- 1School of Physics and Astronomy, University of Edinburgh, Edinburgh, United Kingdom
- 2Boulby Underground Laboratory, Saltburn-by-the-Sea, United Kingdom
- 3Trinity College, Oxford University, Oxford, United Kingdom
The effects of high radiation as a biological extreme have historically been, and continue to be, extensively researched in the fields of radiation biology and astrobiology. However, the absence of radiation as an extreme has received relatively limited attention from the scientific community, with its effects on life remaining unclear. The currently accepted model of the radiation dose-damage relationship for organisms is the linear no-threshold (LNT) model, which predicts a positive linear correlation between dose and damage that intercepts at zero dose corresponding to zero damage. Despite its wide-spread implementation, the LNT model is continuously being challenged by various new models, with the hormesis model as one of its main competitors. This model also postulates damage at high doses but, in contrast to the LNT model, it predicts beneficial stimulation of growth at low doses. Experiments to date have not yet been able to conclusively validate or dismiss either of these models. The aim of the collaborative Subsurface Experiment of Life in Low Radiation (SELLR) project was to test these competing models on prokaryotes in a well-characterised environment and provide a robust experimental set up to investigate low radiation in terrestrial and non-terrestrial environments. Bacterial growth assays using Bacillus subtilis and Escherichia coli were performed under ultra low ionising radiation in the Boulby International Subsurface Astrobiology Laboratory (BISAL) facilities of the Boulby Underground Laboratory at Boulby mine (Redcar & Cleveland, UK) and were used to investigate effects on viability and signs of preconditioning. No significant effect on bacterial growth was observed from exposure to radiation doses ranging from 0.01 times the levels of background radiation typically found in terrestrial surface environments to 100 times that background. Additionally, no preconditioned susceptibility to stress was observed in the bacterial strains grown in sustained low radiation. These data suggest that the extremes of low radiation do not alter growth parameters of these two organisms and that an improved model should be considered for prokaryotes, consisting of a dose-damage response with a threshold at ultra low radiation. We discuss the implications of these data for low radiation as a novel microbiological “extreme.”
Introduction
Radiation biology research is mostly conducted in the context of effects of, and protection from, high radiation exposure such as from radioactive fallout and medical treatments (e.g., de González and Darby, 2004; Preston et al., 2004; Finch, 2007). In this regard, the field of Astrobiology is no exception with a seemingly exclusive focus on the effects of the high radiation conditions of low Earth orbit, interplanetary space, and other planetary environments on organisms (e.g., Horneck, 1992; Moeller et al., 2017; de Vera et al., 2019). The historical and continuing scientific focus on high radiation is by no means unfounded, as the connection between irradiation from an ionising or an energetic radiation source and biological damage, resulting in mutations or cell death, is well-established (Lorenz, 1944; Wanebo et al., 1968; Chang et al., 1985; Ward, 1988). However, extreme opposites of physical variables exist and, to use the title of Richard Feynman's (1959) address to the annual American Physical Society meeting at Caltech (Feynman, 1960), there's “plenty of room at the bottom” of the radiation dose range to explore as a physical extreme. In order to build a holistic understanding of how environmental parameters shape the viability potential of life on Earth and, by extension, on other planetary bodies, all parameter extremes should be examined.
All life on Earth is constantly being subjected to a low dose of naturally occurring radiation in various forms. For example, non-ionising ultraviolet (UV) light (UVA = 315–400 nm, UVB = 280–315 nm) from the sun can pass through the Earth's atmosphere and reach us, sometimes causing skin melanomas (International Agency for Research on Cancer, 1992). Of the naturally occurring radiation, ionising radiation is of particular significance due to its increased potential for causing biological damage (e.g., Prise et al., 2001). Rock radionuclides from various long-lived radioisotopes, such as uranium, thorium and potassium, and cosmic rays are sources of natural ionising radiation (Hamilton, 1989; Shahbazi-Gahrouei et al., 2013). Moreover, airborne radon and its decay radionuclides contribute to more than half the total average worldwide dose rate of naturally occurring radiation of ~2.8 mGy/y. With additional man-made radiation, the mean global surface radiation dose rate is estimated to be ~3.01 mGy/y (United Nations, 2008), although this can vary locally e.g., 0.6 mGy/y measured at the Boulby mine surface to 260 mGy/y in Ramsar, Iran (Ghiassi-Nejad et al., 2002). Usually, doses/dose rates below the global or local background dose average are referred to as “low” or “ultra low” doses in low radiation studies.
There are currently three main ideas on how a low radiation environment might influence an organism's viability: (1) Any amount of radiation, however small, will negatively impact viability and continue to do so in a linear manner (“linear no-threshold” model), (2) A hyperbolic dose-damage response to low radiation in the form of either hypersensitivity or positive stimulation (the latter being the “hormesis” model), which would be a unique property among extreme conditions. In the hormesis model, low levels of ionising radiation are therefore beneficial for life, and (3) At some low level of radiation there is no effect on life, with a threshold below which there is no discernible effect on cellular physiology or growth parameters. However, above the determined threshold the radiation dose-damage continues in a linear manner. This is known as the “threshold” model (e.g., Mancuso et al., 2012; Siasou et al., 2017; Figure 1). Since the mid-twentieth century, the generally accepted model for describing an organism's radiation dose-response relationship has been the linear no-threshold (LNT) model (Muller, 1946; Figure 1), which was further supported by the National Research Council (NRC) (2006). The LNT model is largely based on studies in above-background radiation conditions, i.e., high radiation exposure. Radiation-induced mutations in rats in the early twentieth century (Olson and Lewis, 1928), and subsequent research on fruit fly exposure to high radiation doses (103 times background dose) served as the basis for development of the LNT model (Muller, 1954). However, subsequent studies have indicated that when dealing with lower doses, the LNT model may not be applicable (e.g., Calabrese and Baldwin, 2003; Feinendegen et al., 2007; Smith et al., 2011; Shamoun, 2016; Shibamoto and Nakamura, 2018; Costantini and Borremans, 2019).
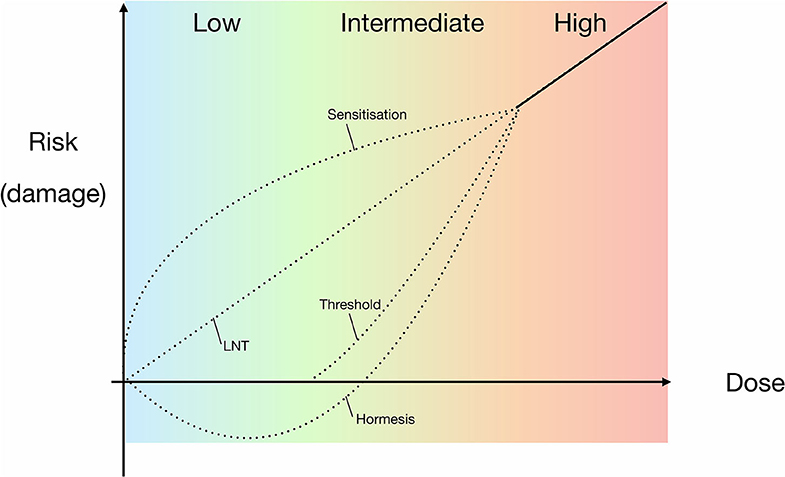
Figure 1. Proposed models for experimental and theoretical correlations of radiation dose and the risk of damage to organisms, including the widely accepted LNT model. Models are based on high-dose data, extrapolating responses for lower dose ranges. High doses are defined as those causing observable, serious or irreparable damage to an organism. The dotted lines indicate the potential models that may be applicable to prokaryotes in the intermediate and low radiation does ranges, i.e., either side of background radiation up until high doses. LNT = linear relationship between dose and risk of damage to an organism; sensitivity = a heightened risk of damage at lower doses; hormesis = high dose inhibition with low dose stimulation; threshold = no/negligible risk of damage below a specific dose.
Despite the long-standing application of the LNT model, it is strongly contested in the literature by proponents of the hormesis (hormetic) model (Figure 1), who argue that the LNT model is not representative of the cellular response to lower radiation doses and dose rates (e.g., Feinendegen et al., 2007). The principle of hormesis is based on a low dose stimulatory effect of a physical or chemical component that, at high doses, has an inhibitory effect (Lorenz, 1950; Stebbing, 1982; Mine et al., 1990). Additional, less prevalent hypotheses include the “threshold” and “sensitization/hypersensitivity” models, with the former proposing a lack of impact below a certain dose range and the latter serving as an opposite to hormesis, implying an increased negative effect on viability at low dose exposure (Figure 1). Although it is reasonable to presume the LNT model is also applicable to prokaryotes at higher doses of radiation, there is still no definitive consensus within the scientific community on the effects of low radiation exposure on microbes (Figure 1).
