- Department of Earth and Planetary Sciences, University of California, Davis, Davis, CA, United States
Urey (1952a,b, 1956) introduced the Urey reaction to explain the origin of carbonates in the continental crust. The reaction extracts CO2 from the atmosphere in acid rain that reacts with calcium silicates, the products are transported to the oceans where organic and inorganic processes results in the deposition of calcium carbonates. The origin of the CO2 can be (1) the early atmosphere and (2) the mantle. The reaction removes almost all the atmospheric CO2 that survived the formation of the Earth. If surface volcanism introduces more CO2 than is lost by subduction, the CO2 entering the atmosphere will be lost to the continental crust by the Urey reaction. Studies of carbon fluxes between surface reservoirs have demonstrated the importance of the Urey reaction in controlling the concentration of CO2 in the atmosphere. At the present time the only surface reservoir with a large mass of carbon is the continental crust. In order to quantify the rate at which the Urey reaction removes CO2 from the atmosphere we utilize data from the Paleocene-Eocene thermal maximum (PETM). This was a period of elevated global temperatures (ΔT = 4 − 5°C) at 56 Ma attributed to a pulse of volcanism and associated CO2 in the north Atlantic. We utilize the decay time of this thermal anomaly to quantify the rate at which CO2 is extracted from the atmosphere by the Urey reaction.
1. Introduction
We first give a brief introduction to the distribution of carbon in the Earth. In doing this we will utilize the concept of carbon reservoirs. In some cases the definition of a carbon reservoir is well-defined. The Earth's atmosphere is an example. Carbon in the atmosphere is primarily in CO2 and its concentration is relatively uniform. In other cases the definition of a carbon reservoir is arbitrary. The Earth's mantle is an example. The relatively uniform composition of mid-ocean ridge basalts (including CO2) is taken as evidence of a near-uniform upper mantle reservoir. However, the variability of ocean-island and hot-spot basalts is evidence for a complex heterogeneous lower-mantle.
Houghton (2007) considered the present distribution of carbon in near-surface reservoirs including the atmosphere, oceans, ocean sediments, vegetation and soil, and fossil fuels. However, the near-surface reservoirs contain only a small fraction of the carbon in the Earth. The major reservoirs for carbon at the present are the core, the mantle, and the continental crust. It is generally accepted that a large fraction of the carbon resides in the core reservoir. Both during early core formation and accretion, and during core formation after the moon-forming giant impact, carbon in the solid Earth was largely partitioned into the core. Dasgupta (2013) estimates that the mass of carbon in the core is Gt (1 Gt = 1012 kg) but there is certainly a large error bar. We suggest that a reasonable estimate for the present mass of carbon in the core is Gt.
A number of authors have studied the global carbon flux at mid-ocean ridges. These values can be used to estimate the composition of carbon in the mantle source region. Michael and Graham (2015) give a mass fraction and Rosenthal et al. (2015) give a value . We suggest that a reasonable estimate for the composition of carbon in this source region is . Assuming the entire mantle to have this concentration, the mass of carbon in the mantle is Gt.
The third large reservoir for carbon at the present time is the continental crust. About 1% of the carbon in the Earth is in the continental crust; a large fraction of this carbon is in carbonates (limestones, marble, etc.) Wedepohl (1995) has given a comprehensive review of the composition of the continental crust with an emphasis on carbon. He gives a total carbon mass in the continental crust Gt. Of this total he attributes about 80% to sediments, almost entirely in carbonates. Hayes and Waldbauer (2006) have reviewed the literature on carbon in the crust and have given a range of masses and gave a preferred total carbon mass Gt. In this paper we will accept the value given by Wedepohl (1995) as a representative value for the present mass of carbon in the continental crust Gt, but suggest that a reasonable range of acceptable values is – 2 × 108 Gt.
A list of the estimated present masses of carbon in the reservoirs we consider is given in Table 1. The estimated total mass of carbon in all of the reservoirs is cM = 4.68 × 109 Gt. With the mass of the Earth, 5.97 × 1024 kg, the concentration of carbon in the bulk Earth by mass is about 0.1%. These values are consistent with previous estimates (Dasgupta, 2013; DePaolo, 2015). We do not give error bars because the uncertainties of some values are difficult to specify.
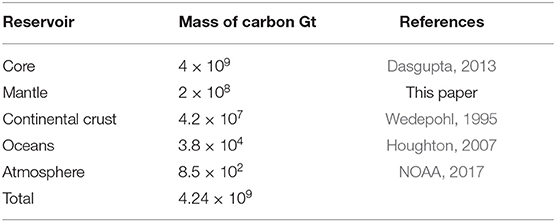
Table 1. Masses of carbon in the Earth's carbon reservoirs considered in this paper (1 Gt = 1012 kg).
