- 1Space Biochemistry Group, Department of Biophysical Chemistry, University of Vienna, Vienna, Austria
- 2Archaea Biology and Ecogenomics Division, Department of Ecogenomics and Systems Biology, University of Vienna, Vienna, Austria
- 3Center for Sensor Technology (CST), Faculty of Mechanical Engineering, University of Maribor, Maribor, Slovenia
- 4Max Planck Institute for Marine Microbiology, Bremen, Germany
Recent studies have uncovered a vast number of thermophilic species in icy environments, permanently cold ocean sediments, cold sea waters, and cool soils. The survival of thermophiles in psychrobiotic habitats requires thorough investigation of the physiological and molecular mechanisms behind their natural cryopreservation. Such investigations are mainly impeded due to a restricted cultivation of thermophiles at low temperatures under the laboratory conditions. Artificial culture media used under the laboratory conditions usually fail to support cultivation of thermophiles at low-temperature range. In this study we cultivated the extreme thermoacidophilic archaeon Metallosphaera sedula with the preliminary powdered and sterilized multimetallic extraterrestrial mineral material (the meteorite NWA 1172) under a low temperature regime in laboratory conditions. Our data indicate that M. sedula withstands cold stress and can be maintained at low temperatures, when supplemented with the meteorite NWA 1172 as the sole energy source. Cultivation with the meteorite NWA 1172 opens up new, previously unknown psychrotolerant characteristics of M. sedula, emphasizing that culture conditions (i.e., the “nutritional environment”) may affect the microbial survival potential in stress related situations. These observations facilitate further investigation of strategies and underlying molecular mechanisms of the survival of thermophilic species in permanently cold habitats.
Introduction
Diverse extremophilic microorganisms have been discovered in habitats characterized by parameters that go beyond the range of their physiological activity. For example, a variety of heat-loving microbial species have been isolated from low temperature environments across the globe. Recent independent investigations have uncovered a vast number of thermophilic species in icy habitats (Bulat et al., 2004; Lavire et al., 2006; Bulat, 2016; Papale et al., 2019; Gura and Rogers, 2020); permafrost environments (Gilichinsky et al., 2007; Demidov and Gilichinsky, 2009; Mironov et al., 2010; Shcherbakova et al., 2011); cool soils with temperatures constantly below 25°C (Marchant et al., 2002, 2008, 2011; Rahman et al., 2004; Zeigler, 2014); “arctic thermophiles” in permanently cold (−2° to 4°C) ocean sediments (Hubert et al., 2009, 2010; de Rezende et al., 2013; Müller et al., 2014; Robador et al., 2016; Bell et al., 2018, 2020; Chakraborty et al., 2018); and non-spore-forming hyperthermophiles in cold (2–4°C) seawater (Huber et al., 1990; Wirth, 2017). How these thermophilic species, abundantly represented in permanently cold habitats, can tolerate low temperatures significantly below their minimum requirement for growth is an intriguing subject of current investigations. Thermophilic spore-forming bacteria in the marine sediments of Svalbard have been constantly reported (Vandieken et al., 2006; Hubert et al., 2009, 2010; Cramm et al., 2019). Their endospores with specialized cellular features that protect cells from extreme harsh environmental factors contribute to the survival of these thermophilic bacteria in Arctic sediments. Black smoker-associated hyperthermophiles utilize both adherence to suitable surfaces and fast motility as the driving forces for survivability in cold seawater for prolonged periods of time (Mora et al., 2014; Wirth, 2017). Wiegel (2002) proposed the hypothesis of temporary nanoniches in the mesobiotic environments. Such temporary nanoniches provide short-termed limited conditions for alkaliphilic thermophilic bacteria and therefore dictate fastened growth rate in order to cope with this limitation (Wiegel, 2002). Icelandic basaltic and rhyolitic glass and minerals in a sub-Arctic environment with the temperature range below required for thermophile activity were proposed to support transient growth of thermophiles in summer months (Cockell et al., 2015). The low albedo of these rocks has been suggested to facilitate their thermal conductivity and support the function of these igneous materials as microclimatic environments to harbor thermophilic microbial communities (Kelly et al., 2010, 2011; Cockell et al., 2015). Apparently, these nanoniches inside the rock pores provide a certain potential for their temporary “re-awakening” of such communities during the warm periods. On the molecular level, it has been suggested that microorganisms capable of growth in temperature range of both thermobiotic and mesobiotic environments may contain two different sets of key enzymes whose synthesis are regulated by temperature (Wiegel, 1990).
Understanding freezing tolerance and survival limits of thermophiles in low temperature habitats is also crucial for studies of microbial transfer through space and between celestial bodies, e.g., in the context of lithopanspermia. One of the scientific concepts for the origin and distribution of life is a long-distance shielded interplanetary viable transfer of the most ancient microbial forms of life entombed in lithic habitats (Mileikowsky et al., 2000; Fajardo-Cavazos et al., 2007; Horneck et al., 2008; Nicholson, 2009; Onofri et al., 2012; Kawaguchi et al., 2016). Extreme temperature fluctuations affect microbial “space travelers” during their interstellar transfer. Knowledge of the thermal limits of microbial life embedded into extraterrestrial mineral materials is crucial for envisaging the microbial survival during all lithopanspermia's stages (microbial launching due to the impact ejection from the planet of origin, long-term interplanetary traveling of microbes inside of rocks, and the capture of new life by the recipient planet). Furthermore, microbial cold stress experiments are helpful in terms of interpreting possible spatial and temporal environmental micro(nano)niches suitable for microbial life on Mars. Such near-surface microenvironments of Mars can provide potential habitable niches protected not only from UV, but also from extreme temperature fluctuations. Chin et al. (2010) indicated that chaotropic metabolites and chaotropic environments can increase tolerance to subzero temperatures, extending growth windows in cold ecosystems. Chaotropic ions in the Mars regolith might form microenvironments that support potential Martian biosphere, favoring the growth and preservation of a microbiota at low temperature. Comprehensive laboratory investigations on the survival limits of thermophiles in low temperature habitats can help to address the question if there are habitable niches on Mars today and if they harbor life.
