- 1SecurAgri, AgroParisTech Innovation, Palaiseau, France
- 2UMR SAD-APT, Université Paris-Saclay, INRAE, AgroParisTech, Palaiseau, France
- 3Institut d'Ecologie et des Sciences de l'Environnement (UMR iEES-Paris), Sorbonne Université, UPEC, UPC, CNRS, INRAE, IRD, Paris, France
- 4UMR SAYFOOD, Université Paris-Saclay, INRAE, AgroParisTech, Palaiseau, France
- 5UMR ECOSYS, Université Paris-Saclay, INRAE, AgroParisTech, Palaiseau, France
- 6Paris City Agronomy Laboratory, Paris Municipal Department Of Green Spaces And Environment (DEVE), Paris, France
Urban agriculture development often faces the problem of soil pollution. Soil engineering consisting in the addition over polluted soils of a top layer made of recycled wastes is a promising solution. This study was co-constructed with urban farmers and aimed at testing in situ the feasibility of growing vegetables safe for consumption in substrates consisting of organic and inorganic waste, directly overlaying soil polluted by trace elements (TE). Two plants were tested: radishes and tomatoes. Three substrates were tested: 1) sheep manure mixed with composted ramial chipped wood (SHW); 2) biowaste compost mixed with mushroom compost and ramial chipped wood (BMW); and 3) deep excavated subsoils mixed with green waste compost (EXC). Only radishes grown in EXC presented levels of TE below the threshold values. For all the other cases, cadmium levels were above the threshold values. This result concerning plant contamination by TE is consistent with a contamination of SHW and BMW substrates by the polluted soil underneath. EXC contained lower TE content, suggesting that mineral materials limited the transfer from the polluted soil towards the substrate overlay. We concluded that adding a combination of mineral and organic waste on top of polluted soils may better mitigate vegetables contamination than adding only organic waste. However, this result was not observed for all tested vegetables. More research is needed to evaluate the best substrate candidate and its adequate thickness, to study its physico-chemical evolution over a longer period of time and to test a larger panel of vegetables.
1 Introduction
Urban agriculture is being developed in many cities of the world, in various forms (e.g. urban farms, community gardens) and at various locations (e.g. on rooftops, on former brownfields) (1–3). Despite its numerous benefits (e.g. decreasing urban heat islands, flood regulation, fostering the social link, access to fresh food) (4, 5), urban agriculture faces a challenging issue: the risk of exposure to natural and anthropogenic pollution (6, 7). Urban soils are known to be potentially contaminated, particularly by trace elements (TE); lead (Pb), cadmium (Cd), mercury (Hg), zinc (Zn) and copper (Cu) are often present at abnormally high levels (8–11). These TE in polluted soils can contaminate vegetables (12–15). Urban cultivators, visitors and consumers may then be exposed to TE hazards by ingestion of vegetables or by ingestion or inhalation of soil particles (16–18).
Phyto-technologies offer well documented and environmentally friendly solutions for the alleviation of TE soil contamination. However, given the low annual remediation factor (19), the time required to decontaminate soil by plants is often estimated to be tens to hundreds of years. As a rapid solution to this major threat for urban agriculture, polluted soils are often covered by a landscape fabric or geotextile membrane and a layer of clean topsoil. Plant contamination and human exposure are thus limited. Yet, the importation into cities of clean topsoil from surrounding areas is expensive and increases the ecological footprint of cities (20).
An alternative to the use of clean topsoil extracted from rural environment can be found in recycling certain organic and mineral wastes to produce fertile Technosols. Some of these Technosols have been shown to allow the production of grassland plants (21, 22), trees (23, 24) and vegetables (25, 26). They have also been used successfully to reduce Pb exposure in urban community gardens (27).
In Nanterre (Ile-de-France region, France), urban farmers are developing a practice on a specific site to cultivate polluted soil using an overlay of fertile substrate. Their substrate is made from local organic wastes and their objective is to produce vegetables, with the lowest environmental and financial impact possible. Such local and low-cost practices aiming at restoring degraded urban soils to produce vegetables are often used by urban farmers (28).
The aim of this study is to build scientific knowledge with these urban farmers about such practice and to analyze its relevance and limits regarding the food production objective. Such innovative approach involving the bridging between academic institutions and non-academic stakeholders is emerging to foster knowledge co-production for a sustainable environment (29).
We tested in situ the feasibility of growing vegetables in three different substrates directly overlaying the polluted soil: the one created by the local farmers and two others presenting contrasted characteristics. In the context of highly polluted soil at the time of harvesting, we compared for the different substrates both the transfer of TE from soil to initially clean overlaying substrate and to the edible parts of grown vegetables.
2 Material and method
2.1 Experimental design
The experimentation was carried out in an urban farm located in a deeply artificialized zone in Nanterre (48°54’01.0”N 2°12’33.2”E, France) (Figure 1A). In the past, the area was repeatedly covered by undefined wastes now reaching a thickness of several meters. The site has been cultivated since 2008. In April 2018, the soil of the experimental zone was cleared, unpacked, sampled and analyzed. In comparison to the regional threshold values defined by Mathieu et al. (30), the TE contents of this soil largely exceeded the thresholds, except for chromium (Table 1). For instance, Pb content was ten times, Cu 20 times and Cd 33 times higher than the respective threshold value. The experimental zone was a rectangle of about 32 m2 comprising three randomized complete blocks. Each block contained three treatments separated by a distance of 50 cm. For each treatment, the layer of substrate covering in the soil was 30 cm thick, with a surface area of 3 m2. Mulch made of straw was added on the inter rows, to hold soil moisture and to avoid contamination by airborne dust (Figure 1B).