Here we present data from the Subsurface Experiment of Life in Low Radiation (SELLR) project on the effects of below background radiation on the growth of Bacillus subtilis and Escherichia coli. We also describe a robust experimental set-up that allows for the study of prokaryotic biology at below background levels of radiation using an underground astrobiology laboratory. The aim of the Subsurface Experiment of Life in Low Radiation (SELLR) project, described in this work, is to provide a well-controlled and characterised experimental set up with minimal variables in which comparable bacterial growth experiments can be carried out. The robust set up establishes a baseline study for future work simulating low radiation (planetary) environments.
Materials and Methods
Previous low radiation experiments (i.e., below the local terrestrial surface radiation doses) have been carried out in various facilities by research groups examining a variety of cells and with different experimental set-ups. Furthermore, when establishing and working in a low radiation experimental set up there are additional factors to those of standard experiments to consider. This and the lack of a universal, gold-standard low radiation set up has continued to impede the comparability of data in low radiation literature. Table 1 illustrates some of the potential experimental difficulties in a low radiation set up and the experimental design adjustments that were adopted for the SELLR project to avoid the outlined issues.
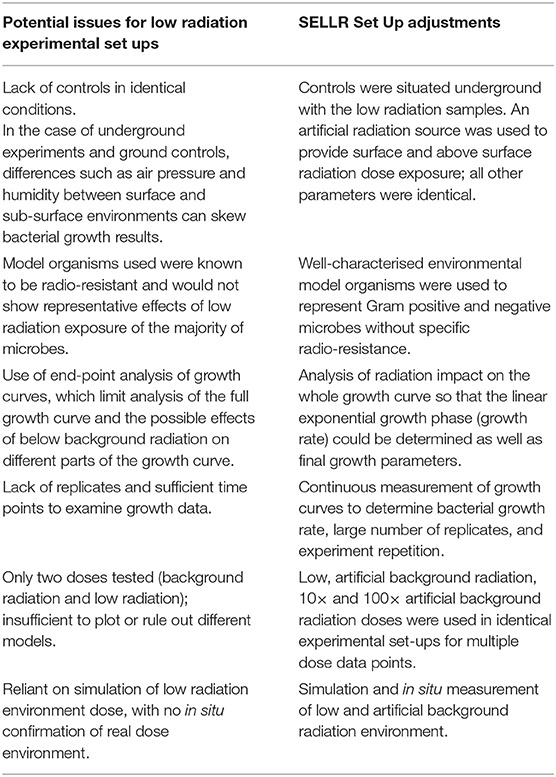
Table 1. Potential problems facing low radiation experiments and the respective adjustments made for the SELLR experimental set up.
Experimental Overview
Two prokaryotic model organisms were grown in the low radiation, underground experimental set-up that consisted of two identical plate readers housed in a lead “castle" to further reduce radiation exposure. Within the castle, the plate readers were separated by a lead brick wall, such that one half of the castle provided the ultra low radiation environment whilst the other half provided an artificially created background radiation environment mimicking that of the local surface, established using a 137Cs source (Figure 2). The model organisms were then grown in the plate readers for varying lengths of time in the low radiation environment and the artificial background radiation environment, which served as the control. After growth in either radiation condition, the organisms were briefly exposed to UVC to test potential differences in stress response.
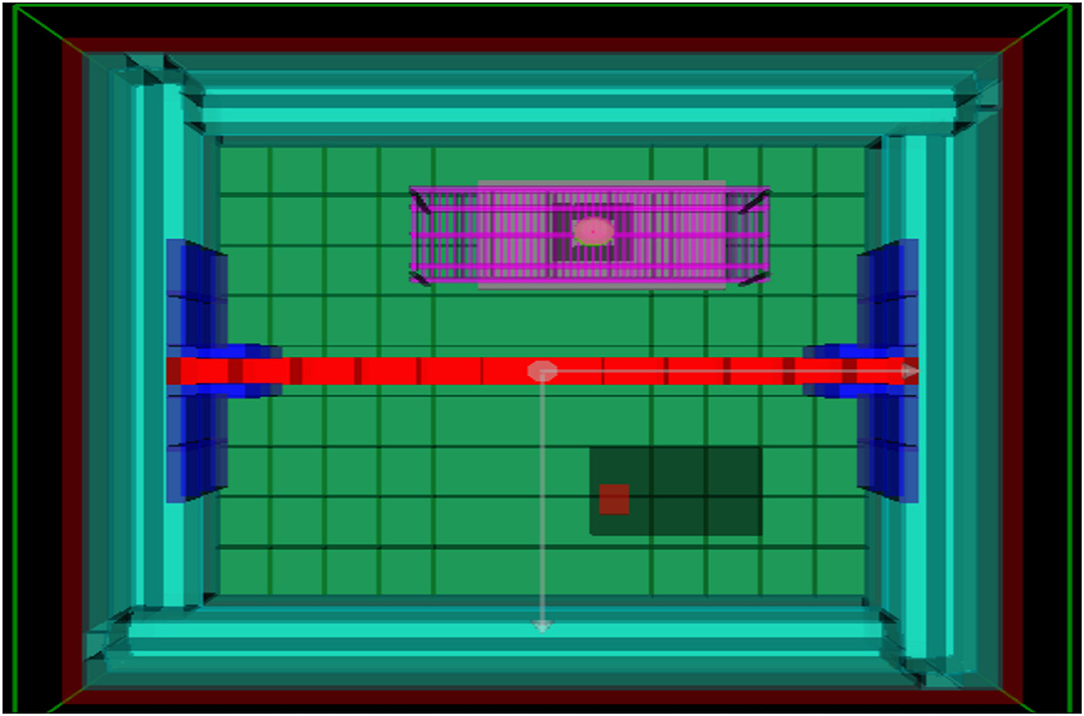
Figure 2. Geometry of lead-lined chamber in GEANT4, view from above. The lead wall is marked in red, separating the container into a low and artificial background radiation environment. The 137Cs γ-source is located on the purple platform above the one plate reader, providing the artificial background radiation.
Location and Radiation Set-Up
Unless specifically stated, all experiments were carried out in the Boulby Underground Laboratory at the Boulby Mine, Redcar & Cleveland UK. At 1.1 km depth, the rock accounts for a cosmic ray attenuation by a factor of 106 (Reichhart et al., 2013). Any changes caused by the attenuation to the cosmic ray energy spectrum are assumed to be irrelevant as the cosmic rays are of high enough energies to be minimally ionising, such that energy deposition by muons and secondary neutron spallation rates will scale linearly with absolute cosmic ray flux.
With the cosmic ray flux minimised, the Boulby Laboratory is particularly well-suited to provide radiation protection as the local salt has low natural background radiation, with low levels of γ-ray and neutron emission. Moreover, the salt and general geographical area of Boulby have a low rate of radon production, resulting in an airborne background level of 2.4 Bq/m3 (Araújo et al., 2012). To establish an ultra low radiation environment, the experiments were additionally encased in a radon-free lead-lined container (castle). This resulted in a 100-fold reduction of the average local ionising surface radiation dose rate (see the following method sections for details on the radiation environment). To reduce systematic uncertainties, measurements were performed in two experimental stations, formed by partitioning the lead-lined container in to two equal halves, separated by a 10 cm-thick wall formed of low-radioactivity lead bricks. Identical SPECTROstar Nano plate readers (BMG Labtech) were then positioned in each half of the lead-lined box (Figure 2).
To achieve elevated (above low background radiation doses), a 137Cs source was placed above one of the plate readers to artificially generate surface radiation doses, and up to 100 times higher, while with no such source, the samples in the other plate reader provided well-defined controls. The 137Cs source was used to approximate ionising radiation biological damage on the surface, recognizing that it is difficult to artificially reconstruct the complete spectrum of natural radiation of the surface in a confined subsurface laboratory environment. The temperature of both plate readers was internally controlled, the outside temperature was constant at 22°C (±1°C) and 82% relative humidity (± 2%).
Artificial Background and Low Radiation Environments
Theoretical Dose Rates
Both the low dose and control samples were located in the same sub-surface experimental set up (described in section Location and Radiation Set-Up) to avoid differences in environmental conditions.
Control samples were exposed to a 15.5 MBq (± 0.1 MBq) 137Cs γ-source to approximate a natural surface background radiation dose rate. The collimated but unattenuated source provided a dose rate of = 27.8 mGy/y (± 0.506 mGy/y) at a 59 cm height from the top of the 96-well plate, which was lowered to 1 mGy/y by placing lead sheets between the source and samples. It was calculated that 11 lead sheets (3.3 cm total thickness) would be required to attenuate radiation from the 137Cs γ-source to obtain a final dose rate comparable to that measured at the local surface (~0.18–0.6 mGy/y).
To generate higher dose rates D(r) of 10 × and 100 × background, the inverse square rule is applied.
As the distance r between source and sample is decreased, the difference in local dose rates across the plate becomes more significant, i.e., as the source is moved closer to the samples there is a larger discrepancy between the dose rate of the centre of the plate compared to that of the periphery. It was calculated that for our maximum dose rate used (100 mGy/y) the maximum difference in dose rate across the plate would be 3.3 mGy/y.