However, our understanding of the physiology of how non-spore-forming thermophiles adapt to the cold is far from being explicit. The major limitation in the field comes from a restricted cultivation of thermophiles at low temperature regiment under laboratory conditions. Artificial culture media used under laboratory conditions usually fail to support cultivation of thermophiles at low-temperature range. One of the rare exceptions is Geobacillus thermoleovorans strain T80 which was cultivated at 4°C during a long-term period (9 month) and supported in soil microcosms at low temperatures during short-term experiments (1 week; Marchant et al., 2008). Wiegel (1990) described that extreme thermophile Methanobacterium thermoautotrophicum can grow between 22 and 78°C and the addition of sterile anaerobic sediments permitted its incubation at lower temperatures. The studies obtained under laboratory conditions show that hyperthermophiles can survive at least 9 months in cold surroundings when stored in low-temperature seawater (Mora et al., 2014). In this data report, we show that the extreme thermoacidophile Metallosphaera sedula is among a very few thermophiles supported at cold temperatures under laboratory conditions. Supplementation with the stony meteorite NWA 1172 permits the preservation of the thermoacidophile M. sedula under low temperature regime and provides further possibilities to study the molecular machinery and mechanisms implicated in survivability of non-spore-forming thermophiles at deep subfreezing temperatures.
Results and Discussion
The heat-, acid-, and heavy metal-resistant M. sedula represents a robust microbiological subject for stress related investigations, with a number of studies published (Peeples and Kelly, 1995; Beblo et al., 2009, 2011; Maezato et al., 2012; Mukherjee et al., 2012; McCarthy et al., 2014; Milojevic et al., 2019a). At the same time, there is an evident gap of knowledge regarding cold stress reactions of this extreme thermophilic archaeon. M. sedula has been described as a well-defined, obligate thermophile which requires a temperature range from 50 to 80°C for growth, with an optimum of 73°C (Huber et al., 1989; Auernik et al., 2008). However, the sediments from Pisciarelli solfatara, a volcanic field near Naples, Italy, where M. sedula was first isolated, are much cooler, between 25 and 52°C (Huber et al., 1989). Employing standard culture techniques and evaluating microbial growth, it is obvious that none of the validated terrestrial energy sources (chalcopyrite, pyrite, and other inorganic electron donors) support the cultivation of M. sedula in this lower temperature range (Huber et al., 1989; Auernik et al., 2008). However, chemolithoautotrophic cultures of M. sedula in presence of preliminary sterilized meteorite material (the stony chondrite H5 type NWA 1172 Russell et al., 2002; Milojevic et al., 2019b) as the sole energy source permitted the maintenance of M. sedula during 2 months at the average temperature of 12°C (Table S1). Ramping down the cultivation temperature of M. sedula to this cold temperature regime was achieved by providing a regularly exchanged ice-supplemented environment for the glass bioreactors (Figure 1). Similar incubation on sulfide ores did not yield in detectable cells after 2 months (Figure S1). Examination by multi-labeled fluorescence in situ hybridization (MiL-FISH) with a M. sedula-specific 16S rRNA-targeted probe, confirmed the identification of cells from the cultures supplemented with the meteorite at cold regime as M. sedula cells (Figure 2 and Figure S2). Furthermore, sequencing of the functional and phylogenetic M. sedula marker gene msed0966 (putative rusticyanin gene; Auernik and Kelly, 2008, 2010a,b) as well as its 16S rRNA gene confirmed M. sedula presence in low temperature cultures (Figure S3). Additionally, the content of the cultures was analyzed by scanning electron microscopy (SEM) (Figures 3A–C and Figure S4). Interestingly, our SEM observations of cold-maintained M. sedula indicated a presence of an extracellular matrix evenly spread over the cell surface, appearing as a layer of cellular appendages wrapping around the colonies of M. sedula similar to a biofilm layer (Figure 3 and Figure S4). In conjunction with these observations, when analyzing the cold-maintained cultures by fluorescence microscopy, slightly autofluorescing, dense, and opaque formations were observed (Figures 2A–C), which can be inferred to be the same aforementioned extracellular matrix. A branched extracellular matrix has not been detected in M. sedula cultures grown at 73°C on terrestrial minerals (Blazevic et al., 2019; Milojevic et al., 2019a) and NWA 1172 (Milojevic et al., 2019b), and might represent an adaptive feature of this thermophilic archaeon for withstanding stressful cold conditions. Another property of cold-maintained cells of M. sedula is their tendency to clump and condense into cellular aggregates (Figure 3 and Figure S4), which is an additional possible strategy for coping with low temperatures.
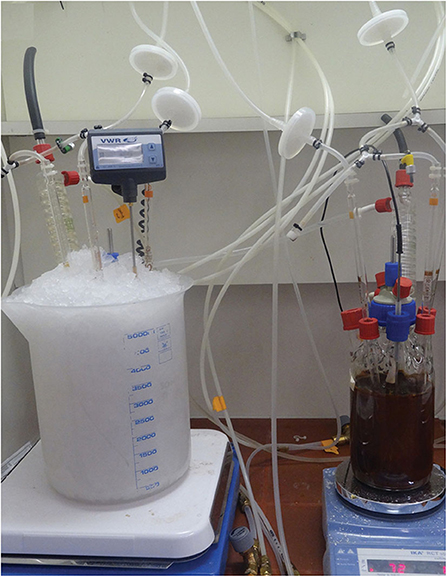
Figure 1. Cultures of M. sedula at cold temperature regime. Fermentation set up for cultivation of M. sedula in 1L glassblower modified Schott-bottle bioreactors at 73°C (right) and at cold temperature regime in ice-supplemented environment with a tap water heat exchange system (left).