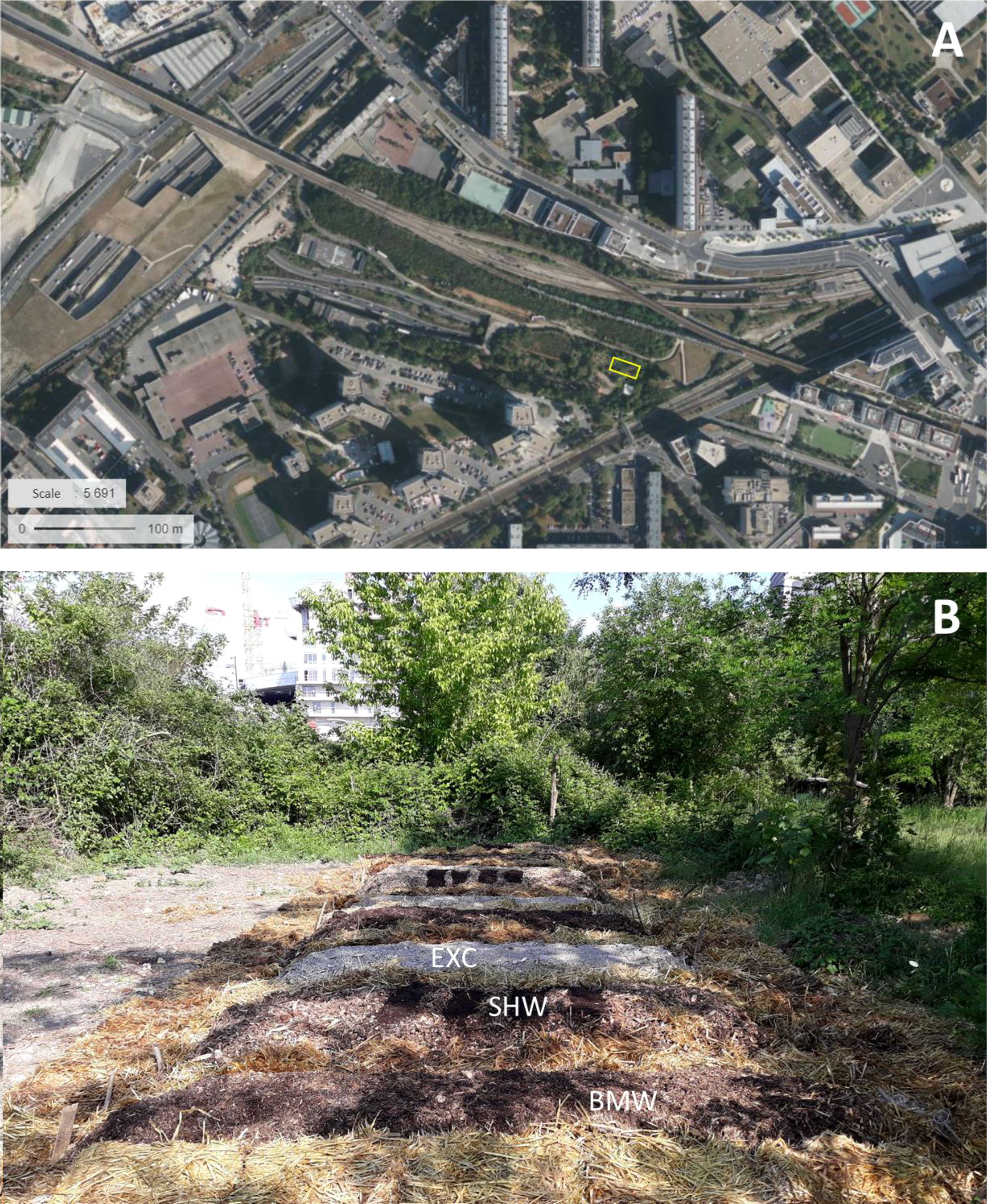
Figure 1 Localization of the site (A) © IGN, 2021 and picture of the experimental zone (B) © A. Barbillon, 2019.
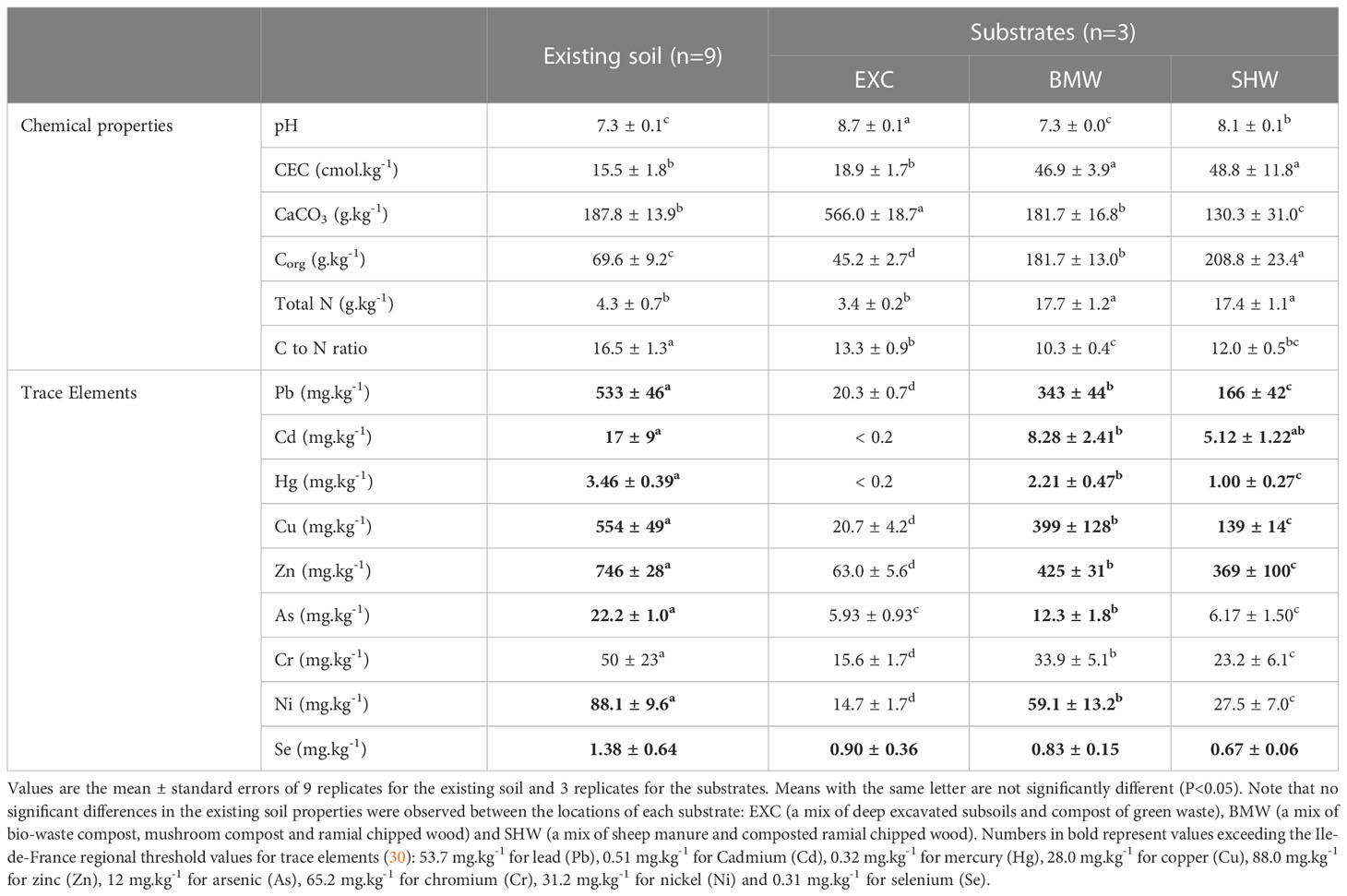
Table 1 Major chemical properties and trace element content (mg.kg-1 of dry weight) of the existing soil and the 3 different substrates added on top of it.