A large fraction of the carbon in the surface reservoirs is in the continental crust, ~99.99%. Much of this carbon is in the carbonates (limestones, marbles). Some 20–30% of the sediments in the continental crust are carbonates, independent of age (Mackenzie and Morse, 1992). Urey (1952a,b, 1956) proposed that a reaction between atmospheric CO2 and calcium silicate was a major source of carbon in the continental crust. This reaction is now known as the Urey reaction. The principal purpose of this paper is to evaluate the role of the Urey reaction in terms of our present knowledge of the distribution of carbon in the Earth.
Two sources of the carbon in the continental crust are available. The first of these is the atmosphere. Today the atmosphere has very little carbon in it, however the atmosphere of Venus has a large mass of carbon as CO2. It is quite possible that the early Earth also had a very high concentration of CO2, the Urey reaction could have removed a significant fraction of this carbon to the continental crust. The second source of the carbon in the continental crust is the mantle. If the flux of carbon out of the mantle in surface volcanism exceeds the loss of carbon in subduction, the mantle could have lost carbon to the continental crust. The Urey reaction could have played an important role in this process.
2. The Urey Reaction
Urey (1952a,b, 1956) introduced the Urey reaction to explain the origin of calcium carbonates (limestones, marbles) in the continental crust. The basic reaction combines atmospheric CO2 with a calcium silicate to generate a calcium carbonate plus silica. In order to include the role of acid rain in the process we write the Urey reaction in the form
The aqueous products are removed by calcium carbonate precipitation. Carbon dioxide (carbonic acid) in acid rain dissolves calcium silicate (wollastonite) sediments to give calcium, bicarbonate, and silica. The resulting calcium and bicarbonate ions flow in rivers to the oceans where either organic or inorganic precipitation produces the calcium carbonate. Blättler and Higgins (2017) have considered Urey's carbonate-silicate cycle in some detail utilizing measured calcium isotope ratios.
The role of the Urey reaction in maintaining a low concentration of CO2 in the Earth's atmosphere was explicitly stated by Urey (1952b). “As carbon dioxide was formed it reacted with silicates to form limestone. Of course the silicates may have been a variety of minerals but the pressure of CO2 was always kept at a low level by this reaction or similar reactions just as it is now.”
Although the mass of carbon in the atmosphere today is small (~850 Gt), the mass may have been much higher in the past. One of the major differences between Venus and the Earth is atmospheric composition. The atmospheric pressure on Venus is about a factor of 100 greater than the atmospheric pressure on Earth and is 96% carbon dioxide. The mass of carbon in the Venus atmosphere is Gt. Scaling the atmospheric carbon masses to the overall masses of Venus and the Earth gives an estimate of the mass of carbon in the early atmosphere of the Earth. The estimated value is Gt (Kasting and Ackerman, 1986).
Another major difference between the Earth and Venus is the giant impact that is hypothesized to have been responsible for the origin of the moon. During the final stages of the Earth's accretion, a collision between the Earth and an astronomical body about the size of Mars occurred 20–100 Myrs after the origin of the solar system. A detailed review of the influence of this collision on the first several hundred million years of Earth evolution has been given by Zahnle et al. (2007). The intensity of the collision melted and vaporized significant fractions of the Earth and the incoming body. Some fraction of the incoming body (and some fraction of the Earth) subsequently accreted and cooled to form Earth's moon. It is estimated that the hot silicate atmosphere cooled and condensed over about a thousand years. A global magma ocean cooled and solidified much more slowly, possibly over tens of millions of years. Sleep and Zahnle (2001) and Sleep et al. (2011, 2014) have proposed that a substantial fraction of the CO2 in the atmosphere was absorbed by the solid surface crust of the global magma ocean. Episodic foundering subduction of this dense crust transported the carbon into the Earth's mantle, removing CO2 from the atmosphere.
An alternative to the mechanism proposed by Sleep and Zahnle (2001) is that carbon was directly transferred to the mantle during accretion (Li et al., 2016) or that carbon was transferred by tectonic processes during the Hadean. Shaw (2008) has provided a comprehensive review of CO2 evolution in the Hadean to the early Proterzoic.
An important question concerning carbon is the origin of the carbon in the continental crust. One hypothesis is that they were extracted from the atmosphere by the Urey reaction relatively early in the Earth's history. This hypothesis was put forward by Kramers (2002) and by Lowe and Tice (2004). It should be noted that the potential mass of carbon in the early atmosphere, 1.57 × 108 Gt, is substantially larger than the estimated present mass of carbon in the continental crust Gt. Thus, a substantial fraction of the carbon in the continental crust could have been transferred from the atmosphere.