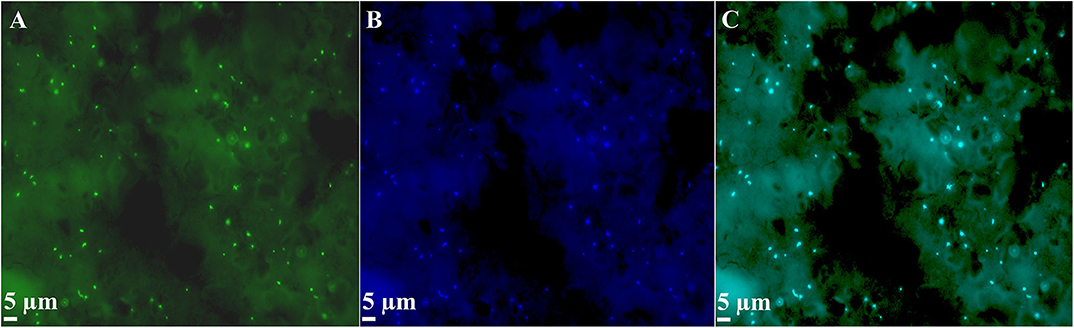
Figure 2. MiL-FISH (multi-labeled fluorescence in situ hybridization) of M. sedula cells supplemented with the stony meteorite NWA 1172 as the sole energy source at cold temperature regime. (A) MiL-FISH image of cells (green) after hybridization with the specific oligonucleotide probe targeting M. sedula. (B) DAPI staining of the same field (blue). (C) Corresponding overlaid epifluorescence image, showing 100% overlap of specific probe with DAPI signals. Cultures of M. sedula were examined with MiL-FISH conducted as described in Kölbl et al. (2017) and Milojevic et al. (2019a) after 2 months of cultivation at the average temperature of 12°C.
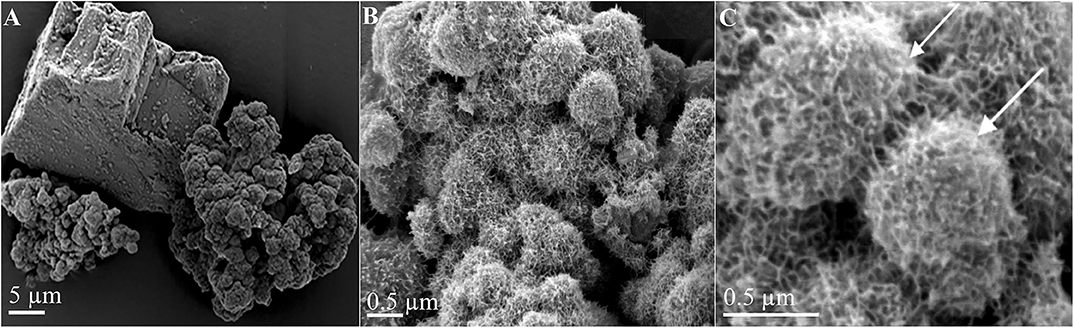
Figure 3. Scanning electron microscopy images of M. sedula supplemented with the stony meteorite NWA 1172 as the sole energy source at cold temperature regime. (A) Scanning electron image, showing an angular mineral fragment of NWA 1172 clustered by M. sedula aggregated cells at the cold temperature regime. (B,C) Higher magnification scanning electron images displaying single cells of M. sedula covered with extracellular matrix. Cells of M. sedula are indicated with white arrows. Cultures of M. sedula were examined with SEM after 2 months of cultivation at the average temperature of 12°C.
Our study indicates that the utilization of a specific mineral source and/or nutrients can influence the resistance to cold stress, contributing to the preservation of microbial cells in the cold. The characteristic inability of thermophilic M. sedula to be preserved in common laboratory media at temperatures below 50°C can be attributed to deficiencies in these media, e.g., in certain essential metabolites/metals which are indispensable for the cultivation of this organism at low temperatures, but that are not required at 73°C. Our previous studies depicted the beneficial contribution of NWA 1172 as the sole electron donor with a superior growth rate of M. sedula over chalcopyrite, suggesting preferential nature of this multimetalic material as energy source for M. sedula (Milojevic et al., 2019b). The NWA1172 stony meteorite is a non-carbonaceous H type ordinary chondrite, with high iron abundance (largely present in metallic form; Russell et al., 2002) and a wide range of other metal elements (Milojevic et al., 2019b). These metals might be alternatively used by M. sedula as specific metabolic cofactors offering more optimal structural and/or constitutive elements for enzyme activities, e.g., altering protein structural flexibility and shaping protein biophysical and biochemical properties.
A plausible explanation of the observed resistance of NWA 1172-grown M. sedula to cold stress can be inferred from heavy metals chaotropicity that extends the life window at low temperatures (Chin et al., 2010). Heavy metals may chaotropically enhance macromolecular flexibility (Chin et al., 2010; Cray et al., 2012) and so enable cell function/help maintain cellular structure at low temperatures. By exerting chaotropic activity heavy metals can cause specific toxic effects, inhibiting growth already at low concentrations in susceptible microorganisms. However, the studied archaeon M. sedula is an extreme metallophilic microorganism that tolerates elevated heavy metal concentrations (Maezato et al., 2012; Mukherjee et al., 2012; Blazevic et al., 2019; Milojevic et al., 2019a). Cultivation on multimetalic NWA 1172 meteorite (see Milojevic et al., 2019b for heavy metal composition of NWA 1172) exposes M. sedula to higher heavy metal concentrations under physiological conditions, thus helping to increase psychrotolerance. It is important to note that the effect of chaotropes that enhance microbial activity and growth at low temperatures appears to be initiated at around 10–12°C (Chin et al., 2010), which is the temperature range in our study. Thus, heavy metal chaotropicity may provide a mechanistic understanding of the increased psychrotolerance of M. sedula grown on NWA 1172.