2.2 Substrate composition
Three different mixtures, called substrates, were used to cover the polluted soil. SHW was made (50%-50%, v/v) of sheep manure and composted Ramial Chipped Wood (RCW). Sheep manure came from the local urban farm and was kept on a pile for one year. RCW came from a pruning firm.
BMW was a mix (15%-68%-17%, v/v) of bio-waste compost, mushroom compost, and RCW. Biowaste compost and mushroom compost were produced by a circular economy firm that grows mushrooms. RCW also came from a pruning firm.
EXC was made (50%-50%, v/v) of deep excavated subsoils and compost of green waste (26). Excavated soil and compost came from a specialized firm in the region.
All components of the three mixtures were analyzed prior to the experiment and had TE levels under the threshold values of NFU 44-051 standard for compost and organic amendments (Table S1).
2.3 Physico-chemical properties
In April 2018, the existing soil was sampled at the exact place where the substrates would be placed afterwards. Each sample was made of 15 subsamples collected using a manual auger on the first 30 cm.
In October 2019, each substrate overlay was sampled. Each sample was made of eight to ten subsamples collected with a manual auger from the first 20 cm of the substrate, so that we had one composite sample for each replicate of the experiment.
The soil samples analyzed were air-dried, weighed and sieved to 2 mm. Aliquots were ground to<250μm particles using an ultra-centrifugal mill (Retsch type MM400), only for C, N and TE analyses.
The particle size distribution of the fine earth (< 2 mm) was determined by sieving and sedimentation (NF ISO 11277). The pH was measured in water (NF ISO 10390). The Cation Exchange Capacity (CEC) was determined after percolation of 1.0M ammonium acetate solution at pH 7 (NF X 31-130). CaCO3 was determined by the volumetric method (NF ISO 10693). Corg and Total N analysis were carried out by dry combustion (ISO 10694).
Regarding the physical properties, BMW and SHW had a similar particle size distribution, with most particles between 0.05 and 2 mm, whereas EXC had more particles that were smaller than 0.05 mm. The existing soil had an intermediate particle size distribution (Figure S1). Regarding chemical properties, the existing soil and BMW had a neutral pH. EXC and SHW had a more basic pH. The CEC of the existing soil and of EXC were more than two times lower than for BMW and SHW. The CaCO3 of EXC was more than 3 times higher than for the existing soil, BMW and SHW. EXC had the lowest level of organic carbon, 1.5 times lower than for the existing soil. BMW and SHW had 2.5 to 4.5 times more organic carbon than the existing soil and EXC. BMW and SHW contained also 4 times more nitrogen than the existing soil and EXC. C to N ratios of EXC, BMW and SHW were similar to those of agricultural soils (31), and the C to N ratio of the existing soil was higher (Table 1).
2.4 Trace element analyses in soil and substrates
Nine TE were analyzed: lead (Pb), cadmium (Cd), mercury (Hg), copper (Cu), zinc (Zn), arsenic (As), chromium (Cr), nickel (Ni) and selenium (Se). Hg was analyzed directly by thermal decomposition, amalgamation and atomic absorption with Milestone’s DMA-80. Others TE were extracted according to the NF ISO 11466 standard for material with a low organic content and according to the NF EN 13346 standard for organic substrates. Single use vessels were used when possible: PFTE tubes for microwave digestions (Anton Paar), and 50 ml PP tubes with HDPE cap, free of heavy metal (Greiner bio-one). Other vessels were cleaned by using high purity water and HNO3 and HCl for TE analyses (Chem-Lab). TE were analyzed by high-frequency induced plasma optical emission spectrometry according to the NF ISO 22036 standard, by using a Thermo ICAP 7000 equipment.
For setting the instruments and quality control of TE analyses of soil extracts, the multi-element calibration range was prepared from certified single-element solutions (Chem-Lab). Control solutions were prepared from two certified multi-element solutions (Chem-Lab and Agilent) and was inserted every ten samples. Control soil material was included from the extraction step in every sample set: two internal reference soil samples for most TE analyses, and one certified reference sediment sample (CRM016, Sigma-Aldrich) for Hg. Periodic control reports checked and validated data sets. Quantification limits expressed in mg.kg-1 soil were 4, 0.2, 5, 10, 0.2, 5, 10, 0.5 and 25 for As, Cd, Cr, Cu, Hg, Ni, Pb, Se and Zn, respectively.