Measured Dose Rates
A series of measurements were taken to establish the in situ dose rates of the artificial background radiation and low radiation environments. The measurements were taken with a NaI detector (InSpector 1000 with an IRPOS-2: Stabilised 2” × 2” probe), which gives real time values with a minimum dose rate equivalent of 10 nSv/h (0.088 mSv/y). Measurements of the unattenuated source yielded an absorbed dose rate of (0) = 29.9 mGy/y ± 0.5 mGy/y and the 11-lead sheet-shielded dose rate of (3.3 cm lead) = 1.067 mGy/y ± 0.2 mGy/y. The higher measured value than the theoretical value for the unattenuated source dose rate is consistent with measurement errors and backscattering of γ-rays from the walls of the lead castle. The measured dose rate within the castle with the lid closed and without the source was 0.0044 mGy/y, a reduction to ~1% natural background radiation. The measured local surface dose rates at Boulby ranged from 0.18 to 0.6 mGy/y. These were measured once at the beginning of the SELLR project with the same detector as stated above.
Intrinsic Radiation From Experimental Apparatus
Due to the need to ensure an ultra low radiation environment, the internal intrinsic radiation of both the equipment and the samples had to be established to quantify the contribution to the final radiation dose. The radioactivity of the components used in this experiment was determined using γ-ray spectroscopy at the Boulby Underground Germanium Suite (BUGS). BUGS operates several low-background high purity germanium detectors in the Boulby Underground Laboratory (Scovell et al., 2018). This technique measures γ-ray emission associated with the decay of radioisotopes (usually U/Th/K along with anthropogenic radioisotopes) found in all materials and uses the detected flux to calculate a specific activity for each (Gilmore, 2011). This technique is widely used in material radio-assay for low-background particle physics experiments (Araújo et al., 2012; Abgrall et al., 2016; Aprile et al., 2017) where even small contaminations can severely impact detector sensitivity to rare event searches. For SELLR, the plate readers, plates and 500 mL of bacterial growth media (with and without bacteria) were assayed. Each sample was placed on a germanium detector for ~1 week. From this, it was possible to determine that the potassium activity of all the constituent parts was substantially sub-dominant to the potassium activity of the bacteria. Table 2 shows the bacterial radiation contribution.
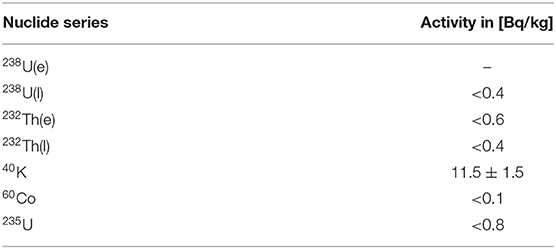
Table 2. Dose contribution of nuclide series in the bacterial sample (growth media + B. subtilis inoculate).
The highest internal nuclide activity from the bacterial sample was potassium (40K), which undergoes β-decay (1,311 keV end point energy with an 89% branching ratio) and electron capture (10.7% branching ratio, almost always followed by a 1,461 keV γ-ray emitted from the 40Ar daughter). Values of other nuclides are upper limits as they are below the sensitivity of the detector. The expected dose rate due to potassium is:
and the expected maximum absorbed dose rate due to other nuclides is given by:
Although the expected dose rate of nuclides (excluding potassium) would exceed the levels necessary for the low radiation experiments, these values are the expected maximum calculated at 90% confidence level. The true values—and, by extension the true dose rate—are therefore highly likely to be below the maxima shown in Table 2 and below the expected dose rate. To further constrain the actual experimental radiation environment, additional simulations, and in situ measurements were also carried out (see sections Artificial Background and Low Radiation Environments and Radiation Simulation).
Radiation Simulation
To understand the radiation environment in the lead-lined castle, the entire experimental set up was constructed in GEANT4, and known radiation sources were added to simulate the resulting radiation. GEANT4 is a toolkit using Monte Carlo methods for simulating the passage of particles through matter (Agostinelli et al., 2003). This simulation was performed to provide insight into possible daughter particle radiation that must be taken into consideration when establishing the artificial background and low radiation environments. Radiation from a variety of sources was simulated, including the 137Cs source, radon from the air, activities in the surrounding bedrock, and lead in the castle. Uranium and thorium decay chains were assumed to be in secular equilibrium. It was concluded that γ-rays from the decay chains of uranium-thorium-potassium contamination in lead and the decay products of airborne radon could result in a ~0.1 mGy/y contribution to the radiation background in both halves of the castle. However, due to the higher dose contribution of the growth medium (see section Intrinsic Radiation From Experimental Apparatus) the contribution of the airborne radon was discounted. Additionally, the simulation showed the 137Cs source used to produce the artificial radiation environment resulted in an additional dose rate of 314 nGy/year in the shielded, low radiation part of the castle. This value is however low enough to not significantly contribute to the low radiation environment.
Model Organism Selection
Bacillus subtilis (strain 168, DM 402) and Escherichia coli (MG 1655) were chosen as model organisms to test the effect of low radiation doses on microbial growth. Both microbes are well-characterised environmental bacteria that can be cultured in the laboratory and were chosen to represent Gram positive and negative bacteria, respectively. Both have approximately the same, short doubling time of ~20 min, which allows for an efficient collection of multiple growth curves. Neither is classed as radio-resistant. We deliberately chose these characteristics to maximise the likelihood of detecting a response to the low radiation doses.
Cell Growth Set-Up
Bacillus subtilis and E. coli were grown in the artificial background and low radiation environments for 24 and 48 h. In some experiments, the dose rate was increased to 10 × and 100 × artificial surface background radiation by adjusting the distance of the 137Cs source to the samples. The gradient of the bacterial exponential growth phase (growth rate) was determined, to establish whether either radiation environment had an effect on the bacterial growth. In addition to affecting growth rate, previous research (Satta et al., 1995) has claimed that prolonged exposure to a low radiation environment leaves cells more sensitive to stress factors, such as toxins or exposure to brief increases in radiation. To investigate this hypothesis, B. subtilis and E. coli were subjected to brief UVC radiation exposure after growth in the different radiation environments (see section UV Radiation Exposure).
Bacterial Growth Experiments
Because of the limited access to the mine, the growth experiments were performed in blocks of 5–6 days at a time. The terminology of “week 1, 2.” refers to the week in which the set of experiments was carried out, for ease of comparison (see Table 3 for experimental nomenclature). To obtain aliquots of bacteria for the experiments, monocultures of B. subtilis and E. coli were grown at 37°C before being frozen into 25% glycerol 1 mL-aliquots and stored at −80°C at the University of Edinburgh. To obtain overnight cultures for experiments, 40 mL of nutrient broth was inoculated with 10 μL of thawed aliquots and cultured overnight at 37°C before use in the experiments. This was done at the overground laboratory at Boulby. The culture was then brought down to the underground laboratory at the beginning of each experimental run. At the beginning of each growth experiment, 5 μL of culture was added to 84 wells of a 96-well plate containing 200 μL of fresh nutrient broth. The last row of the plate contained only nutrient broth to serve as a blank for the plate reader. B. subtilis was grown at 30°C and E. coli at 37°C, respectively, for 24 h, with optical density (OD600) measurements being taken every 20 min to coincide with both bacterial doubling times. For the 48 h experiments, 5 μL of the 24 h culture was added to 84 wells of a 96-well plate containing 200 μL of fresh nutrient broth and grown for 24 h under the same conditions as it was in the previous 24 h. The reason for having both 24 and 48 h growth data was to determine whether an extended period of exposure to the low radiation environment accentuated any observed responses in bacterial growth. This also gave us information on an increased number of generations, with 48 h resulting in ~144 generations for both B. subtilis and E. coli.
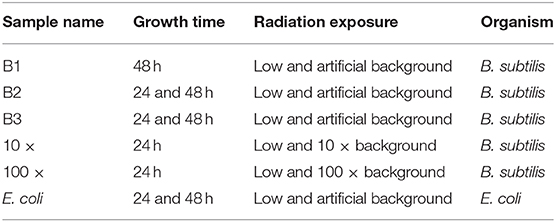
Table 3. SELLR experiment nomenclature for B. subtilis replicate experiments and E. coli experiments indicating growth time, radiation exposure, and the week of experimental execution; art., artificial.
UV Radiation Exposure
In order to assess whether the microbes' ability to cope with stress was influenced by growth in the various radiation environments, cells were exposed to a short pulse of UVC and their viability was assessed by plating on Oxoid (LP0011) nutrient agar. Immediately after being grown for the specified amount of time in the respective radiation environments, the plates were irradiated with a monochromatic UVC lamp (λ = 254 nm; I = 11.2 W/m2; formally UVP Company now Analytik Jena AG) at distance of 5 cm for 30 s. Triplicates were selected from random wells of each condition and serially diluted by a factor of 106. One hundred microliters of each triplicate was plated in triplicate on nutrient agar and cultured overnight at 37°C. All steps of the UVC exposure and cultivation were conducted underground.