In order for the Urey reaction to extract CO2 from the atmosphere the early Earth must have had continental crust in order to generate surface deposits of calcium silicates. In addition the Earth must of had oceans in order for the acid rain to catalyze the Urey reaction between atmospheric CO2 and the surface deposits of calcium silicates. Little data is available for timing the initiation of the extraction of CO2 from the atmosphere. We will assume that the process began at a time t0 after the solidification of the magma ocean at about 4.5 Ga. We further assume that the Urey reaction extracted carbon from the atmosphere at a constant rate until the concentration of CO2 in the atmosphere was reduced to a very low level. The rate of loss of carbon from the atmosphere is given by
We require at t = t0 and obtain
This transport is completed at t = τ when where
Taking Gt we give the dependence of the carbon flux on the flux time τ − t0 in Figure 1. Combining Equations (3) and (4) we find the time dependence of the mass of carbon in the atmosphere
for the period t0 < t < t0 + τ. For t0 + τ < t < t0 + tp, we have added to the continental crust.
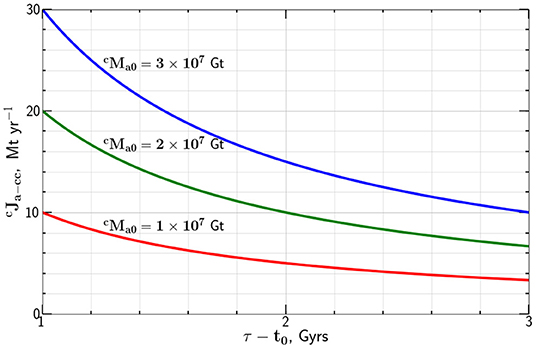
Figure 1. Dependence of the constant mass flux of carbon from the atmosphere to the continental crust required to transfer the mass of carbon 1, 2, and 3 × 107 Gt to the continental crust in a time τ − t0.
Again taking Gt we give the mass of carbon in the continental crust as a function of time t in Figure 2 for t0 = 1 Gyr and τ = 1 and 3 Gyr. Carbon is lost from the atmosphere to the continental crust after plate tectonics provides the calcium silicate required for the Urey reaction to proceed.
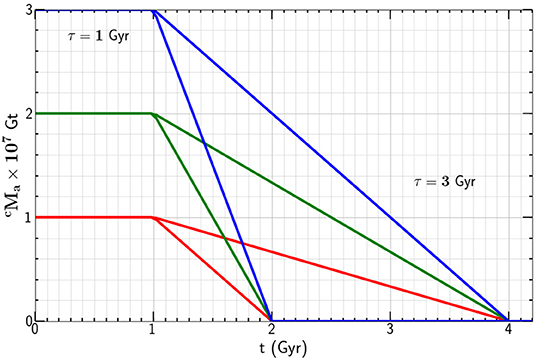
Figure 2. Dependence of the mass of carbon in the atmosphere on time t for t0 = 1 Gyr, τ = 1and 3 Gyr, and Gt.
In discussing the role of the Urey reaction we focus our attention on its role in the generation of carbonate sediments in the continental crust. Above we discussed how it could be directly responsible for the extraction of primordial CO2 from the atmosphere. We now turn our attention to its indirect role in the addition of carbon from the mantle into the continental crust. Carbon is extracted from the mantle by volcanism and is returned to the mantle by subduction. If the rate of addition exceeds the rate of loss then the difference may be responsible for the origin of a large fraction of the carbon in the continental crust. Order of magnitude estimates of this process given by Hayes and Waldbauer (2006) demonstrated the plausibility of this mechanism.
A comprehensive model for the variability of atmosphere CO2 over Phanerozoic times has been given by Berner and Kothavala (2001). This model, GEOCARB III, is complex and involves both organic and inorganic processes. Transport of carbon between the atmosphere, oceans, and continental crust is quantified on the million year time scale. The balance is dominated by the exchange of carbon between carbonates in the continental crust and carbon in the surficial reservoirs (oceans and atmosphere) and organic carbon (Berner and Caldeira, 1997). This exchange occurs through the Urey reaction given in Equation (1). When erosion is high, the Urey reaction extracts CO2 from the atmosphere adding carbonates to the continental crust. High erosion rates are associated with low sea level and large continental areas. When erosion is low, the Urey reaction operates in the opposite direction (from right to left in Equation 1) with carbonates decomposing to give CO2. An example of this metamorphic process is the subduction of carbonate sediments and the generation and return of CO2 to the atmosphere in subduction zone volcanics (Frezzotti et al., 2011).