Conclusion
In this study we report that when cultivated with meteorite, but not with chalcopyrite, cells of M. sedula can be preserved at cold temperature regime, denoting that the optimized nutrient fitness favor microbial preservation in extreme stressful conditions. Cultures of M. sedula supported at low temperature may serve as a laboratory model to explore metabolic potential of non-spore-forming thermophiles in psychrobiotic environments and the molecular mechanisms behind natural cryopreservation. It is important to note that future follow-up investigations should deliver a systematic study to determine which constituents of the meteorite are the crucial ingredients to ensure M. sedula culture preservation at low temperatures. In this regard, the survivability of M. sedula at low temperatures is a topic that deserves more attention and thorough analysis in the future. Moreover, the fact that an ancient inhabitant of terrestrial thermal springs and extreme thermophilic chemolithotroph M. sedula can be maintained at cold temperatures may complement the on-going scientific debates concerning psychrophilicity or thermophilicity of a last universal common ancestor (Wächtershäuser, 1992; Bada et al., 1994; Bada and Lazcano, 2002; Stetter, 2006; Akanuma et al., 2013), adding new circumstances in terms of survivability of ancestral thermophiles in cool primordial environments.
Materials and Methods
Strain and Media Composition
Metallosphaera sedula (DSMZ 5348) cultures were grown aerobically as described previously (Kölbl et al., 2017; Blazevic et al., 2019; Milojevic et al., 2019a,b) in DSMZ88 Sulfolobus medium containing 1.3 g (NH4)2SO4, 0.28 g KH2PO4, 0.25 g MgSO4·7 H2O, 0.07 g CaCl2·2 H2O, and 0.02 g FeCl3·6 H2O dissolved in 1 L of water. After autoclaving, Allen's trace elements solution was added to 1 L media resulting in 1.80 mg MnCl2·4 H2O, 4.50 mg Na2B4O7·10 H2O, 0.22 mg ZnSO4·7 H2O, 0.05 mg CuCl2·2 H2O, 0.03 mg Na2MoO4·2 H2O, 0.03 mg VSO4·2 H2O, and 0.01 mg CoSO4 final concentration. The pH was adjusted to 2.0 with 10 N H2SO4. Chemicals of high purity grade were used for media preparation.
Cultivation Setup
Chemolithoautotrophic cultivation of M. sedula was performed as described before (Blazevic et al., 2019; Milojevic et al., 2019a,b) in DSMZ88 Sulfolobus medium defined above in 1L glassblower modified Schott-bottle bioreactors (Duran DWK Life Sciences GmbH, Wertheim/Main, Germany), operated with a thermocouple linked to a heating and magnetic stirring plate (IKA RCT Standard/IKA C-MAG HS10, Lab Logistics Group GmbH, Meckenheim, Germany) for agitation and temperature control. The cultivation of M. sedula under cold temperature regime was achieved by providing a regularly exchanged ice-supplemented environment of fermentation glass bioreactors (Figure 1). For this the bioreactors were placed in plastic vessels filled with crushed ice and additionally supplemented with a tap water heat exchange system, consisting of 10 mm inner diameter silicon tubes, which were connected to a tap water supply. Melting of ice due to the room temperature fluctuations resulted in an established dynamic temperature profile of M. sedula cultures with the average temperature of 12°C, which was monitored with an electronic thermocouple inside the bioreactor and with a thermometer outside the bioreactor. For fermentation at cold temperature regime, the media was maintained at room temperature for 2 h prior inoculation. Directly after inoculation the glass bioreactors were provided with ice-supplemented environment and water heat exchange system in order to maintain cold temperature regime. Inocula used were exponentially growing H2-oxidizing autotrophic cultures of M. sedula. For chemolithoautotrophic growth cultures were supplemented with 10 g/liter either chalcopyrite (provided by E. Libowitzky from the mineral collection of the Department of Mineralogy and Crystallography, University of Vienna) or NWA 1172 (provided by the NHM, Vienna). The minerals were ground and temperature sterilized at 180°C in a heating oven for a minimum of 24 h prior to autoclaving (121°C, 20 min). Cells were monitored by phase contrast/epifluorescence microscopy. For the visualization of cells wiggling on solid particles they were stained by a modified “DAPI” (4′-6′- Diamidino-2-phenylindole) procedure (Huber et al., 1985), observed and recorded with ProgRes® MF cool camera (Jenoptik) attached to Nikon eclipse 50i microscope, operated with F36-500 Bandpass Filterset (ex, 377/50 nm; em, 447/60 nm).
PCR Assays for Marker Genes
DNA extracted by two different DNA isolation methods (Bead beating with Phenol-Chloroform extraction; MoBio PowerSoil DNA Isolation Kit) was used as template for PCR (Polymerase Chain Reaction) amplification. The PCR was targeting the 16S rRNA gene and the msed0966 gene (M. sedula putative rusticyanin) with the archaeal-specific 16S rRNA primers (109f 5′- ACKGCTCAGTAACACGT-3′; 1492r 5′-GGYTACCTTGTTACGACTT-3′), the universal bacterial primers (27f 5′-AGAGTTTGATYMTGGCTCAG-3′; 1492r 5′-GGTTACCTTGTTACGACTT-3′) and newly designed M. sedula msed0966 gene-specific primers msed0966f (5′-TACCTTCCCAATTACTACTCTCAGCAATCTGTCG-3′), and msed0966r (5′-CGTCACCACGATCTCCCC-3′), respectively. The obtained PCR products (Figure S3) were cloned and sequenced, retrieving M. sedula 16S rRNA and msed0966 gene sequences.
Scanning Electron Microscopy
Cells of M. sedula harvested at stationary phase were prepared for electron microscopy by fixing in a solution of 1% (v/v) glutaraldehyde in Na-Cacodylate buffer. Samples were dehydrated in a graded series of ethanol solutions and dried chemically using Hexamethyldisilazan (HMDS). Fixed samples were mounted on aluminum stubs, sputter-coated with Au, and examined with a Hitachi S-4100 SEM (Hitachi, Tokyo, Japan).