Experimental Nomenclature
Three replicate low and artificial background radiation experiments were run with B. subtilis. For clarity when presenting these results, each set of experiments will be referred to with the nomenclature in Table 3 to differentiate between experimental runs. Whether the data is from the low or artificial background environment, and whether it is from the 24 or 48 h growth curves, will be stated in the text and figures. Henceforth, the one half of the experimental set up with 1% background radiation environment will be referred to as the “low” condition. The experimental set up involving enhanced radiation (background, 10 × and 100 × background radiation) containing the 137Cs source will henceforth be referred to as the “artificial background” condition.
Analysis
All plate reader experiments were performed using 84 replicates; UV experiments were performed in triplicate with each triplicate plated on three agar plates. Numbers in figures show averages of replicates, error bars show standard error (s.e.) among replicates. Gradients of the exponential growth phases were established by an automated, linearly-fit trendline in excel. Error of gradients was calculated using the LINEST function in excel. Growth rates were calculated with the equation:
which when converted to the decadic logarithm becomes:
OD1 and OD2 as well as respective t1 and t2 were determined in each growth curve as the beginning of the exponential growth phase. Statistical analysis was performed using one-way ANOVA and two-tailed unpaired equal variance Student's t-tests, where p < 0.05 was considered significant. Analysis of the bacterial growth curves focused on the linear exponential growth phases, which were most comparable and were used as a proxy for the relative fitness of each sample. When a non-linear “shoulder” in the exponential growth phase was observed, only data from the primary linear phase was used to calculate gradients.
Results
Overview of Bacterial Growth in Low and Artificial Background Radiation
Variations in growth were observed between experimental runs, but not between the differing radiation conditions.
All B. subtilis samples progressed through the lag, exponential and stationary growth phases. The upper cluster of final yield data in Figure 3 contained data points from the 48 h B1 data, 24 and 48 h B2 data, as well as the B3 24 h data, whilst the lower cluster contained all B3 48 h data points. Each growth curve showed the presence of a “shoulder” in the exponential growth phase approximately between 480 and 600 min into growth after which all experimental runs, except the B3 48 h artificial background, progressed to a secondary exponential phase.
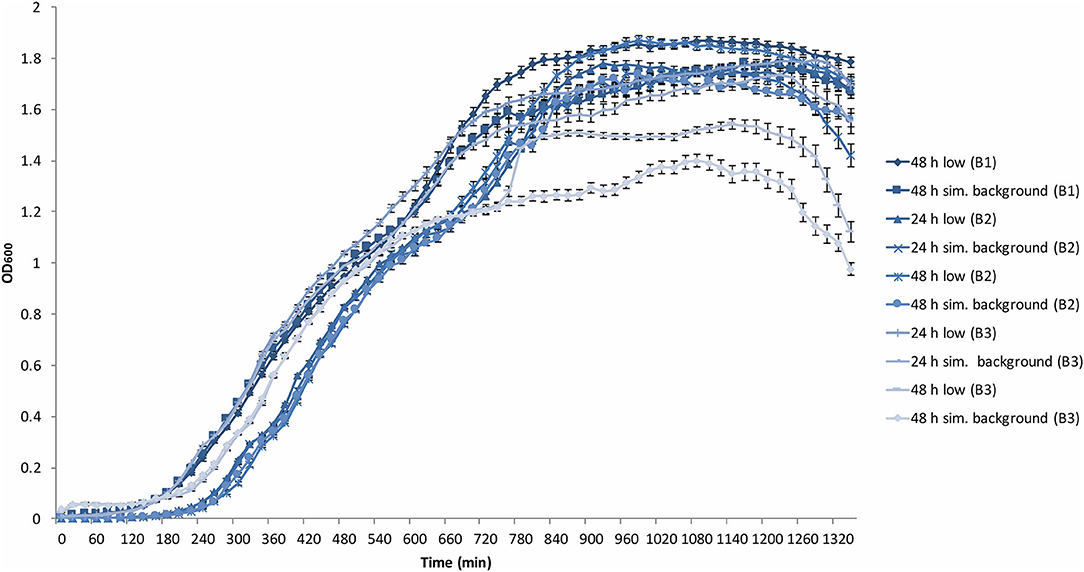
Figure 3. Average (n = 84) of B. subtilis growth curves at the determined low radiation (low) and artificial background radiation (“sim. background”) dose rate: 24 and 48 h denote time in hours that bacteria batch has spent in respective conditions; B1-3 = experiment run number. Error bars show ± standard error (n = 84).
The stationary phase was reached with varying ODs from 1.5 to 1.8 with the exceptions of both B3 low and artificial background samples that reached between 1.2 and 1.5.
The last 2 h of the plate reader data showed the onset of the death phase in all samples. However, in B3 48 h low and artificial background samples, the OD dropped more rapidly to below 1 and 1.2, respectively. These variations between samples is addressed in the discussion.
To verify whether this lack of effect of the different radiation environments on the exponential growth phase could be extended to other bacteria, experiments were replicated using E. coli (Figure 4). Once again, there was grouping according to experimental run (24 and 48 h), not according to radiation environment, with the 24 h run having a shorter lag phase than that of the 48 h run. Similar to B. subtilis, “shoulders” appeared in the growth curves at ~200 min and at 300–400 min. However, unlike the wide variation of OD in the stationary and death phase of B. subtilis, the E. coli stationary phase OD values remained between 1 and 1.2. Additionally, there was no obvious grouping in the final yield between the low and artificial background samples, in contrast to the B. subtilis samples. The E. coli death phase was not captured during the aliquoted time of the experimental run.
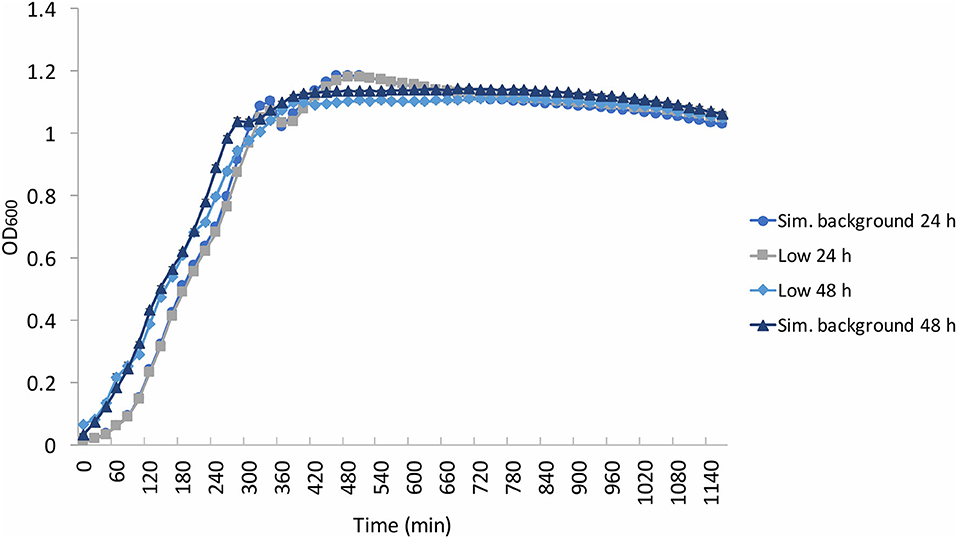
Figure 4. Average (n = 84) of E. coli growth curves at the determined low radiation (“low”) and artificial background radiation (“sim. background”) dose rate: 24 and 48 h denote time in hours that bacteria batch has spent in respective conditions. Error bars show ± standard error (n = 84).
Effects of Radiation on Growth Gradients and Rates
Figures 5A,B show the growth gradients and growth rates, respectively, of each experimental condition during the linear exponential growth phase. There was no significant (p > 0.05) difference between the low and artificial background radiation growth gradient at either time condition in the B. subtilis samples. However, the E. coli growth gradient showed a significant (p < 0.05) difference between the two time conditions in the artificial background radiation. The E. coli growth gradients for 24 and 48 h in artificial background radiation were 0.0866 (±4.33 × 10−3) and 0.0693 (± 3.92 × 10−3), respectively. The growth rates in Figure 5B were calculated for the average of all 84 samples in each condition and reflect the general trends shown by the growth gradients in Figure 5A.