2.5 Plant growth conditions and trace element analyses
This article focuses on a “root” vegetable and a “fruit” vegetable cultivated in 2019. Radish seeds (“Cherry Belle bio” variety) and tomato seedlings (“Coeur de Boeuf” variety) were both bought from an organic seed company. Two tomato seedlings per substrate were planted on 28th June 2019. Tomatoes grew well in all three substrates. Radishes were sown on 3rd September 2019 and again in the BMW substrate on 20th September because the first seeds had not germinated. The radishes grew well in SHW and EXC but struggled in BMW and remained small. On 25th September and 9th October, a sample of one or two tomatoes for each substrate was harvested. All the radishes were harvested on 30th October. There were three radishes per sample, for each substrate. After washing the vegetables with ordinary tap water, the fresh and dry matter was weighed to estimate their water content.
They were mineralized using a digestion block (DigiPREP SCP SCIENCE) and aqua regia according to NF X 31,415 using ultrapure HCl and HNO3 (PlasmaPure, SCP Science). Disposable vessels verified for leachable metals were used (50 mL PP DigiTubes 50mL). Five TE – Cd, Pb, Cu, Zn and Hg were analyzed by Atomic Absorption Spectrophotometry (AAS), using High-Resolution Continuum Source instrumentation (contrAA 800D Analytik Jena) and a polarized Zeeman AAS model (Z5000 Hitachi).
Furnace atomization was used for Pb, Cu and Cd absorbance measurements. For calibrating the instruments, standard solutions were prepared from 1000 mg/L solutions (SCP Science) at 0, 10, 20 ppb for Pb and Cu, and 0, 2, 4 ppb for Cd. HNO3 5% was used to dilute the standards, as well as for samples when needed. The modifiers NH4H2PO4 1% and (Pd(NO3)2 0.2% + Mg(NO3)2 0.1%) were used for analyzing Pb and Cd, respectively. No modifier was required for Cu. All reagents were certified for SAA and ICP analyses.
Flame AAS and cold vapor AAS were used to determine Zn and Hg, respectively. Standard solutions 0, 0.5, 1, 2 mg/L in HNO3 5% were used for the calibration curve of Zn. For Hg, 10 ml of solution at 0, 0.5, 1 µg/L was used, because of the specific method applied: 10 ml of sample or standard was introduced in a polypropylene tube, 0.5 ml of SnCl2 (25% in HCl 20%) was added to reduce Hg, which was carried away under argon degassing flow and amalgamated on a golden trap. The trap was then heated at 500°C and the Hg vapor produced was measured by AAS.
All measurements were duplicated. To verify the absence of matrix effects, serial dilutions were made on one sample to see if the AAS measurements of all the subsamples matched their dilutions. All methods for plant analyses were validated using a certified reference material: Poplar Leaves NCS DC73350 (SCP Science).
2.6 Bioconcentration factor calculation for radishes
The bioconcentration factor (BCF) was calculated as the ratio of contents in the vegetable and in the growing substrate (32, 33).
TE content in radishes was expressed in mg.kg-1 of fresh matter (Cveg) and TE content in substrates in mg.kg-1 of dry weight (Cs).
2.7 Statistical analysis
All statistical analyses were carried out using R software version 3.3.1 (R Development Core Team). After normality and homoscedasticity verifications (Shapiro and Bartlett test), the different parameters were analyzed using an ANOVA to compare the different modalities of substrates. When significant (P< 0.05) effects were found, comparisons among means were performed using the Least Significant Difference (LSD) test (“agricolae” package). A radar diagram was drawn up using SigmaPlot 14.0 (Systat Software Inc.).
3 Results
3.1 Trace elements in vegetables grown in different substrates
Radishes grown in EXC and SHW had the same weight on average (42 ± 6 g and 42 ± 7 g) when collected. When grown in BMW they weighed significantly (P<0.001) less with only 5 ± 2 g. Concentrations of TE in radishes varied significantly (P<0.05) with the substrates (Table 2). Radishes grown in EXC showed the lowest TE content and those grown in BMW showed the highest levels of TE. Pb and Cu content in radishes grown in EXC was at least half that of radishes grown in SHW, and four times lower than that of radishes grown in BMW. Cd content was four times lower than in radishes grown in SHW and eight times lower than in those grown in BMW. For Hg, radishes grown in each substrate presented similar low content levels.
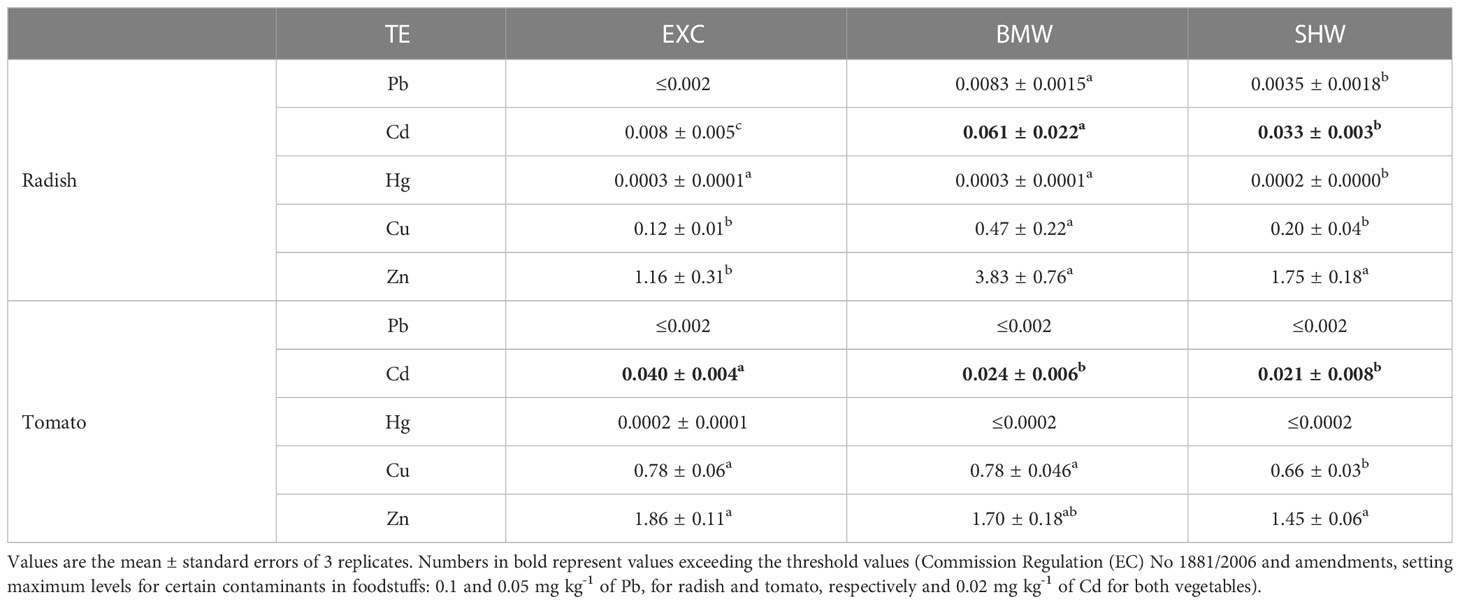
Table 2 Total trace element (TE) contents (mg.kg-1 of fresh weight) in the vegetables grown in the 3 different substrates: EXC (a mix of deep excavated subsoils and compost of green waste), BMW (a mix of bio-waste compost, mushroom compost and ramial chipped wood) and SHW (a mix of sheep manure and composted ramial chipped wood).