Multi-Labeled-Fluorescence in situ Hybridization (MiL-FISH)
Metallosphaera sedula cells were fixed in 2% (v/v) paraformaldehyde (PFA) at room temperature for 1 h, washed three times in distilled water, centrifuged at 10,000 rpm and stored in 50:50, ethanol:PBS (phosphate buffer saline). A 16 S rRNA phylotype specific probe for M. sedula was designed with the software package ARB31 and labeled with 4x Atto488 via Click chemistry (biomers.net GmbH, Ulm, Germany; Table 1). Fixed cells were mounted on 10 well Diagnostica glass slides (Thermo Fisher Scientific Inc. Waltham, USA) and MiL-FISH conducted directly on them as described previously (Schimak et al., 2015; Kölbl et al., 2017; Milojevic et al., 2019b). Briefly, cells were hybridized with 30% (v/v) formamide for 16 h. For experimental positive controls Gramella forsetii strain KT0803 was hybridized with a 4x labeled general bacterial probe EUB338. Positive control for the specificity of the phylotype specific probe M.sedula_174 was given by including M. sedula DSM5348 in all experiments. After hybridization slides were washed for 15 min at 48°C [14–900 mM NaCl, 20 mM Tris-HCl (pH 8), 5 mM EDTA (pH 8), and 0.01% SDS (v/v)] at a stringency adjusted to the formamide concentration used. Cells were counterstained by incubation for 10 min with 10 mg ml−1 DAPI followed by rinsing in distilled water 3 times before CitiFluor (CitiFluor Ltd., London, England) mounting medium was applied to slides with a coverslip. Fluorescence images were taken with an AxioCam Mrm camera mounted on an Axioscope2 epifluorescence microscope (Carl Zeiss AG, Oberkochen, Germany) equipped with F36-525 Alexa 488 (ex, 472/30 nm; em, 520/35 nm) filter cube. Images were recorded with the PC-based AxioVision (release 4.6.3 SP1) imaging software.
Data Availability Statement
All data generated for this study are included in the article/Supplementary Material.
Author Contributions
ZZ, MS, and TM performed experiments and provided editorial input. All authors made extensive contributions to the analysis, acquisition, and interpretation of data provided in this report. All authors reviewed the report and accepted the final version of it.
Funding
This study was supported by the Austrian Science Fund (FWF) within an Elise-Richter Research Program V333 Iron- and Sulfur-oxidizing Machinery of the bioleaching Archaeon Metallosphaera sedula.
Conflict of Interest
The authors declare that the research was conducted in the absence of any commercial or financial relationships that could be construed as a potential conflict of interest.
Acknowledgments
We warmly thank Andreas Klingl and Michaela Stieglmeier (Biocenter LMU Munich, Germany) for the support of with electron microscopy investigations. Christian Koeberl, Ludovic Ferrière, and Brandstätter Franz (Natural History Museum of Vienna, Austria) are deeply acknowledged for providing the NWA 1172 meteorite sample and fruitful discussions. Simon K.-M. R. Rittmann, Christa Schleper, and the other team members of Archaea Biology and Ecogenomics Division (University of Vienna) are greatly appreciated for fruitful discussions and help with low temperature cultivation.
Supplementary Material
The Supplementary Material for this article can be found online at: https://www.frontiersin.org/articles/10.3389/fspas.2020.00037/full#supplementary-material
References
Akanuma, S., Nakajima, Y., Yokobori, S., Kimura, M., Nemoto, N., Mase, T., et al. (2013). Experimental evidence for the thermophilicity of ancestral life. Proc. Natl. Acad. Sci. U.S.A. 110, 11067–11072. doi: 10.1073/pnas.1308215110
Auernik, K. S., and Kelly, R. M. (2008). Identification of components of electron transport chains in the extremely thermoacidophilic crenarchaeon Metallosphaera sedula through iron and sulfur compound oxidation transcriptomes. Appl. Environ. Microbiol. 74, 7723–7732. doi: 10.1128/AEM.01545-08
Auernik, K. S., and Kelly, R. M. (2010a). Physiological versatility of the extremely thermoacidophilic archaeon Metallosphaera sedula supported by transcriptomic analysis of heterotrophic, autotrophic, and mixotrophic growth. Appl. Environ. Microbiol. 76, 931–935. doi: 10.1128/AEM.01336-09
Auernik, K. S., and Kelly, R. M. (2010b). Impact of molecular hydrogen on chalcopyrite bioleaching by the extremely thermoacidophilic archaeon Metallosphaera sedula. Appl. Environ. Microbiol. 76, 2668–2672. doi: 10.1128/AEM.02016-09
Auernik, K. S., Maezato, Y., Blum, P. H., and Kelly, R. M. (2008). The genome sequence of the metal- mobilizing, extremely thermoacidophilic archaeon Metallosphaera sedula provides insights into bioleaching-associated metabolism. Appl. Environ. Microbiol. 74, 682–692. doi: 10.1128/AEM.02019-07