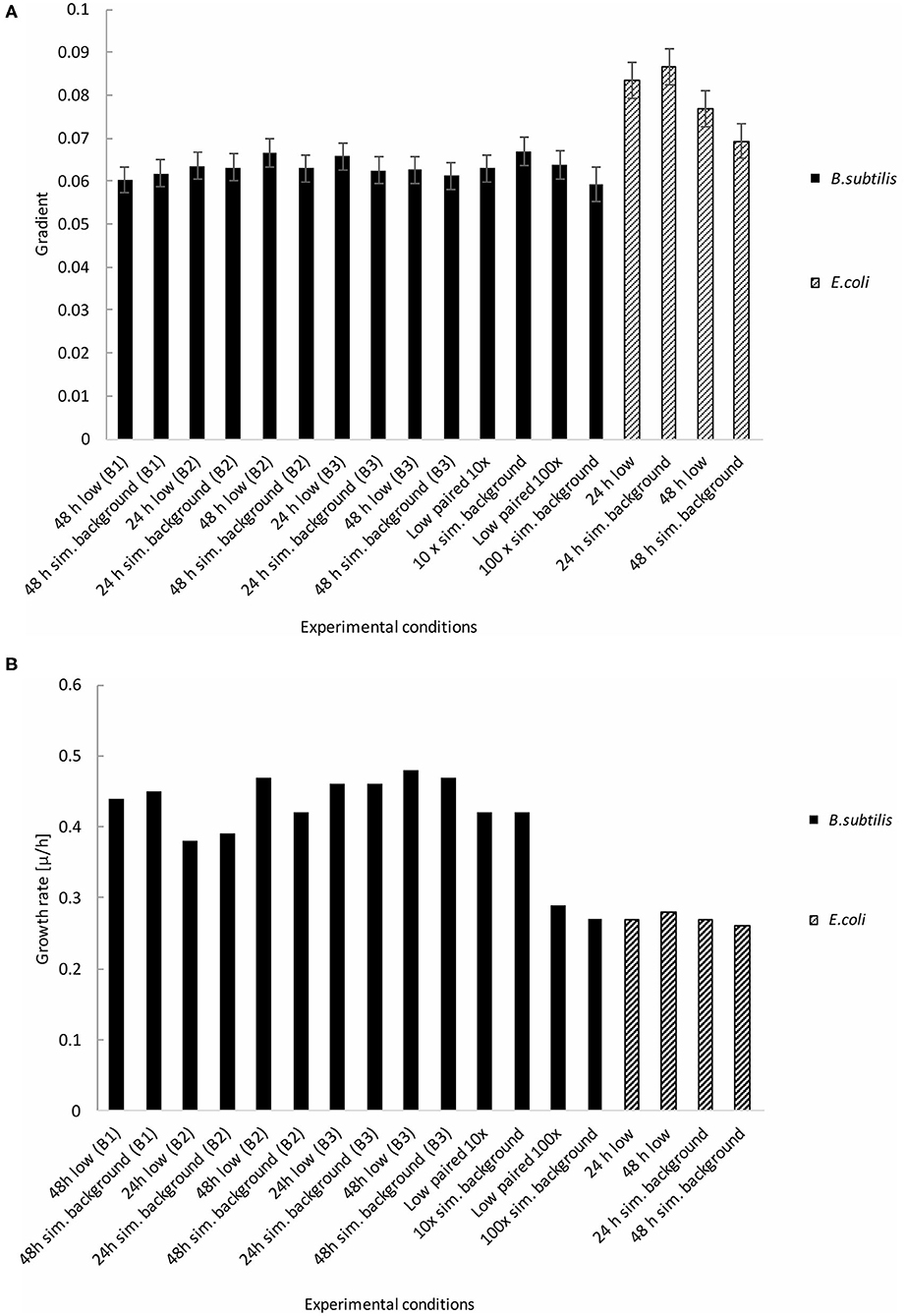
Figure 5. Growth gradients and rates of B. subtilis and E. coli linear exponential growth phases at the determined low radiation (“low”) and artificial background radiation (“sim. background”) dose rate: 24 and 48 h denote time in hours that bacteria batch has spent in respective conditions; B1-3 denote week number when the multiple B. subtilis samples were run; 10 × = 10 × the artificial background dose rate; 100 × = 100 × the artificial background dose rate; Low paired = low dose rate samples run parallel to 10 × and 100 × dose rates. Error bars show ± error of gradient. “B.s” and “E.c.” stand for B. subtilis and E. coli, respectively. (A) Growth gradients of B. subtilis and E. coli from the linear exponential growth phase. (B) Growth rates of B. subtilis and E. coli from the linear exponential growth phase.
B. subtilis Exposure to 10 × and 100 × Background Radiation
The B. subtilis growth curves displayed the same characteristics as the previous experiments, exhibiting a grouping in the exponential phase according to time condition, not radiation environment, in addition to a “shoulder” in the growth curve, and variation in OD during the stationary and death phases (Figure 6). Despite a non-significant (p = 0.13) difference in growth gradient (Figure 5A) and difference growth rate (Figure 5B) between the 10 × and 100 × artificial background radiation values, neither the 10 × or 100 × values were significantly (p > 0.05) different from the corresponding low radiation values in experiments run simultaneously as controls (Figure 5A). This implies that the difference in radiation dose rate was not the variable affecting growth.
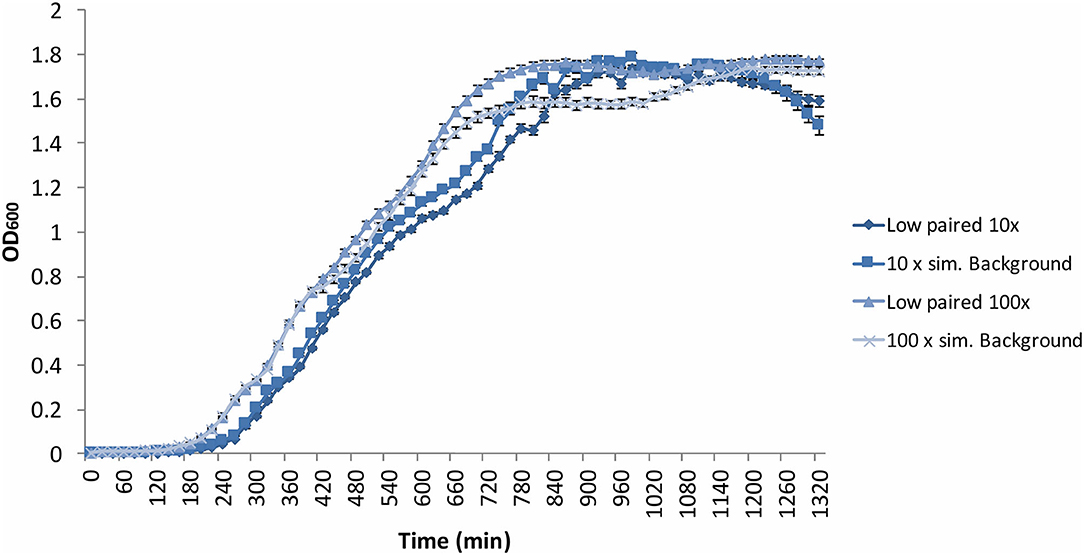
Figure 6. Average (n = 84) of B. subtilis growth curves at increased radiation dose rates: 10 × = 10 × the artificial background dose rate; 100 × = 100 × the artificial background dose rate; Low paired = samples run parallel at the low dose rate. Error bars show ± standard error (n = 84).
Response to Stress
The final preconditioning experiment was carried out to determine whether cells grown in a low radiation environment are more susceptible to short-term imposed stress compared to cells grown with the artificial background radiation exposure. B. subtilis and E. coli cultures that had been growing in low and artificial background radiation environments for 24 and 48 h, respectively, were exposed to a short burst of UVC radiation (λ = 254 nm) for 30 s and plated to assess their post-irradiation viability (Figure 7). There was no significant (p > 0.05) difference in viability observed after stress exposure between the cells grown in low or artificial background radiation, or between the two organisms.
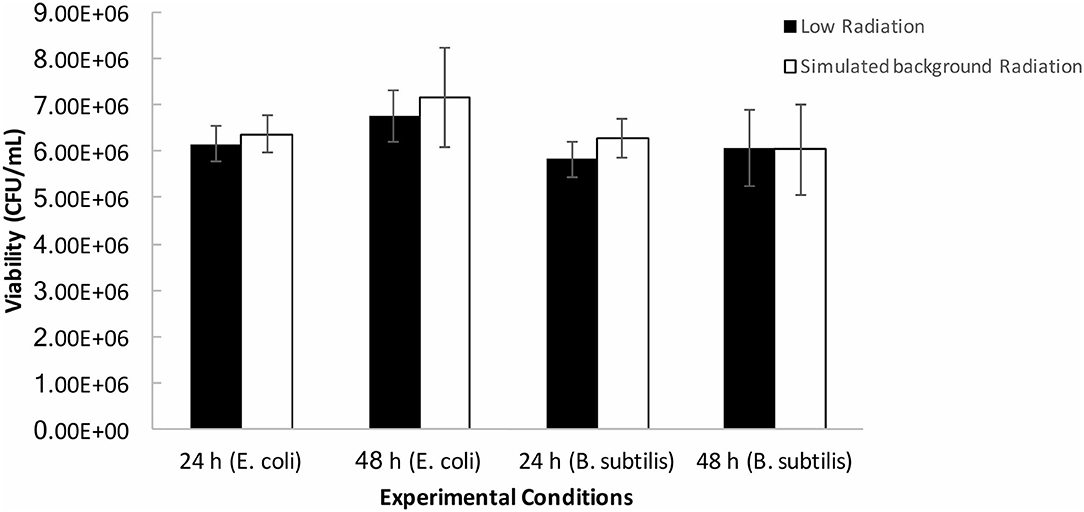
Figure 7. Averages (n = 9 per condition) of post-UV irradiation cell counts of B. subtilis and E. coli grown under the determined low radiation (“low”) and artificial background radiation (“sim. background”) dose rate: 24 and 48 h denote time in hours that bacteria batch has spent in respective conditions. Error bars show ± standard error (n = 9 per condition).