Regulatory threshold values exist for Pb and Cd (Commission Regulation (EC) No 1881/2006 and amendments, setting maximum levels for certain contaminants in foodstuffs). For Pb, radishes grown in all substrates remained under these threshold values. For Cd, radishes grown in SHW and BMW were 1.5 to 3 times above the threshold value.
The bioconcentration factor (BCF) for Pb and Cd was calculated for radishes. The BCF-Pb level for EXC was ≤ 9.9.10-5 (Pb content in radishes grown in EXC was ≤ 0.002 mg.kg-1), BCF-Pb for BMW was 2.4.10-5 and BCF-Pb for SHW was 2.1.10-5. The BCF-Cd level for EXC was ≥ 4.0.10-2 (Cd content in EXC was ≤ 0.2 mg.kg-1), BCF-Cd for BMW was 7.4.10-3 and BCF-Pb for SHW was 6.4.10-3.
Tomatoes grew similarly in all substrates with 327 ± 82 g, 346 ± 25 g and 378 ± 98 g, respectively in EXC, SHW and BMW. With the exception of Pb and Hg, significant (P<0.05) differences in TE content in tomatoes were found between at least two of the three substrates (Table 2). Pb content in tomatoes was always below quantification limits. Tomatoes grown in EXC showed a Cd content two times higher than tomatoes grown in the other two substrates, and two times higher than the regulatory threshold value of 0.02 mg.kg-1 fresh matter. Cd content in tomatoes grown in BMW and SHW were just above their threshold value.
3.2 Trace elements in soil and substrates
The substrates used to cover polluted soil were variously contaminated but still had lower TE content than the existing soil (Table 1). Statistical analyses showed that all TE concentrations in the soil varied significantly (P<0.001) among the different substrates, with the exception of Se. EXC was not contaminated by TE, except for Se. BMW had the highest TE contamination and showed TE levels 2 to 40 times higher than in EXC, except for Se. But BMW still had 1.5 to 2 times lower levels than the existing soil, for all TE. SHW had a contamination level midway between BMW and EXC (Table 1).
For BMW, Pb content was six times higher than the threshold value, Hg seven times higher, Cd 16 times higher, and Cu 14 times higher. SHW was less contaminated than BMW but its Pb and Hg levels were still three times higher than the threshold values. Cd levels were ten time higher and Cu five times higher than the threshold values. TE content in EXC was below threshold values in all cases except for Se (Table 1).
TE content in substrates can be compared to the soil initial concentrations (Figure 2). The mitigation potential is much higher for EXC than the two others substrates. BMW decreased by a maximum of 50% the TE content compared to the soil underneath, with even no significant difference for Cu. The TE mitigation is higher for SHW or equivalent to that of BMW for Cr, Cd and Zn. For EXC, TE content is about 10% of that found in the underlying soil and the mitigation is significantly higher than the two other substrates, except for As, which is the same level as for SHW.
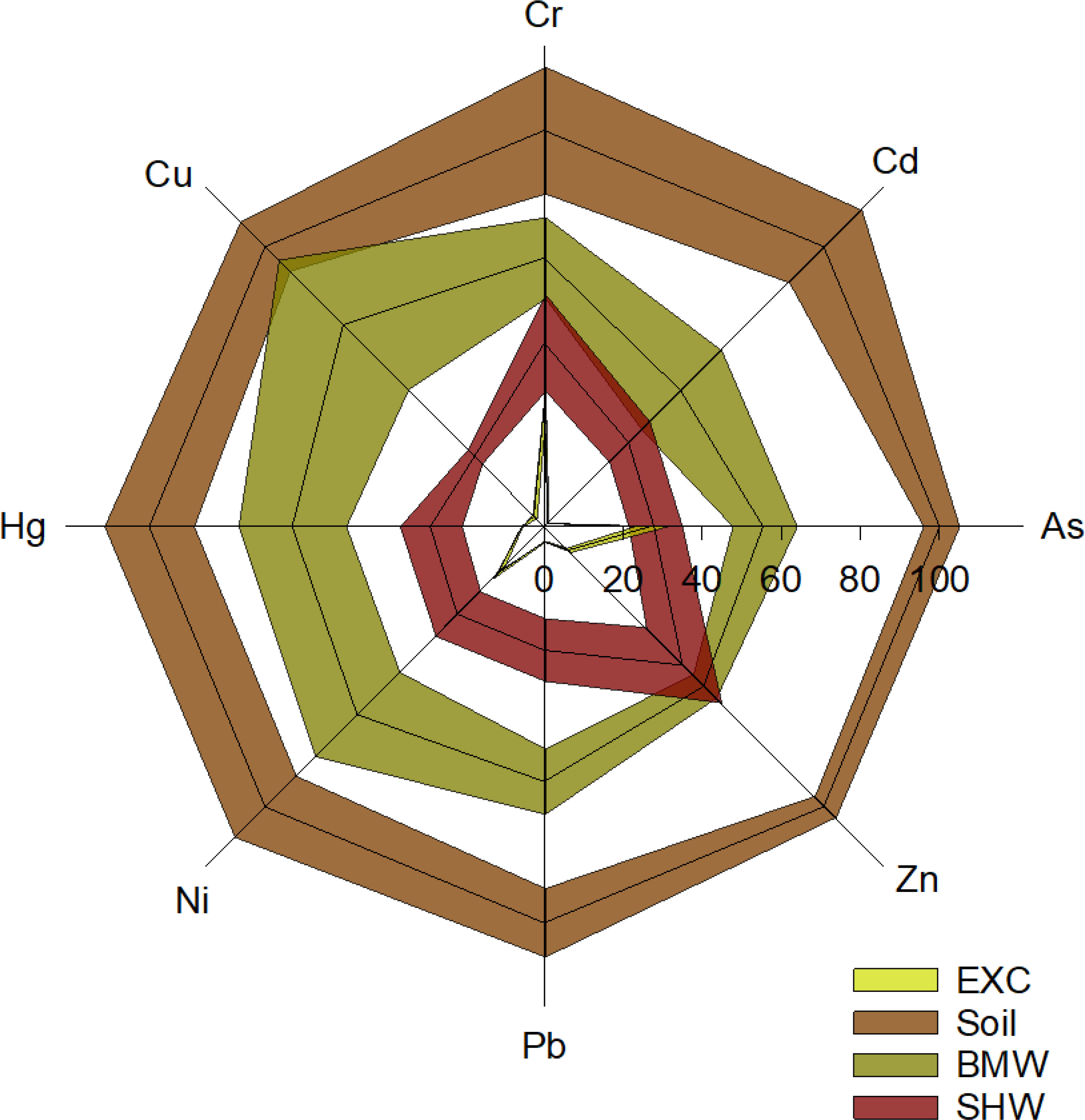
Figure 2 Percentage of decrease in trace elements (Pb: lead, Ni: nickel, Hg: mercury, Cu: copper, Cr: chromium, Cd: cadmium, As: arsenic, Zn: zinc) content in the 3 different substrates in comparison to the soil underneath. Values are the mean ± standard errors (n=9 for the soil and n=3 for the substrates). .
4 Discussion
The similarity of TE patterns (Figure 2) between BMW, SHW and the existing soil suggests that the samples of BMW and SHW substrates might have been mixed with the polluted soil underneath, because of the partial sinking of the substrate layer that was observed over time. That was not the case for EXC, since the thickness of the substrate layer did not change during the experiment, which limited the risks of it mixing with the polluted soil underneath. The decrease of the thickness of substrate layer over the polluted soil may also explain the higher TE contamination of radishes grown in BMW and SHW than in EXC.