Bada, J. L., Bigham, C., and Miller, S. L. (1994). Impact melting of frozen oceans on the early Earth: implications for the origin of life. Proc. Natl. Acad. Sci. U.S.A. 91, 1248–1250. doi: 10.1073/pnas.91.4.1248
Bada, J. L., and Lazcano, A. (2002). Origin of life. Some like it hot, but not the first biomolecules. Science 296, 1982–1983. doi: 10.1126/science.1069487
Beblo, K., Douki, T., Schmalz, G., Rachel, R., Wirth, R., Huber, H., et al. (2011). Survival of thermophilic and hyperthermophilic microorganisms after exposure to UV-C, ionizing radiation and desiccation. Arch. Microbiol. 193, 797–809. doi: 10.1007/s00203-011-0718-5
Beblo, K., Rabbow, E., Rachel, R., Huber, H., and Rettberg, P. (2009). Tolerance of thermophilic and hyperthermophilic microorganisms to desiccation. Extremophiles 13, 521–531. doi: 10.1007/s00792-009-0239-1
Bell, E., Blake, L. I., Sherry, A., Head, I. M., and Hubert, C. R. J. (2018). Distribution of thermophilic endospores in a temperate estuary indicate that dispersal history structures sediment microbial communities. Environ. Microbiol. 20, 1134–1147. doi: 10.1111/1462-2920.14056
Bell, E., Sherry, A., Pilloni, G., Suárez-Suárez, A., Cramm, M. A., Cueto, G., et al. (2020). Sediment cooling triggers germination and sulfate reduction by heat-resistant thermophilic spore-forming bacteria. Environ. Microbiol. 22, 456–465. doi: 10.1111/1462-2920.14866
Blazevic, A., Albu, M., Mitsche, S., Rittmann, S. K.-M. R., Habler, G., and Milojevic, T. (2019). Biotransformation of scheelite CaWO4 by the extreme thermoacidophile Metallosphaera sedula: tungsten–microbial interface. Front. Microbiol. 10:1492. doi: 10.3389/fmicb.2019.01492
Bulat, S. A. (2016). Microbiology of the subglacial Lake Vostok: first results of borehole-frozen lake water analysis and prospects for searching for lake inhabitants. Philos. Trans. A Math. Phys. Eng. Sci. 374:292. doi: 10.1098/rsta.2014.0292
Bulat, S. A., Alekhina, I. A., Blot, M., Petit, J. R., Angelis, M., Waggenbach, D., et al. (2004). DNA signature of thermophilic bacteria from the aged accretion ice of Lake Vostok, Antarctica: implications for searching for life in extreme icy environments. Int. J. Astrobiol. 3, 1–12. doi: 10.1017/S1473550404001879
Chakraborty, A., Ellefson, E., Li, C., Gittins, D., Brooks, J. M., Bernard, B. B., et al. (2018). Thermophilic endospores associated with migrated thermogenic hydrocarbons in deep Gulf of Mexico marine sediments. ISME J. 12, 1895–1906. doi: 10.1038/s41396-018-0108-y
Chin, J. P., Megaw, J., Magill, C. L., Nowotarski, K., Williams, J. P., Bhaganna, P., et al. (2010). Solutes determine the temperature windows for microbial survival and growth. Proc. Natl. Acad. Sci. U.S.A. 107, 7835–7840. doi: 10.1073/pnas.1000557107
Cockell, C., Cousins, C., Wilkinson, P., Olsson-Francis, K., and Rozitis, B. (2015). Are thermophilic microorganisms active in cold environments? Int. J. Astrobiol. 14, 457–463. doi: 10.1017/S1473550414000433
Cramm, M. A., Chakraborty, A., Li, C., Ruff, S. E., Jørgensen, B. B., and Hubert, C. R. J. (2019). Freezing tolerance of thermophilic bacterial endospores in marine sediments. Front. Microbiol. 10:945. doi: 10.3389/fmicb.2019.00945
Cray, J. A., Russell, J. T., Timson, D. J., Singhal, R. S., and Hallsworth, J. E. (2012). A universal measure of chaotropicity and kosmotropicity. Environ. Microbiol. 15, 287–296. doi: 10.1111/1462-2920.12018
de Rezende, J. R., Kjeldsen, K. U., Hubert, C. R., Finster, K., Loy, A., and Jørgensen, B. B. (2013). Dispersal of thermophilic Desulfotomaculum endospores into Baltic Sea sediments over thousands of years. ISME J. 7, 72–84. doi: 10.1038/ismej.2012.83
Demidov, N. E., and Gilichinsky, D. A. (2009). “Terrestrial permafrost models and analogues of Martian habitats and inhabitants,” in Permafrost Soils, ed R. Margesin (Berlin; Heidelberg: Springer), 334.
Fajardo-Cavazos, P., Schuerger, A. C., and Nicholson, W. L. (2007). Testing interplanetary transfer of bacteria between Earth and Mars as a result of natural impact phenomena and human spaceflight activities. Acta Astronaut. 60, 534–540. doi: 10.1016/j.actaastro.2006.09.018
Gilichinsky, D. A., Wilson, G. S., Friedmann, E. I., McKay, C. P., Sletten, R. S., Rivkina, E. M., et al. (2007). Microbial populations in Antarctic permafrost: biodiversity, state, age, and implication for astrobiology. Astrobiology 7, 275–311. doi: 10.1089/ast.2006.0012
Gura, C., and Rogers, S. O. (2020). Metatranscriptomic and metagenomic analysis of biological diversity in subglacial Lake Vostok (Antarctica). Biology 9:55. doi: 10.3390/biology9030055
Horneck, G., Stöffler, D., Ott, S., Hornemann, U., Cockell, C. S., Moeller, R., et al. (2008). Microbial rock inhabitants survive hypervelocity impacts on Mars-like host planets: first phase of lithopanspermia experimentally tested. Astrobiology 8, 17–44. doi: 10.1089/ast.2007.0134