Discussion
Scientific investigation of the lethal consequences caused by high radiation exposure for both prokaryotic and eukaryotic organisms continues to shape the understanding of habitability and the limits of life. Although there is an abundance of off-planet, high-radiation environments of interest (e.g., Cockell et al., 2000; Marion et al., 2003), naturally occurring low radiation environments represent equally important extreme conditions of potential habitability to examine. These might include subsurface environments with low abundances of radioactive minerals, older planets with decayed radioactive minerals, solar systems containing stars with a lower ionising radiation output and regions of the galaxy with a quieter cosmic ray background, compared to the Earth.
Effects of Radiation on Bacterial Growth
We did not observe significant effects of low, artificial background, or heightened radiation environments (10 and 100 × surface background levels) on the linear exponential growth phase gradients of B. subtilis and E. coli when compared to the respective controls. This contradicts the LNT model which predicts increasing levels of radiation would have an increasing effect on growth rate from a zero dose upwards. These data also contradict the hormesis model, that predicts a beneficial effect on growth rate at low levels of radiation. Instead, the data point to a threshold model for bacterial growth, whereby at low levels of radiation below background and up to 100 times background no effect is observable on growth. It should be noted that although the SELLR experimental set up was designed to observe the bacterial responses without bias toward any particular model, the set up may unintentionally favour certain models. We cannot rule out that hormesis takes place between any of the tested dose rates of 1 ×, 10 ×, and 100 × background radiation. Future studies may wish to investigate a further narrowing of dose rates to more accurately rule out hormesis below our chosen radiation threshold.
We did observe variations in our experiments. The variation of lag phase is observed in all experiments performed. However, the variation in samples is grouped by experimental run and not by radiation environment, making the difference in radiation dose rate unlikely to be the cause. The variation in lag phase length is most likely due to small discrepancies in starting concentration of the samples. This experimental problem is not applicable to the exponential growth phase as it will be comparable regardless of small variations in starting inoculum.
An additional artefact in the B. subtilis and E. coli growth curves is the presence of a “shoulder” in the exponential growth phase. This may be a result of the bacteria exhausting the primary nutrient source and switching to a secondary one, which is probable in the nutrient-rich media used. We did not use this secondary growth in our calculations.
The final growth yield data also show a wide diversity of OD values for B. subtilis. Factors that explain this may include, for example, cell lysis resulting in the release of various organics, which would influence cell growth and thus the final optical density measurement. Previous research has used final yield OD data as a measure of microbial responses to low radiation conditions (e.g., Castillo et al., 2015). However, the different contributions of cell growth, stationary phase, and cell death will determine final OD readings and can therefore make the final OD interpretation potentially unreliable. As the exponential phase provides a measure of the rate of cell division and thus the effects of the radiation environment on cell reproduction, we consider this to be a good way to measure the effects of radiation.
Although the Boulby data challenges the LNT and hormesis models for low radiation doses, the indication that low dose rates do not impede bacterial growth is not unexpected. The empirical lethal dose necessary to sterilise 90% (“lethal dose” LD90) of E. coli MG 1655 is 700 Gy (2.59 × 108 higher than the 24 h total dose received by Boulby control cells) (Daly et al., 2004). Although no LD90 data could be found for vegetative B. subtilis cells, the majority of life is not radio-resistant and can be killed by <500 Gy (Moeller et al., 2010; Slade and Radman, 2011). The cells subjected to 24 h of artificial background radiation in our experiment are exposed to a total dose of 2.7 μGy (or 5.4 μGy for 48 h experiments), whereas the cells' total dose after 24 h in the low radiation environment amounts to 0.012 μGy (0.024 μGy for 48 h experiments). Therefore, the total dose experienced by cells would have to be up to 8 orders of magnitude higher than the artificial background total dose to reach LD90 (Daly, 2012). This also explains why, when the artificial background dose rate is increased by 10 × and 100 ×, it is unsurprising that no effect on bacterial growth was observed.
To further understand the scale at which radiation dose can influence cell viability, the likelihood of radiation-cell interaction must be considered. This likelihood was calculated by Lampe et al. (2016) for evolutionary research in the Modane Underground Laboratory (LSM) at 1.7 km below the Fréjus Peak [4.8 km water equivalent (Piquemal, 2012), Boulby is 3 km water equivalent Robinson et al., 2003]. Using 7.4 × 10−4 mutations per generation as the spontaneous point mutation occurrence in E. coli (Barrick et al., 2009), they calculate that such natural mutation occurrences would happen at a 1.04 × 102 higher frequency than what they calculate for radiation-cell interactions. They compute that a dose rate of >20 μGy/h (=175 mGy/y) is required for the radiation-cell interactions to have a detectable impact above the natural mutation “noise.” These calculations further explain the lack of observable difference between the Boulby low, artificial background, and 100 × artificial background (100 mGy/y) radiation dose rates on bacterial exponential growth. These data suggest that the radiation levels studied here are much lower than those required for significant cellular damage and reduction in growth.
Although the growth data may not rule out sublethal biochemistry and genetic responses caused by low radiation, as suggested by Castillo et al. (2015, 2018), growth (i.e., reproduction) is the ultimate metric of whether an organism is able to expand into a habitat. If sublethal effects are present, they remain insufficient to ramify through metabolic pathways to impede growth rates. Based on our study, we can assume that exposure to an extremely low radiation environment would not negatively impact life.
Response to Stress
Previous research has put forward the hypothesis that growth in a low radiation environment causes heightened sensitivity to stress factors. Satta et al. (1995) demonstrated that Saccharomyces cerevisiae had increased sensitivity to the carcinogen methyl methanesulfonate after growth in a low radiation environment (0.6 μGy/d, compared to Boulby's 0.012 μGy/d). Additionally, it has been suggested (Castillo et al., 2015) that cultures grown in low radiation for 24 h are less able to respond to reactive oxygen species (ROS) attacking the cell. ROS can be produced by radiation interacting with water (Ward, 1988), with higher dose rates causing higher ROS production. It was proposed that the lack of radiation, and by extension lower ROS production, fails to “prime” cells' response pathways for internal ROS removal, resulting in ROS accumulation and consequent stress.
This prompted our own experiment using UVC radiation exposure as a stress factor to determine whether there was any difference in cell sensitivity to stress after growth at low radiation vs. growth under artificial surface radiation. The cellular response was measured in terms of cell viability after UV exposure. The results show no significant difference in either B. subtilis or E. coli viability grown at low or artificial background radiation dose rates.
These results suggest that even if there are biochemical effects in cells at below background radiation levels that are not manifested in growth, they also do not affect a cell's short-term response to oxidative and radiation stress imposed by UV radiation.
Threshold Model
Figure 8 synthesises the results obtained in this work. Between the total dose range of 10−8 to 10−4 Gy our data suggests a threshold model where there is no effect on bacterial growth at this dose and lower. The lowest total dose received by the Boulby samples was that of the 24 h exposure at the artificial background dose rate of 0.01 mGy/y resulting in a total dose of 2.74 × 10−8 Gy; the highest total dose received was the 100 × the artificial background sample resulting in 2.74 × 10−4 Gy. Above this threshold but below doses that lead to loss of viability (10−4 to 102 Gy), we cannot rule out hypersensitivity or hermetic effects. There may be many additional “saddle points” in the model depending on factors such as dose-rate and organism-specific effects. Thus, the possibility of hypersensitivity or hormetic effect models may still be valid. Our results also focus on the exponential growth phase. However, if sublethal biochemical effects occur at below background radiation levels, as has been suggested for other cells (e.g., Kawanishi et al., 2012; Castillo et al., 2018), then it could suggest that different models apply depending on the scale or process considered and that no universal model for low radiation effects exists.