We will next provide estimates for the rate at which surface volcanism transfers carbon from the mantle to the surface reservoirs. Volumetrically, surface volcanism is dominated by mid-ocean ridge volcanism. A number of authors have studied the global carbon flux from the mantle at mid-ocean ridges. Some studies involve direct measurements of CO2 concentrations and others utilize correlations with other elements, such as barium. Hayes and Waldbauer (2006) summarized the early literature and gave a mid-ocean ridge global carbon flux Mtyr−1. Dasgupta and Hirschmann (2010) summarized the available data and gave a global carbon flux Mtyr−1. Michael and Graham (2015) obtained an estimate of the carbon flux from mid-ocean ridges using measurements of CO2/Ba ratios with published compilations of trace elements. They suggest that the global flux of carbon out of the mantle at mid-ocean ridges is Mtyr−1. Rosenthal et al. (2015) used CO2/Ba and CO2/Nb systematics and conclude that the global flux of carbon is Mtyr−1. Chavrit et al. (2014) carried out CO2 vesicularity measurements on some 400 mid-ocean ridge glasses. These results were interpreted to give a global carbon flux Mtyr−1. Since not all CO2 would be expected to be in cavities, the actual carbon flux would be expected to be somewhat higher. Based on these previous studies we suggest that a reasonable choice for the carbon flux from the mantle at mid-ocean ridges is Mtyr−1.
Flux estimates of carbon in ocean island basalts and hot spot volcanics have been reviewed by Hayes and Waldbauer (2006). Volumetrically this volcanism is about 10% of the mid-ocean ridges volcanism but some of this volcanism may be tapping a primitive lower mantle reservoir with higher concentrations of carbon. Considering the uncertainties we will neglect this source of carbon to the atmosphere in our modeling.
Just as carbon is lost from the mantle at mid-ocean ridges, carbon is returned to the mantle at subduction zones. A detailed study of carbon fluxes at subduction zones has been carried out by Kelemen and Manning (2015). These authors suggest that the downward flux of carbon at global subduction zones is 53 ± 13 Mtyr−1. However, a substantial fraction of this carbon never makes it to the mantle. Carbon is returned to the oceans and atmosphere in subduction zone volcanism. Kelemen and Manning (2015) estimate the loss of carbon due to subduction zone volcanics is in the range 30 ± 12 Mtyr−1. Taking the difference of these values, the present subduction flux of carbon to the mantle is Mtyr−1. Dasgupta and Hirschmann (2010) have also provided a comprehensive study of carbon subduction and conclude that Mtyr−1 reach the mantle. An earlier review of carbon subduction was given by Hayes and Waldbauer (2006). These authors concluded that the input flux of carbon is 36 ± 12 Mtyr−1 and that the flux to the mantle is Mtyr−1.
We obtain an estimate for the net flux of carbon from the mantle required to produce a large mass of carbon in the continental crust assuming that this flux has been a constant during the period tp − t0 to tp (present time). We use the relation
Taking the carbon mass transported from the mantle to the continental crust Gt we give the dependence of the carbon flux on the flux time tp − t0 in Figure 3. The results are essentially identical to those given in Figure 1 for the transfer from the atmosphere. This is not surprising since Equation (6) is basically the same as Equation (3).
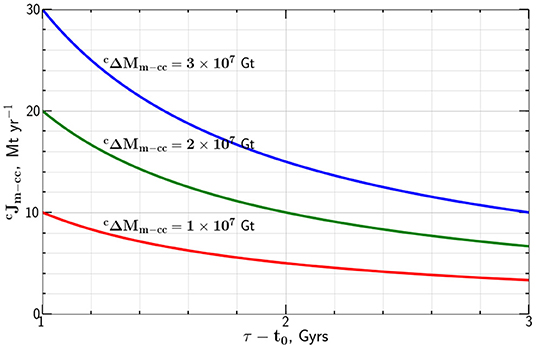
Figure 3. Dependence of the present mass flux of carbon from the mantle to the continental crust , required to generate mass of carbon in the continental crust, in a time tp − t0, on this time difference.
The time dependence of the mass transferred from the mantle to the continental crust is given by
Again taking Gt we give the dependence of the mass of carbon added to the continental crust as a function of time t in Figure 4 for t0 = 1.4 Gyr.
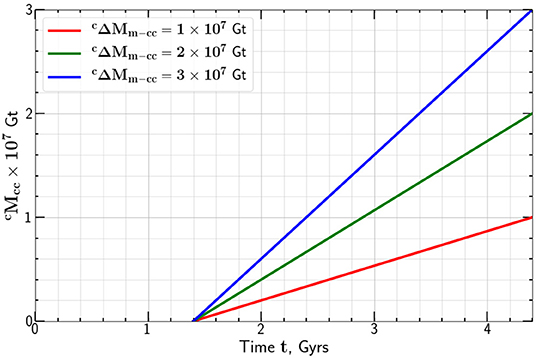
Figure 4. Dependence of the mass of carbon added to the continental crust on time, t for t0 = 1.4 Gyrs.