Huber, G., Spinnler, C., Gambacorta, A., and Stetter, K. O. (1989). Metallosphaera sedula gen. and sp. nov. represents a new genus of aerobic, metal-mobilizing, thermoacidophilic archaebacteria. Syst. Appl. Microbiol. 12, 38–47. doi: 10.1016/S0723-2020(89)80038-4
Huber, H., Huber, G., and Stetter, K. (1985). O. A modified DAPI fluorescence staining procedure suitable for the visualization of lithotrophic bacteria. Syst. Appl. Microbiol. 6, 105–106. doi: 10.1016/S0723-2020(85)80021-7
Huber, R., Stotters, P., Cheminee, J. L., Richnow, H. H., and Stetter, K. O. (1990). Hyperthermophilic archaebacteria within the crater and open-sea plume of erupting Macdonald Seamount. Nature 345, 179–182. doi: 10.1038/345179a0
Hubert, C., Arnosti, C., Brüchert, V., Loy, A., Vandieken, V., and Jørgensen, B. B. (2010). Thermophilic anaerobes in Arctic marine sediments induced to mineralize complex organic matter at high temperature. Environ. Microbiol. 12, 1089–1104. doi: 10.1111/j.1462-2920.2010.02161.x
Hubert, C., Loy, A., Nickel, M., Arnosti, C., Baranyi, C., Brüchert, V., et al. (2009). A constant flux of diverse thermophilic bacteria into the cold Arctic seabed. Science 325, 1541–1544. doi: 10.1126/science.1174012
Kawaguchi, Y., Yokobori, S., Hashimoto, H., Yano, H., Tabata, M., Kawai, H., et al. (2016). Investigation of the interplanetary transfer of microbes in the Tanpopo mission at the exposed facility of the International Space Station. Astrobiology 16, 363–376. doi: 10.1089/ast.2015.1415
Kelly, L. C., Cockell, C. S., Herrera-Belaroussi, A., Piceno, Y., Andersen, G., DeSantis, T., et al. (2011). Bacterial diversity of terrestrial crystalline volcanic rocks, Iceland. Microb. Ecol. 62, 69–79. doi: 10.1007/s00248-011-9864-1
Kelly, L. C., Cockell, C. S., Piceno, Y. M., Andersen, G. L., Thorsteinsson, T., and V., Marteinsson (2010). Bacterial diversity of weathered terrestrial Icelandic volcanic glasses. Microb. Ecol. 60, 740–752. doi: 10.1007/s00248-010-9684-8
Kölbl, D., Pignitter, M., Somoza, V., Schimak, M. P., Strbak, O., Blazevic, A., et al. (2017). Exploring fingerprints of the extreme thermoacidophile Metallosphaera sedula grown on synthetic Martian regolith materials as the sole energy sources. Front. Microbiol. 8:1918. doi: 10.3389/fmicb.2017.01918
Lavire, C., Normand, P., Alekhina, I., Bulat, S., Prieur, D., Birrien, J. L., et al. (2006). Presence of Hydrogenophilus thermoluteolus DNA in accretion ice in the subglacial Lake Vostok, Antarctica, assessed using rrs, cbb and hox. Environ. Microbiol. 8, 2106–2114. doi: 10.1111/j.1462-2920.2006.01087.x
Maezato, Y., Johnson, T., McCarthy, S., Dana, K., and Blum, P. (2012). Metal resistance and lithoautotrophy in the extreme thermoacidophile Metallosphaera sedula. J. Bacteriol. 194, 6856–6863. doi: 10.1128/JB.01413-12
Marchant, R., Banat, I. M., and Franzetti, A. (2011). “Thermophilic bacteria in cool soils: metabolic activity and mechanisms of dispersal,” in Biogeography of Microscopic Organisms Is Everything Small Everywhere? ed D. Fontaneto (Cambridge: Cambridge University Press), 43–57.
Marchant, R., Banat, I. M., Rahman, T. J., and Berzano, M. (2002). The frequency and characteristics of highly thermophilic bacteria in cool soil environments. Environ. Microbiol. 4, 595–602. doi: 10.1046/j.1462-2920.2002.00344.x
Marchant, R., Franzetti, A., Pavlostathis, S. G., Tas, D. O., Erdbrugger, I., Unyayar, A., et al. (2008). Thermophilic bacteria in cool temperate soils: are they metabolically active or continually added by global atmospheric transport? Appl. Microbiol. Biotechnol. 78, 841–852. doi: 10.1007/s00253-008-1372-y
McCarthy, S., Ai, C., Wheaton, G., Tevatia, R., Eckrich, V., Kelly, R., et al. (2014). Role of anarchaeal PitA transporter in the copper and arsenic resistance of Metallosphaera sedula, an extreme thermoacidophile. J. Bacteriol. 196, 3562–3570. doi: 10.1128/JB.01707-14
Mileikowsky, C., Cucinotta, F. A., Wilson, J. W., Gladman, B., Horneck, G., Lindegren, L., et al. (2000). Natural transfer of viable microbes in space: I. from Mars to Earth and Earth to Mars. Icarus 145, 391–427. doi: 10.1006/icar.1999.6317
Milojevic, T., Albu, M., Blazevic, A., Gumerova, N., Konrad, L., and Cyran, N. (2019a). Nanoscale zungsten-microbial interface of the metal immobilizing thermoacidophilic archaeon Metallosphaera sedula cultivated with tungsten polyoxometalate. Front. Microbiol. 10:1267. doi: 10.3389/fmicb.2019.01267
Milojevic, T., Kölbl, D., Ferrière, L., Albu, M., Kish, A., Flemming, R. L., et al. (2019b). Exploring the microbial biotransformation of extraterrestrial material on nanometer scale. Sci. Rep. 9:18028. doi: 10.1038/s41598-019-54482-7
Mironov, V. A., Rivkina, E. M., Vishnivetskaya, T. A., Shcherbakova, V. A., and Gilichinsky, D. A. (2010). Thermophilic Life Inside the Permafrost. Legue, TX: AbSciCon.