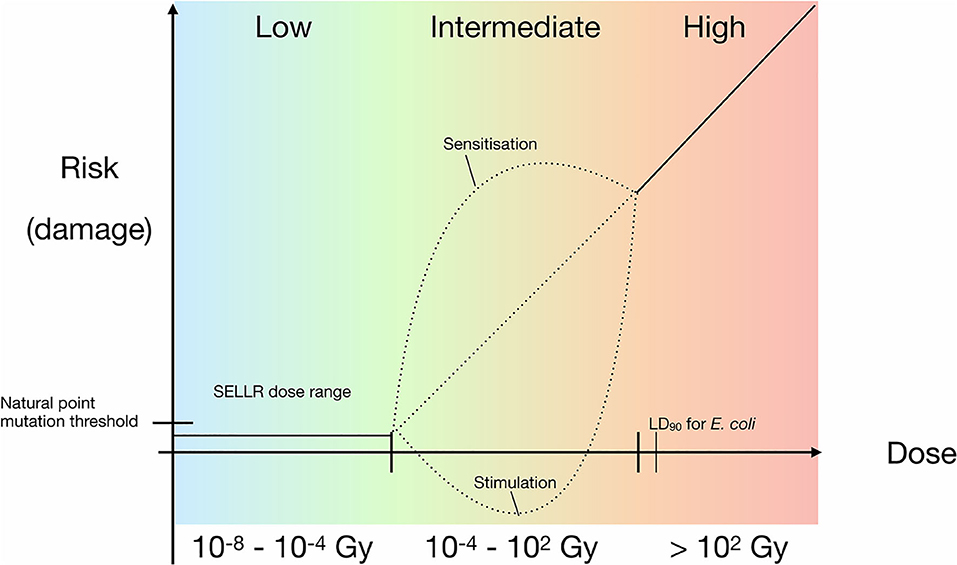
Figure 8. Updated prokaryotic dose-response model with a linear relationship for higher doses and added SELLR (Subsurface Experiment of Life in Low Radiation) observations from sub-background to 100 × background, representing the “low” dose range where we observe no difference in growth. Black dotted lines indicate hypothetical risk of damage at intermediate radiation exposure, which requires further research.
The data presented here indicate that there are no significant effects at either ultra low, artificial terrestrial surface, or heightened radiation dose rates on bacterial growth, and no difference in bacterial sensitivity to stress in the form of shortwave UV exposure after growth in low radiation environments. Thus, the preliminary data indicate that the extreme condition of low radiation in an environment would not restrict microbial expansion in a potential habitat. Additionally, the lack of a measurable linear effect or beneficial effects of low-level radiation suggest that both the LNT and hormesis models are inapplicable at these doses. Instead, a threshold model is suggested with no observable effect on viability below the terrestrial surface radiation dose up to a critical level exceeding the surface dose. The “critical threshold model” that emerges from our data might be applicable to a wide range of physiological processes in prokaryotes and be a useful model to consider in future low radiation environment studies. However, the further questions raised by our data show that further research on life in low radiation environments is merited.
Data Availability Statement
The raw data supporting the conclusions of this article will be made available by the authors, without undue reservation, to any qualified researcher.
Author Contributions
Internal dose measurements were performed by PS. Internal and external dose calculations were carried out by LC. External dose measurements were carried out by SP and LC. GEANT4 simulations were performed by AN under the supervision of AM. Growth measurements were performed by JW with the help of CT and EM. The manuscript was written by JW with help from CC, AM, and PS. All authors contributed to the article and approved the submitted version.
Funding
JW was funded by the UK Space Agency Aurora Studentship grant (STFC ST/M003612/1); CC was funded by the Science and Technology Facilities Council grant (ST/R000875/1); AN was funded by the Commonwealth Scholarship Commission grant (BDCS-2016-49); AM was funded by the Science and Technology Facilities Council grant (ST/N000269/1); LC was funded though the Trinity College M.Sc Physics programme; Boulby Underground Laboratory staff were funded by core Science and Technology Facilities Council facility funding.
Conflict of Interest
The authors declare that the research was conducted in the absence of any commercial or financial relationships that could be construed as a potential conflict of interest.
Acknowledgments
We would like to acknowledge the technical assistance provided by Scott McLaughlin and general assistance provided by Barbara Suckling and Louise Yeoman.
References
Abgrall, N., Arnquist, I. J., Avignone, F. T. III., Barabash, A. S., Bertrand, F. E., Boswell, M., et al. (2016). The majorana demonstrator radioassay program. Nucl. Instrum. Meth. A 828, 22–36. doi: 10.1016/j.nima.2017.08.005
Agostinelli, S., Allison, J., Amako, K., Apostolakis, J., Araujo, H., Arce, P., et al. (2003). GEANT4—a simulation toolkit. Nucl. Instrum. Meth. A 506, 250–303. doi: 10.1016/S0168-9002(03)01368-8
Aprile, E., Aalbers, J., Agostini, F., Alfonsi, M., Amaro, F. D., Anthony, M., et al. (2017). The XENON1T dark matter experiment. Eur. Phys. J. C 77:881. doi: 10.1140/epjc/s10052-017-5326-3
Araújo, H. M., Akimov, D. Y., Barnes, E. J., Belov, V. A., Bewick, A., Burenkov, A. A., et al. (2012). Radioactivity backgrounds in, ZEPLIN–III. Astropart. Phys. 35, 495–502. doi: 10.1016/j.astropartphys.2011.11.001
Barrick, J. E., Yu, D. S., Yoon, S. H., Jeong, H., Oh, T. K., Schneider, D., et al. (2009). Genome evolution and adaptation in a long-term experiment with Escherichia coli. Nature 461, 1243–1247. doi: 10.1038/nature08480
Calabrese, E. J., and Baldwin, L. A. (2003). Hormesis: the dose-response revolution. Ann. Rev. Pharmacol. Toxicol. 43, 175–197. doi: 10.1146/annurev.pharmtox.43.100901.140223
Castillo, H., Li, X., Schilkey, F., and Smith, G. B. (2018). Transcriptome analysis reveals a stress response of Shewanella oneidensis deprived of background levels of ionising radiation. PLoS ONE 13:e0196472. doi: 10.1371/journal.pone.0196472
Castillo, H., Schoderbek, D., Dulal, S., Escobar, G., Wood, J., Nelson, R., et al. (2015). Stress induction in the bacteria Shewanella oneidensis and Deinococcus radiodurans in response to below-background ionising radiation. Int. J. Radiat. Biol. 91, 749–756. doi: 10.3109/09553002.2015.1062571
Chang, J. C., Ossoff, S. F., Lobe, D. C., Dorfman, M. H., Dumais, C. M., Qualls, R. G., et al. (1985). UV inactivation of pathogenic and indicator microorganisms. Appl. Environ. Microbiol. 49, 1361–1365. doi: 10.1128/AEM.49.6.1361-1365.1985
Cockell, C. S., Catling, D. C., Davis, W. L., Snook, K., Kepner, R. L., Lee, P., et al. (2000). The ultraviolet environment of mars: biological implications past, present, and future. Icarus 146, 343–359. doi: 10.1006/icar.2000.6393
Costantini, D., and Borremans, B. (2019). The linear no-threshold model is less realistic than threshold or hormesis-based models: an evolutionary perspective. Chem. Biol. Interac. 301, 26–33. doi: 10.1016/j.cbi.2018.10.007
Daly, M. J. (2012). Death by protein damage in irradiated cells. DNA Repair 11, 12–21. doi: 10.1016/j.dnarep.2011.10.024
Daly, M. J., Gaidamakova, E. K., Matrosova, V. Y., Vasilenko, A., Zhai, M., Venkateswaran, A., et al. (2004). Accumulation of Mn (II) in deinococcus radiodurans facilitates gamma-radiation resistance. Science 306, 1025–1028. doi: 10.1126/science.1103185
de González, A. B., and Darby, S. (2004). Risk of cancer from diagnostic X-rays: estimates for the UK and 14 other countries. Lancet 363 345–351. doi: 10.1016/S0140-6736(04)15433-0
de Vera, J. P., Alawi, M., Backhaus, T., Baqué, M, Billi, D., Böttger, U., et al. (2019). Limits of life and the habitability of mars: the ESA space experiment BIOMEX on the ISS. Astrobiology 19, 145–157. doi: 10.1089/ast.2018.1897
Feinendegen, L. E., Pollycove, M., and Neumann, R. D. (2007). Whole-body responses to low-level radiation exposure: new concepts in mammalian radiobiology. Exp. Hematol. 35, 37–46. doi: 10.1016/j.exphem.2007.01.011
Feynman, R. P. (1960). There's Plenty of Room at the Bottom. Boca Raton, FL: California Institute of Technology, Engineering and Science Magazine.