When excess CO2 in the atmosphere has been depleted by the Urey reaction an approximate steady state balance is established between the volcanic input of CO2 and extraction by the Urey reaction. It has been argued by many authors that this balance is controlled by the Urey reaction. We approximate this balance by the relation
where is the rate of volcanic input of carbon into the atmosphere. We assume that the extraction rate is proportional to the mass of carbon in the atmosphere . The rate at which the Urey reaction rate processes carbon τa−cc takes account of the rate at which acid rain can interact with calcium silicate sediments, clearly τa−cc can be a function of time.
3. PETM
As a further test of our approach to the use of the Urey reaction to explain variations in atmospheric CO2 concentrations we will consider the Paleocene-Eocene thermal maximum (PETM). This was a period of elevated global temperatures that occurred at about 56 Ma. The elevated temperatures are attributed to increased concentrations of CO2 and possibly methane in the atmosphere. The maximum increase in global surface temperatures is in the range 4−5°C and the period of excess temperatures lasted some 106 yrs. The occurrence of this period of high atmospheric CO2 and temperatures was first documented by Kennett and Stott (1991) from an observation of large carbon and oxygen isotope ratios in an ocean drilling core.
A comprehensive review of the subsequent studies of this event was given by McInerney and Wing (2011). By the time this review paper was published some 400 research papers on a wide variety of aspects of PETM were included. The onset of the period of high atmosphere CO2 and temperature was dated at 56.3 Ma, the onset lasted <10 ka, and the subsequent decay lasted some 120–220 ka. Observational data used to constrain the structure of the thermal pulse involves a variety of isotope measurements in drilling cores. One example is the temporal dependence of the observed δ13C anomaly in a drilling core (Cui et al., 2011). A second example is the use of boron isotope data as a proxy for seawater (Gutjahr et al., 2017). Jones et al. (2013) have carried out a detailed study of the warming associated with the PETM. They conclude that the global mean surface anomaly is in the range 4–5°C.
Several sources of the carbon associated with the PETM have been proposed. These include methane clathrates and melting of permafrost. Storey et al. (2007) have made a very strong case for associating the PETM with the flood basalt volcanism resulting from the opening of the north Atlantic. This volcanism is documented in east Greenland and the Faroe Islands. The earliest of these flood basalts have an age of 56.1±0.5 Ma. We support the hypothesis that a substantial fraction of the carbon responsible for the PETM was CO2 entering the atmosphere from these flood basalts.
Studies of isotopic anomalies associated with the PETM systematically show a rapid increase at about 55.8 Ma with a subsequent decay of the anomaly over about 105 yrs. There is considerable scatter between drill sites. As an example of an isotopic anomaly we give data from the Deep Sea Drilling Project (DSDP) site 401 in the northeast Atlantic Ocean (Gutjahr et al., 2017). A strong negative carbon isotope anomaly was found in foraminifera Morozovella subbotinae. The observed δ13C anomaly is given as a function of time tPETM relative to the onset of the anomaly in Figure 5.
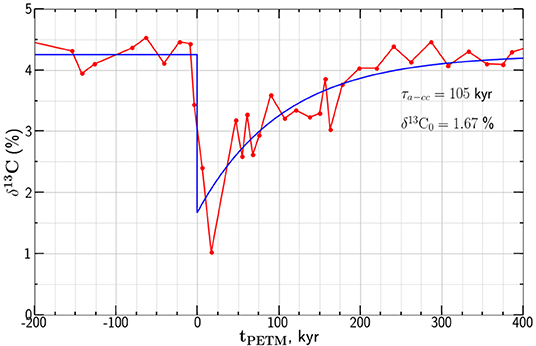
Figure 5. An example of the carbon isotope anomaly associated with the PETM. The dependence of the δ13C values on depositional time tPETM relative to the onset of the anomaly are given. The data are from the DSDP site 401 with the carbon in foraminifera M. subbotinae (Gutjahr et al., 2017). Also shown is the least squares best fit of our relaxation model from Equation (12) with relaxation time τa−cc = 105 kyr and .
Gutjahr et al. (2017) have carried out an extensive modeling study using the available isotope data to provide estimates for the carbon generated during the PETM. We will utilize these values and take the total carbon added to the Earth's surface to be 12,000 Gt. Before and after the PETM we take the background carbon mass in the atmosphere to be Gt and the peak mass of carbon in the atmosphere during the PETM to be Gt.