Mora, M., Bellack, A., Ugele, M., Hopf, M., and Wirth, R. (2014). The temperature gradient forming device: an accessory unit for normal light microscopes to study the biology of hyperthermophilic microorganisms. Appl. Environ. Microbiol. 80, 4764–4770. doi: 10.1128/AEM.00984-14
Mukherjee, A., Wheaton, G. H., Blum, P. H., and Kelly, R. M. (2012). Uranium extremophily is an adaptive, rather than intrinsic, feature for extremely thermoacidophilic Metallosphaera species. Proc. Natl. Acad. Sci. U.S.A. 109, 16702–16707. doi: 10.1073/pnas.1210904109
Müller, A. L., de Rezende, J. R., Hubert, C. R., Kjeldsen, K. U., Lagkouvardos, I., Berry, D., et al. (2014). Endospores of thermophilic bacteria as tracers of microbial dispersal by ocean currents. ISME J. 8, 1153–1165. doi: 10.1038/ismej.2013.225
Nicholson, W. L. (2009). Ancient micronauts: interplanetary transport of microbes by cosmic impacts. Trends Microbiol. 17, 243–250. doi: 10.1016/j.tim.2009.03.004
Onofri, S., de la Torre, R., de Vera, J. P., Ott, S., Zucconi, L., Selbmann, L., et al. (2012). Survival of rock-colonizing organisms after 1.5 years in outer space. Astrobiology 12, 508–516. doi: 10.1089/ast.2011.0736
Papale, M., Lo Giudice, A., Conte, A., Rizzo, C., Rappazzo, A. C., Maimone, G., et al. (2019). Microbial assemblages in pressurized Antarctic brine pockets (Tarn Flat, Northern Victoria Land): a hotspot of biodiversity and activity. Microorganisms 7:333. doi: 10.3390/microorganisms7090333
Peeples, T. L., and Kelly, R. M. (1995). Bioenergetic response of the extreme thermoacidophile Metallosphaera sedula to thermal and nutritional stress. Appl. Environ. Microbiol. 61, 2314–2321. doi: 10.1128/AEM.61.6.2314-2321.1995
Rahman, T. J., Marchant, R., and Banat, I. M. (2004). Distribution and molecular investigation of highly thermophilic bacteria associated with cool soil environments. Biochem. Soc. Trans. 32, 209–213. doi: 10.1042/bst0320209
Robador, A., Müller, A. L., Sawicka, J. E., Berry, D., Hubert, C. R. A., et al. (2016). Activity and community structures of sulfate-reducing microorganisms in polar, temperate and tropical marine sediments. ISME J. 10, 796–809. doi: 10.1038/ismej.2015.157
Russell, S., Zipfel, J., Grossman, J., and Grady, M. (2002). The meteoritical bulletin No. 86. Meteorit. Planet. Sci. 37, 157–184. doi: 10.1111/j.1945-5100.2002.tb00913.x
Schimak, M. P., Kleiner, M., Wetzel, S., Liebeke, M., Dubilier, N., and Fuchs, B. M. (2015). MiL-FISH: Multilabeled oligonucleotides for fluorescence in situ hybridization improve visualization of bacterial cells. Appl. Environ. Microbiol. 82, 62–70. doi: 10.1128/AEM.02776-15
Shcherbakova, V., Rivkina, E., Pecheritsyna, S., Laurinavichius, K., Suzina, N., and Gilichinsky, D. A. (2011). Methanobacterium arcticum sp. nov., a methanogenic archaeon from Holocene Arctic permafrost. Int. J. Syst. Evol. Microbiol. 61, 144–147. doi: 10.1099/ijs.0.021311-0
Stetter, K. O. (2006). Hyperthermophiles in the history of life. Philos. Trans. R. Soc. Lond. B. Biol. Sci. 361, 1837–1842. doi: 10.1098/rstb.2006.1907
Vandieken, V., Knoblauch, C., and Jørgensen, B. B. (2006). Desulfotomaculum arcticum sp. nov., a novel spore-forming, moderately thermophilic, sulfate-reducing bacterium isolated from permanently cold fjord sediment of Svalbard. Int. J. Sys. Evol. Microbiol. 56, 687–690. doi: 10.1099/ijs.0.64058-0
Wächtershäuser, G. (1992). Groundworks for an evolutionary biochemistry: the iron-sulphur world. Prog. Biophys. Mol. Biol. 58, 85–201. doi: 10.1016/0079-6107(92)90022-X
Wiegel, J. (1990). Temperature spans for growth: hypothesis and discussion. FEMS Microbiol. Rev. 75, 155–170. doi: 10.1111/j.1574-6968.1990.tb04092.x
Wiegel, J. (2002). “Thermophiles: anaerobic alkalithermophiles,” in Encyclopedia of Environmental Microbiology, ed G. Bitton (New York, NY: John Wiley and Sons Inc), 3127–3140.
Wirth, R. (2017). Colonization of black smokers by hyperthermophilic microorganisms. Trends Microbiol. 2, 92–99. doi: 10.1016/j.tim.2016.11.002
Keywords: Metallosphaera sedula, meteorite, low temperature, cold environments, cryopreservation, thermophiles, archaea
Citation: Milojevic T, Zebec Z and Schimak MP (2020) Cultivation With Powdered Meteorite (NWA 1172) as the Substrate Enhances Low-Temperature Preservation of the Extreme Thermoacidophile Metallosphaera sedula. Front. Astron. Space Sci. 7:37. doi: 10.3389/fspas.2020.00037
Received: 18 April 2020; Accepted: 28 May 2020;
Published: 08 July 2020.
Edited by:
Daniela Billi, University of Rome Tor Vergata, ItalyReviewed by:
John E. Hallsworth, Queen's University Belfast, United KingdomJan Hendrik Bredehöft, University of Bremen, Germany
Copyright © 2020 Milojevic, Zebec and Schimak. This is an open-access article distributed under the terms of the Creative Commons Attribution License (CC BY). The use, distribution or reproduction in other forums is permitted, provided the original author(s) and the copyright owner(s) are credited and that the original publication in this journal is cited, in accordance with accepted academic practice. No use, distribution or reproduction is permitted which does not comply with these terms.
*Correspondence: Tetyana Milojevic, dGV0eWFuYS5taWxvamV2aWMmI3gwMDA0MDt1bml2aWUuYWMuYXQ=