al. (2017). H2 formation on interstellar dust grains: the viewpoints of theory, experiments, models and observations. Mol. Astrophys. 9, 1–36. doi: 10.1016/j.molap.2017.11.001
Walmsley, C. M., Flower, D. R., and Forêts, G. P. D. (2004). Complete depletion in prestellar cores. Astron. Astrophys. 418, 1035–1043. doi: 10.1051/0004-6361:20035718
Woon, D. E. (2002). Modeling gas-grain chemistry with quantum chemical cluster calculations. I. Heterogeneous hydrogenation of CO and H(2)CO on icy grain mantles. Astrophys. J. 569, 541–548. doi: 10.1086/339279
Woon, D. E. (2011). Ion-ice astrochemistry: barrierless low-energy deposition pathways to HCOOH, CH3OH, and CO2 on icy grain mantles from precursor cations. Astrophys. J. 728:44. doi: 10.1088/0004-637X/728/1/44
Keywords: DFT, water ice, solvated electron, astrochemical modeling, interstellar medium
Citation: Rimola A, Ceccarelli C, Balucani N and Ugliengo P (2021) Interaction of HCO+ Cations With Interstellar Negative Grains. Quantum Chemical Investigation and Astrophysical Implications. Front. Astron. Space Sci. 8:655405. doi: 10.3389/fspas.2021.655405
Received: 18 January 2021; Accepted: 05 March 2021;
Published: 29 April 2021.
Edited by:
Majdi Hochlaf, Université Paris Est Marne la Vallée, FranceReviewed by:
David Woon, University of Illinois at Urbana–Champaign, United StatesAlexis Markovits, Sorbonne Universités, France
Copyright © 2021 Rimola, Ceccarelli, Balucani and Ugliengo. This is an open-access article distributed under the terms of the Creative Commons Attribution License (CC BY). The use, distribution or reproduction in other forums is permitted, provided the original author(s) and the copyright owner(s) are credited and that the original publication in this journal is cited, in accordance with accepted academic practice. No use, distribution or reproduction is permitted which does not comply with these terms.
*Correspondence: Albert Rimola, YWxiZXJ0LnJpbW9sYUB1YWIuY2F0