Finch, S. C. (2007). Radiation-induced leukemia: lessons from history. Best Prac. Res. Clin. Haematol. 20, 109–118. doi: 10.1016/j.beha.2006.10.009
Ghiassi-Nejad, M., Mortazavi, S. M. J., Cameron, J. R., Niroomand-Rad, A., and Karam, P. A. (2002). Very high background radiation areas of ramsar, Iran: preliminary biological studies. Health Phys. 82, 87–93. doi: 10.1097/00004032-200201000-00011
Hamilton, E. I. (1989). Terrestrial radiation—an overview. Int. J. Radiat. Appl. Instrument. Part C 34, 195–212. doi: 10.1016/1359-0197(89)90230-0
Horneck, G. (1992). Radiobiological experiments in space: a review. Int. J. Radiat. Appl. Instrument. Part D 20, 185–205. doi: 10.1016/1359-0189(92)90099-H
International Agency for Research on Cancer (1992). Monograph on the Evaluation of Carcinogenic Risks to Humans: Solar and Ultraviolet Radiation, Vol. 55. World Health Organization, 113–122.
Kawanishi, M., Okuyama, K., Shiraishi, K., Matsuda, Y., Taniguchi, R., Shiomi, N., et al. (2012). Growth retardation of paramecium and mouse cells by shielding them from background radiation. J. Radiat. Res. 53, 404–410. doi: 10.1269/jrr.11145
Lampe, N., Biron, D. G., Brown, J. M. C., Incerti, S., Marin, P., Maigne, L., et al. (2016). Simulating the impact of the natural radiation background on bacterial systems: implications for very low radiation biological experiments. PLoS ONE 11:e016364. doi: 10.1371/journal.pone.0166364
Lorenz, E. (1944). Radioactivity and lung cancer; a critical review of lung cancer in the miners of schneeberg and joachimsthal. J. Natl. Cancer Inst. 5, 1–15.
Lorenz, E. (1950). Some biologic effects of long continued irradiation. Am. J. Roentgenol. Radium Ther. 63, 176–185.
Mancuso, M., Pasquali, E., Giardullo, P., Leonardi, S., Tanori, M., Di Majo, V., et al. (2012). The radiation bystander effect and its potential implications for human health. Curr. Mol. Med. 12, 613–624. doi: 10.2174/156652412800620011
Marion, G. M., Fritsen, C. H., Eicken, H., and Payne, M. C. (2003). The search for life on Europa: limiting environmental factors, potential habitats, and Earth analogues. Astrobiology 3, 785–811. doi: 10.1089/153110703322736105
Mine, M., Okumura, Y., Ichimaru, M., Nakamura, T., and Kondo, S. (1990). Apparently beneficial effect of low to intermediate doses of A-bomb radiation on human lifespan. Int. J. Radiat. Biol. 58, 1035–1043. doi: 10.1080/09553009014552341
Moeller, R., Douki, T., Rettberg, P., Reitz, G., Cadet, J., Nicholson, W. L., et al. (2010). Genomic bipyrimidine nucleotide frequency and microbial reactions to germicidal UV radiation. Arch. Microbiol. 192, 521–529. doi: 10.1007/s00203-010-0579-3
Moeller, R., Raguse, M., Leuko, S., Berger, T., Hellweg, C. E., Fujimori, A., et al. (2017). STARLIFE—An international campaign to study the role of galactic cosmic radiation in astrobiological model systems. Astrobiology 17101–109. doi: 10.1089/ast.2016.1571
Muller, H. J. (1954). Damage to posterity caused by irradiation of the gonads. Am. J. Obstetr. Gynecol. 67, 467–483. doi: 10.1016/0002-9378(54)90039-3
National Research Council (NRC) (2006). Health Risks from Exposure to Low Levels of Ionizing Radiation: BEIR VII Phase 2, Vol. 7. Washington, DC: National Academies Press.
Olson, A. R., and Lewis, G. N. (1928). Natural reactivity and the origin of species. Nature 121673–674. doi: 10.1038/121673a0
Piquemal, F. (2012). Modane underground laboratory: Status and project. Eur. Phys. J. Plus 127:110. doi: 10.1140/epjp/i2012-12110-3
Preston, D. L., Pierce, D. A., Shimizu, Y., Cullings, H. M., Fujita, S., Funamoto, S., et al. (2004). Effect of recent changes in atomic bomb survivor dosimetry on cancer mortality risk estimates. Radiat. Res. 162, 377–389. doi: 10.1667/RR3232
Prise, K. M., Pinto, M., Newman, H. C., and Michael, B. D. (2001). A review of studies of ionising radiation-induced double-strand break clustering. Radiat Res. 156, 572–576. doi: 10.1667/0033-7587(2001)1560572:AROSOI2.0.CO
Reichhart, L., Lindote, A., Akimov, D. Y., Araújo, H. M., Barnes, E. J., Belov, V. A., et al. (2013). Measurement and simulation of the muon-induced neutron yield in lead. Astroparticle Phys. 47, 67–76. doi: 10.1016/j.astropartphys.2013.06.002
Robinson, M., Kudryavtsev, V. A., Lüscher, R., McMillan, J. E., Lightfoot, P. K., Spooner, N. J. C., et al. (2003). Measurements of muon flux at 1070m vertical depth in the Boulby underground laboratory. Nucl. Inst. Methods Phys. Res. Section A 511, 347–353. doi: 10.1016/S0168-9002(03)01973-9
Satta, L., Augusti-Tocco, G., Ceccarelli, R., Esposito, A., Fiore, M., Paggi, P., et al. (1995). Low environmental radiation background impairs biological defence of the yeast Saccharomyces cerevisiae to chemical radiomimetic agents. Mutat. Res. Lett. 347, 129–133. doi: 10.1016/0165-7992(95)00031-3
Scovell, P. R., Meehan, E., Araújo, H. M., Dobson, J., Ghag, C., Kraus, H., et al. (2018). Low-background gamma spectroscopy at the Boulby Underground Laboratory. Astropart. Phys. 97, 160–173. doi: 10.1016/j.astropartphys.2017.11.006
Shahbazi-Gahrouei, D., Gholami, M., and Setayandeh, S. (2013). A review on natural background radiation. Adv. Biomed. Res. 2:65 doi: 10.4103/2277-9175.115821
Shamoun, D. Y. (2016). Linear No-Threshold model and standards for protection against radiation. Reg. Toxicol. Pharmacol. 77, 49–53. doi: 10.1016/j.yrtph.2016.02.011
Shibamoto, Y., and Nakamura, H. (2018). Overview of biological, epidemiological, and clinical evidence of radiation hormesis. Int. J. Mol. Sci. 19:2387. doi: 10.3390/ijms19082387
Siasou, E., Johnson, D., and Willey, N. J. (2017). An extended dose–response model for microbial responses to ionising radiation. Front. Environ. Sci. 5:6. doi: 10.3389/fenvs.2017.00006
Slade, D., and Radman, M. (2011). Oxidative stress resistance in Deinococcus radiodurans. Microbiol. Mol. Biol. Rev. 75, 133–191. doi: 10.1128/MMBR.00015-10
Smith, G. B., Grof, Y., Navarrette, A., and Guilmette, R. A. (2011). Exploring biological effects of low level radiation from the other side of background. Health Phys. 100, 263–265. doi: 10.1097/HP.0b013e318208cd44
Stebbing, A. R. D. (1982). Hormesis—the stimulation of growth by low levels of inhibitors. Sci. Total Environ. 22, 213–234. doi: 10.1016/0048-9697(82)90066-3
United Nations (2008). Scientific Committee on the Effects of Atomic Radiation. Report of the United Nations Scientific Committee on the Effects of Atomic Radiation: Fifty-Sixth Session (10-18 July 2008). No. 46. United Nations Publications.
Wanebo, C. K., Johnson, K. G., Sato, K., and Thorslund, T. W. (1968). Breast cancer after exposure to the atomic bombings of hiroshima and nagasaki. N Engl J Med. 279, 667–671. doi: 10.1056/NEJM196809262791301
Keywords: subsurface microbiology, low radiation, extreme habitat, dose response, threshold model
Citation: Wadsworth J, Cockell CS, Murphy AS, Nilima A, Paling S, Meehan E, Toth C, Scovell P and Cascorbi L (2020) There's Plenty of Room at the Bottom: Low Radiation as a Biological Extreme. Front. Astron. Space Sci. 7:50. doi: 10.3389/fspas.2020.00050
Received: 05 May 2020; Accepted: 06 July 2020;
Published: 14 August 2020.
Edited by:
Geoffrey Battle Smith, New Mexico State University, United StatesReviewed by:
Evgenios Agathokleous, Nanjing University of Information Science and Technology, ChinaOliver Strbak, Comenius University, Slovakia
Copyright © 2020 Wadsworth, Cockell, Murphy, Nilima, Paling, Meehan, Toth, Scovell and Cascorbi. This is an open-access article distributed under the terms of the Creative Commons Attribution License (CC BY). The use, distribution or reproduction in other forums is permitted, provided the original author(s) and the copyright owner(s) are credited and that the original publication in this journal is cited, in accordance with accepted academic practice. No use, distribution or reproduction is permitted which does not comply with these terms.
*Correspondence: Jennifer Wadsworth, amVubkB3YWRzd29ydGguY2g=