We now carry out an approximate analysis of the decay of the PETM due to the loss of CO2 from the atmosphere by the Urey reaction. We extend the balance given in Equation (8) to include the transient removal of carbon from the atmosphere and write
From Equation (8) the background mass of carbon in the atmosphere is given by
We prescribe an initial mass of carbon in the atmosphere at , with and solve Equation (10) taking τa−cc to be constant with the result
The excess mass of carbon in the atmosphere decays exponentially as the Urey reaction extracts carbon from the atmosphere.
In order to estimate the value of the characteristic decay time τa−cc we assume that the negative carbon isotope anomaly is proportional to the mass of carbon in the atmosphere and write Equation (11) as
where is the background isotope ratio before and after PETM and is the minimum isotope ratio prescribed at t = 0. Taking the dependence of δ13C on tPETM from Equation (12) is compared with the isotope anomaly values given in Figure 5. The least squares fit of the exponential decay to the data points in given taking τa−cc = 105 kyr and .
We next use this value of τa−cc to obtain the dependence of atmosphere carbon mass on time during PETM based on the model dependence given in Equation (11). Taking the values Gt and Gt as given by Gutjahr et al. (2017) with τa−cc = 105 kyr the model results are given in Figure 6.
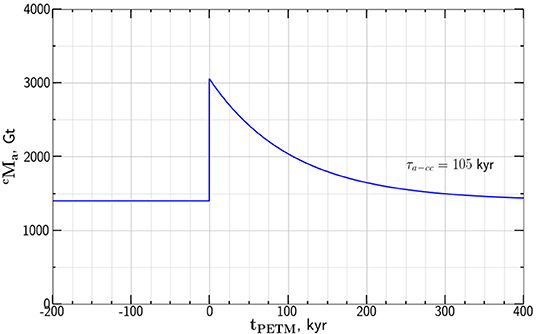
Figure 6. Dependence of the atmosphere carbon mass values for the PETM anomaly on time tPETM relative to the onset of the anomaly. The values are from our relaxation model give in Equation (11) with Gt and Gt.
We now return to Equation (10). This result relates the background atmospheric carbon mass to the background rate of volcanic input of CO2 carbon into the atmosphere and the Urey decay time τa−cc. During the PETM we have taken the background carbon mass Gt. Taking τa−cc = 105 kyr we find from Equation (12) that Mtyr−1. This is an independent determination of the volcanic flux of carbon into the atmosphere. In Figure 4 we gave volcanic the flux required to generate a specific mass of carbon in the continental crust
4. Discussion
Urey (1952a) proposed the Urey reaction, Equation (1) to explain the origin of carbonates in the continental crust. He argued that the reaction would essentially remove all CO2 from the atmosphere. It is now accepted that in analogy to Venus, there may have been a large mass of carbon in the Earth's early atmosphere, as much as 108 Gt. However, only a fraction of this may have survived the moon forming impact. We give a very simplified model for the extraction of carbon from the atmosphere to the continental crust, taking the extraction rate to be constant. There are basically no constraints on the variation of this rate with time. If a significant fraction of the carbon in the continental crust was extracted from the atmosphere, it is likely that it occurred early in Earth's history as illustrated in Figure 1.
The Urey reaction also controls the equilibrium mass of carbon in the atmosphere after the removal of any large initial concentration. The input of carbon to the atmosphere is from volcanism and we show that the equilibrium mass of carbon in the atmosphere is proportional to the rate of volcanic injection divided by a characteristic Urey time τu. We quantify the value of τu by studying the observed relaxation of the Paleocene-Eocene thermal maximum, which occurred at 56 Ma, and the relaxation time is about yrs.
We also give a simplified model for the extraction of carbon from the atmosphere to the continental crust. If the volcanic loss of carbon from the mantle by volcanism exceeds the return of carbon by subduction, the difference is added to the continental crust. If the volcanic carbon enters the oceans organic precipitation creates carbonates. If the volcanic carbon enters the atmosphere it enters the continental crust by the Urey reaction. Current estimates of carbon fluxes from and to the mantle are sufficient to have produced all the carbon in the continental crust. At the present time it is not possible to quantify the relative importance of carbon addition to the continental crust from the early atmosphere and the mantle.
We have addressed two major questions concerning carbon in the atmosphere in this paper. The first is the origin of the carbon in the continental crust. We conclude that it is possible the carbon could have been extracted either from the early atmosphere or from the mantle over a longer period of time. Studies of the concentration of carbon in the atmosphere and continental crust over geologic time are required and should receive a high priority.
The second question we have addressed is the relaxation of injections of carbon into the atmosphere back to equilibrium values. We quantify this by studying the Paleocene-Eocene thermal maximum (PETM). This has obvious implications for the recovery from the process of anthropogenic injection of carbon into the atmosphere. We find the relaxation time to be about 50,000 yrs.
Data Availability Statement
All datasets generated for this study are included in the manuscript/supplementary files.