Relatively high Se levels found for the EXC substrate is consistent with studies carried out by the French Geological Survey (BRGM) (34, 35), showing that Se concentrations in ground water and surface water of the Parisian basin are mostly influenced by natural concentration in sedimentary layers from the Paleocene or Eocene. These often correspond to sulfur minerals such as pyrite or marcassite with sulfur substitutions by selenium (36).
Pb content in vegetables is above quantification limits only for radish, grown in BMW and SHW (Table 2). This confirms that “root” vegetables accumulate more Pb than do “fruit” vegetables (16, 37–39), which can be due to the low solubility of Pb overall and biochemical barriers in plant cells from root to fruit. Pb contamination of aerial organs may however also occur through soil particle dispersal and redeposition over short distances, and may remain even when the vegetable is washed (38). In our field experiment, the Pb content of radish, quantified only for two of the three substrates, remained under regulatory thresholds.
Considering cadmium, it appears that its content in radishes grown in BMW and SHW is 2 or 3 times higher than the threshold values. Such vegetables are not usable for consumption. On the contrary, radishes grown in EXC have Cd content lower than threshold values and are acceptable for consomption. Bioconcentration factors calculated for radishes harvested on each substrate are at least 100 times higher for Cd than for Pb – a ratio close to the one presented by Pelfrene et al. (16) and confirming the known bioavailability of this TE (40, 41).
When comparing TE levels in radishes and in the three substrates, the same order is observed, for Pb, Cd, Cu and Zn (Tables 1, 2). Radishes grown in BMW, which is the most contaminated substrate, are the most contaminated, followed by radishes grown in SHW, and lastly those grown in EXC. Soil factors other than the total TE content can influence TE uptake by radishes. With respect to pH, they are all above 7 (Table 1) and it is known that the retention rate of Cd, in particular, by common soils is very high and varies little above this pH (42). Considering other retention factors, Table 1 shows CEC varying between 19 cmol.kg-1 for EXC and 49 for SHW, i.e, by a factor of 2.5, whereas Cd or Pb levels increase by 1 order of magnitude between EXC and the two organic substrates. Variations of organic carbon and CaCO3 between substrates appear a little wider than for the CEC, although by less than one order of magnitude. The organic components of the substrates may also have an influence. Finally, this study shows that TE content of radishes are mainly determined by TE content within the soil in which they grow and that a substrate with persistent thickness overlaying the polluted soil may mitigate the contamination.
The TE content of tomatoes does not follow the same order in the three substrates (Tables 1, 2). Only Cd content in tomatoes needs to be discussed, since no significant differences were otherwise recorded. None of the substrates are safe for growing tomatoes since Cd levels are all higher (EXC) or slightly higher (BMW, SHW) than the threshold value. The high bioavailability of Cd has already been mentioned, and even if “fruit” vegetables generally contain less Cd than “leafy” and “root” vegetables, this TE can be transported from roots to stems and leaves, and to the seeds and organs of fruit (10, citing 43, 41).
The Cd content of tomatoes grown in EXC is moreover twice as high as that in tomatoes grown in BMW and SHW, even though EXC is the least contaminated substrate of all. SHW and BMW substrates contain 4 time more nitrogen (respectively 17.4 and 17.7 g.kg-1) than EXC (3.4 g.kg-1), so the root exploration of tomatoes may have stayed more superficial for those grown in SHW and BMW than in EXC. The higher Cd content of tomatoes grown in the EXC substrate may correspond to deeper root development toward the polluted existing soil. Machado and Oliveira (44) demonstrated that most of the tomato plant’s root system was concentrated in the top 40 cm of the soil profile in their experimental conditions, while in our study the initial thickness of all the substrates was 30 cm.