Author Contributions
All authors listed have made a substantial, direct and intellectual contribution to the work, and approved it for publication.
Conflict of Interest
The authors declare that the research was conducted in the absence of any commercial or financial relationships that could be construed as a potential conflict of interest.
Acknowledgments
The authors would like to acknowledge financial support from the Alfred P. Sloan Foundation under Grant G-2818-10087 through the Deep Carbon Observatory.
References
Berner, R. A., and Caldeira, K. (1997). The need for mass balance and feedback in the geochemical carbon cycle. Geology 25, 955–956.
Berner, R. A., and Kothavala, Z. (2001). GEOCARB III: a revised model of atmospheric CO2 over phanerozoic time. Am. J. Sci. 301, 182–204. doi: 10.2475/ajs.301.2.182
Blättler, C. L., and Higgins, J. A. (2017). Testing Urey's carbonate–silicate cycle using the calcium isotopic composition of sedimentary carbonates. Earth Planet. Sci. Lett. 479, 241–251. doi: 10.1016/j.epsl.2017.09.033
Chavrit, D., Humler, E., and Grasset, O. (2014). Mapping modern CO2 fluxes and mantle carbon content all along the mid-ocean ridge system. Earth Planet. Sci. Lett. 387, 229–239. doi: 10.1016/j.epsl.2013.11.036
Cui, Y., Kump, L. R., Ridgwell, A. J., Charles, A. J., Junium, C. K., Diefendorf, A. F., et al. (2011). Slow release of fossil carbon during the Palaeocene–Eocene thermal maximum. Nat. Geosci. 4, 481–485. doi: 10.1038/ngeo1179
Dasgupta, R. (2013). Ingassing, storage, and outgassing of terrestrial carbon through geologic time. Rev. Miner. Geochem. 75, 183–229. doi: 10.2138/rmg.2013.75.7
Dasgupta, R., and Hirschmann, M. M. (2010). The deep carbon cycle and melting in Earth's interior. Earth Planet. Sci. Lett. 298, 1–13. doi: 10.1016/j.epsl.2010.06.039
DePaolo, D. J. (2015). Sustainable carbon emissions: the geologic perspective. MRS Energy Sustain. 2:E9. doi: 10.1557/mre.2015.10
Frezzotti, M. L., Selverstone, J., Sharp, Z. D., and Compagnoni, R. (2011). Carbonate dissolution during subduction revealed by diamond-bearing rocks from the Alps. Nat. Geosci. 4, 703–706. doi: 10.1038/ngeo1246
Gutjahr, M., Ridgwell, A., Sexton, P. F., Anagnostou, E., Pearson, P. N., Pälike, H., et al. (2017). Very large release of mostly volcanic carbon during the Palaeocene–Eocene thermal maximum. Nature 548, 573–577. doi: 10.1038/nature23646
Hayes, J. M., and Waldbauer, J. R. (2006). The carbon cycle and associated redox processes through time. Philos. Trans. R. Soc. Lond. B Biol. Sci. 361, 931–950. doi: 10.1098/rstb.2006.1840
Houghton, R. A. (2007). Balancing the global carbon budget. Annu. Rev. Earth Planet. Sci. 35, 313–347. doi: 10.1146/annurev.earth.35.031306.140057
Jones, T. D., Lunt, D. J., Schmidt, D. N., Ridgwell, A., Sluijs, A., Valdes, P. J., et al. (2013). Climate model and proxy data constraints on ocean warming across the Paleocene–Eocene thermal maximum. Earth Sci. Rev. 125, 123–145. doi: 10.1016/j.earscirev.2013.07.004
Kasting, J. F., and Ackerman, T. P. (1986). Climatic consequences of very high carbon dioxide levels in the Earth's early atmosphere. Science 234, 1383–1386. doi: 10.1126/science.11539665
Kelemen, P. B., and Manning, C. E. (2015). Reevaluating carbon fluxes in subduction zones, what goes down, mostly comes up. Proc. Natl. Acad. Sci. U.S.A. 112, E3997–E4006. doi: 10.1073/pnas.1507889112
Kennett, J. P., and Stott, L. D. (1991). Abrupt deep-sea warming, palaeoceanographic changes and benthic extinctions at the end of the Palaeocene. Nature 353:225.