Numerous studies aimed to reduce soil-plant transfer of metal contaminants by various amendments, as reviewed by Hamid et al. (45). Very few attempted to consider current gardener practices using local residue resources. Egendorf et al. (27) used a mixture of mineral and organic materials looking like EXC of our study. However, they applied a layer separated from the polluted soil by a landscape fabric. In other field experiments, a large amount of amendment was incorporated to the polluted soil itself (46–48), which did not always induce a reduction of TE transfer toward vegetables. These studies focused on Pb, or Pb and As, and not on Cd. The presented results enlightened, beyond the nature of added substrates, the plausible role of their placement and fertilizing potential.
5 Conclusion
In this experiment we tested – for the first time in situ – if the use of organic and mineral waste substrates directly in contact with a polluted urban soil could allow to grow vegetables safe for consumption. For the substrate consisting in a mixture of mineral and organic wastes, our experiment showed that grown radishes were safe for human consumption. This organo-mineral mixture appears to be a better candidate to create a relatively safe substrate to grow edible crops on top of contaminated urban soils than organic mixtures. However, this advantage shown for shallow rooted legumes such as radishes, is not confirmed for the edible part of deeper rooted vegetables. In our experiment, it appears that none of tomatoes grown in the three substrates are safe for human consumption, even if “fruit” vegetable are known to be less contaminated by TE than “root” vegetables. It hence appears that the thickness of the substrate overlaid over polluted soils and its durability is of main importance for a safe cultivation of vegetables. Our results also show that the thickness of the substrate covering the polluted soils may be adapted with respect to the depth of the root system of the cultivated vegetable.
The use of local resources to build a clean topsoil over a polluted soil could be a low-cost and environmentally friendly way to manage the challenging issue of polluted soils that urban agriculture faces in various situations across the world.
Nevertheless, we demonstrated that there was a risk of contamination of the topsoil by mixing with the polluted existing soil, or by the vertical mobility of pollutants and the subsequent TE transfer to vegetables through deep root development.
These first results obtained in this study are an encouragement to conduct further research, especially on substrates containing a mixture of organic and mineral waste, which seems to lower TE contamination of vegetables. Future research should focus on substrate evolution after long periods of time and TE vertical mobility, for example by testing different thicknesses and compositions of substrates, and observing various vegetables’ root development in various existing soil contamination situations. More research could also be done to test different sustainable physical barriers, other than textile membranes, that could be placed between polluted soil and clean topsoil to limit pollutant transfers.
Data availability statement
The raw data supporting the conclusions of this article will be made available by the authors, without undue reservation.
Author contributions
AB wrote the manuscript with significant contributions from TL and active participation of PC and HR; AB, CA and NM designed the research; AP-B, JA and HR set up the experiments. AB carried out the sampling. FN and SB carried out the analyses. AB, TL and PC analyzed the data. All the authors contributed to the article and approved the submitted version.
Funding
The authors thank the French Agency for Ecological Transition (ADEME) and the Ile-de-France Region for their financial support under the research program REFUGE: Risques en Fermes Urbaines - Gestion et Evaluation (ADEME: n°18IFC0080, Ile-de-France region: n°17009063).
Acknowledgments
The authors thank Mathieu Ohlmann (La Ferme du Bonheur), Maximilien Koegler (Upcycle) and Roberto Garcia (AgroParisTech) for their technical assistance. Bastien Lambert, Chenchen Fan, Aurélie Sécheret, and Florie Gay, who contributed to this study during their training period, are also acknowledged.
Conflict of interest
The authors declare that the research was conducted in the absence of any commercial or financial relationships that could be construed as a potential conflict of interest.
Publisher’s note
All claims expressed in this article are solely those of the authors and do not necessarily represent those of their affiliated organizations, or those of the publisher, the editors and the reviewers. Any product that may be evaluated in this article, or claim that may be made by its manufacturer, is not guaranteed or endorsed by the publisher.
Supplementary material
The Supplementary Material for this article can be found online at: https://www.frontiersin.org/articles/10.3389/fsoil.2023.1163356/full#supplementary-material
References
1. Goldstein B, Hauschild M, Fernández J, Birkved M. Urban versus conventional agriculture, taxonomy of resource profiles: a review. Agron Sustain Dev (2016) 36(1):9. doi: 10.1007/s13593-015-0348-4
2. Sanye-Mengual E, Specht K, Vavra J, Artman M, Orsini F, Gianquinto G. Ecosysems services of urban agriculture: perceptions of project leaders, stakeholders and the general public. Sustainability (2020) 12:10446. doi: 10.3390/su122410446
3. Aubry C, Giacchè G, Maxime F, Soulard C. Les Agricultures urbaines en France: comprendre les dynamiques, accompagner les acteurs. Quae « savoir faire » (2022) 224 p.