Kramers, J. D. (2002). Global modelling of continent formation and destruction through geological time and implications for CO2 drawdown in the Archaean eon. Geol. Soc. Lond. Spec. Publ. 199, 259–274. doi: 10.1144/GSL.SP.2002.199.01.13
Li, Y., Dasgupta, R., Tsuno, K., Monteleone, B., and Shimizu, N. (2016). Carbon and sulfur budget of the silicate earth explained by accretion of differentiated planetary embryos. Nat. Geosci. 9, 781–785. doi: 10.1038/ngeo2801
Lowe, D. R., and Tice, M. M. (2004). Geologic evidence for Archean atmospheric and climatic evolution: fluctuating levels of CO2, CH4, and O2 with an overriding tectonic control. Geology 32, 493–496. doi: 10.1130/G20342.1
Mackenzie, F. T., and Morse, J. W. (1992). Sedimentary carbonates through phanerozoic time. Geochim. Cosmochim. Acta 56, 3281–3295. doi: 10.1016/0016-7037(92)90305-3
McInerney, F. A., and Wing, S. L. (2011). The Paleocene-Eocene thermal maximum: a perturbation of carbon cycle, climate, and biosphere with implications for the future. Annu. Rev. Earth Planet. Sci. 39, 489–516. doi: 10.1146/annurev-earth-040610-133431
Michael, P. J., and Graham, D. W. (2015). The behavior and concentration of CO2 in the suboceanic mantle: inferences from undegassed ocean ridge and ocean island basalts. Lithos 236, 338–351. doi: 10.1016/j.lithos.2015.08.020
NOAA (2017). Earth System Research Laboratory, Global Monitoring Division. Available online at: www.esrl.noaa.gov/gmd/ccgg/trends/
Rosenthal, A., Hauri, E., and Hirschmann, M. (2015). Experimental determination of C, F, and H partitioning between mantle minerals and carbonated basalt, CO2/Ba and CO2/Nb systematics of partial melting, and the CO2 contents of basaltic source regions. Earth Planet. Sci. Lett. 412, 77–87. doi: 10.1016/j.epsl.2014.11.044
Shaw, G. H. (2008). Earth's atmosphere–Hadean to early Proterozoic. Chem. Erde Geochem. 68, 235–264. doi: 10.1016/j.chemer.2008.05.001
Sleep, N. H., Bird, D. K., and Pope, E. C. (2011). Serpentinite and the dawn of life. Philos. Trans. R. Soc. Lond. B Biol. Sci. 366, 2857–2869. doi: 10.1098/rstb.2011.0129
Sleep, N. H., and Zahnle, K. (2001). Carbon dioxide cycling and implications for climate on ancient earth. J. Geophys. Res. Planets 106, 1373–1399. doi: 10.1029/2000JE001247
Sleep, N. H., Zahnle, K. J., and Lupu, R. E. (2014). Terrestrial aftermath of the moon-forming impact. Philos. Trans. R. Soc. Lond. A Math. Phys. Eng. Sci. 372:20130172. doi: 10.1098/rsta.2013.0172
Storey, M., Duncan, R. A., and Swisher, C. C. (2007). Paleocene-Eocene thermal maximum and the opening of the northeast atlantic. Science 316, 587–589. doi: 10.1126/science.1135274
Urey, H. C. (1952a). On the early chemical history of the earth and the origin of life. Proc. Natl. Acad. Sci. U.S.A. 38, 351–363. doi: 10.1073/pnas.38.4.351
Urey, H. C. (1952b). The Planets: Their Origin and Development. New Haven, CT: Yale University Press.
Urey, H. C. (1956). Regarding the early history of the earth's atmosphere. Geol. Soc. Am. Bull. 67, 1125–1128. doi: 10.1130/0016-7606(1956)67[1125:RTEHOT]2.0.CO;2
Wedepohl, K. H. (1995). The composition of the continental crust. Geochim. Cosmochim. Acta 59, 1217–1232. doi: 10.1016/0016-7037(95)00038-2
Keywords: Urey reaction, carbon, continental crust, atmosphere, early Earth
Citation: Kellogg LH, Turcotte DL and Lokavarapu H (2019) On the Role of the Urey Reaction in Extracting Carbon From the Earth's Atmosphere and Adding It to the Continental Crust. Front. Astron. Space Sci. 6:62. doi: 10.3389/fspas.2019.00062
Received: 30 April 2019; Accepted: 13 September 2019;
Published: 01 November 2019.
Edited by:
Sami Mikhail, University of St Andrews, United KingdomReviewed by:
Fang Huang, Rensselaer Polytechnic Institute, United StatesNikolai Bagdassarov, Goethe University Frankfurt, Germany
Copyright © 2019 Kellogg, Turcotte and Lokavarapu. This is an open-access article distributed under the terms of the Creative Commons Attribution License (CC BY). The use, distribution or reproduction in other forums is permitted, provided the original author(s) and the copyright owner(s) are credited and that the original publication in this journal is cited, in accordance with accepted academic practice. No use, distribution or reproduction is permitted which does not comply with these terms.
*Correspondence: Donald L. Turcotte, ZGx0dXJjb3R0ZUB1Y2RhdmlzLmVkdQ==