4. Dorr E, Goldstein B, Horvath A, Aubry C, Gabrielle B. Environmental impacts and resource use of urban agriculture: a systematic review and meta-analysis. Environ Res Lett (2021) 16(9):093002. doi: 10.1088/1748-9326/ac1a39
5. Payen FT, Evans DL, Falagán N, Hardman CA, Kourmpetli S, Liu L, et al. How much food can we grow in urban areas? food production and crop yields of urban agriculture: a meta-analysis. Earth’s Future (2022) 10(8). doi: 10.1029/2022EF002748
6. Xiong T, Dumat C, Dappe H, Vezin E, Schreck M, Sahid M, et al. Copper oxide nanoparticle foliar uptake, phytotoxicity, and consequences for sustainable urban agriculture. Environ Sci Technol (2017) 51(9):5242–51. doi: 10.1021/acs.est.6b05546
7. Dumat C, Pierart A, Shahid M, Khalid S. Pollutants in urban agriculture: sources, health risk assessment and sustainable management. In: Bioremediation of Agricultural Soils. Sanchez-Hernandez JC (ed). CRC press Taylor & Francis Group (2019) 61-84. doi: 10.1201/9781315205137
8. Foti L, Dubs F, Gignoux J, Lata JC, Lerch TZ, Mathieu J, et al. Trace element concentrations along a gradient of urban pressure in forest and lawn soils of the Paris region (France). Sci Total Environ (2017) 598:938–48. doi: 10.1016/j.scitotenv.2017.04.111
9. Gitton C, Verger Y, Brondeau F, Charvet R, Nold F, Branchu P, et al. L’économie circulaire: cercle vertueux ou cercle vicieux? le cas de l’utilisation de terres maraîchères pour aménager des espaces verts urbains. Géocarrefour - Rev géographie Lyon (2018) 92(2). doi: 10.4000/geocarrefour.11950
10. Bidar G, Pelfrêne A, Schwartz C, Waterlot C, Sahmer K, Marot F, et al. Urban kitchen gardens: effect of the soil contamination and parameters on the trace element accumulation in vegetables – a review. Sci Total Environ (2020) 738:139569. doi: 10.1016/j.scitotenv.2020.139569
11. Zhong X, Joimel S, Schwartz C, Sterckeman T. Assessing the future trends of soil trace metal contents in French urban gardens. Environ Sci pollut Res (2022) 29:3900–17. doi: 10.1007/s11356-021-15679-4
12. Samsøe-Petersen L, Larsen EH, Larsen PB, Bruun P. Uptake of trace elements and PAHs by fruit and vegetables from contaminated soils, environ. Sci Technol (2002) 36(14):3057–63. doi: 10.1021/es015691t
13. Gupta N, Yadav KK, Kumar V, Kumar S, Chadd RP, Kumar A. Trace elements in soil-vegetables interface: translocation, bioaccumulation, toxicity and amelioration-a review. Sci Total Environ (2019) 651:2927–42. doi: 10.1016/j.scitotenv.2018.10.047
14. Paltseva AA, Cheng ZQ, Egendorf SP, Groffman PM. Remediation of an urban garden with elevated levels of soil contamination. Sci Total Environ (2020) 722:137965. doi: 10.1016/j.scitotenv.2020.137965
15. Taylor MP, Isley CF, Fry KL, Liu X, Gillings MM, Rouillon M, et al. A citizen science approach to identifying trace metal contamination risks in urban gardens. Environ Int (2021) 155:106582. doi: 10.1016/j.envint.2021.106582
16. Pelfrêne A, Douay F, Richard A, Roussel H, Girondelot B. Assessment of potential health risk for inhabitants living near a former lead smelter. part 2: site-specific human health risk assessment of cd and Pb contamination in kitchen gardens. Environ Monit (2013) 185:2999–3012. doi: 10.1007/s10661-012-2767-x
17. Barbillon A, Aubry C, Nold F, Besancon S, Manouchehri N. Health risks assessment in three urban farms of Paris region for different scenarios of urban agricultural users: a case of soil trace metals contamination. Agric Sci (2019) 10:352–70. doi: 10.4236/as.2019.103029
18. Paltseva AA, Cheng Z, McBride M, Deeb M, Egendorf SP, Groffman PM. Legacy lead in urban garden soils: communicating risk and limiting exposure. Front Ecol Evol (2022) 10:873542. doi: 10.3389/fevo.2022.873542
19. Mench M, Schwitzguébel JP, Schroeder P, Schroeder P, Gawronski S, Gupta S. Assessment of successful experiments and limitations of phyto-technologies: contaminant uptake, detoxification and sequestration, and consequences for food safety. Environ Sci Pollut Res (2009) 16:876–900. doi: 10.1007/s11356-009-0252-z
20. Walsh D, Glass K, Morris S, Zhang H, McRae I, Anderson N, et al. Sediment exchange to mitigate pollutant exposure in urban soil. J Environ Manage (2018) 214:354–61. doi: 10.1016/j.jenvman.2018.03.013
21. Deeb M, Desjardins T, Podwojewski P, Pando A, Blouin M, Lerch TZ. Interactive effects of compost, plants and earthworms on the aggregations of constructed technosols. Geoderma (2017) 305:305–13. doi: 10.1016/j.geoderma.2017.06.014
22. Rees F, Dagois R, Derrien D, Fiorelli J-L, Watteau F, Morel JL, et al. Storage of carbon in constructed technosols: in situ monitoring over a decade. Geoderma (2019) 337:641–8. doi: 10.1016/j.geoderma.2018.10.009
23. Cannavo P, Guénon R, Galopin G, Vidal-Beaudet L. Technosols made with various urban wastes showed contrasted performance for tree development during a 3-year experiment. Environ Earth Sci (2018) 77(18):650. doi: 10.1007/s12665-018-7848-x
24. Pruvost C, Mathieu J, Nunan N, Gigon A, Pando A, Lerch TZ, et al. Tree growth and macrofauna colonization in technosols constructed from recycled urban wastes. Ecol Eng. (2020) 153:105886. doi: 10.1016/j.ecoleng.2020.105886
25. Grard B, Manouchehri N, Aubry C, Frascaria-Lacoste N, Chenu C. Potential of technosols created with urban by-products for rooftop edible production. Int J Environ Res Public Health (2020) 17:3210. doi: 10.3390/ijerph17093210
26. Araujo JHR, Pando-Bahuon A, Hartmann C, Aroui-Boukbida H, Desjardins T, Lerch TZ. Making green(s) with black and white: constructing soils for urban agriculture using earthworms, organic and mineral wastes. Front Ecol Evol (2022) 10:884134. doi: 10.3389/fevo.2022.884134
27. Egendorf SP, Cheng Z, Deed M, Flores V, Paltseva A, Walsh D, et al. Constructed soils for mitigating lead (Pb) exposure and promoting urban community gardening: the new York city clean soil bank pilot study. Landsc Urban Plan (2018) 175:184–94. doi: 10.1016/j.landurbplan.2018.03.012
28. Mougin C, Douay F, Canavese M, Lebeau T, Rémy E. Les Sols urbains sont-ils cultivables? Quae « matière à débattre décider » (2020) 228 p.
29. Irwin EG, Culligan PJ, Fischer-Kowalski M, Law KL, Murtugudde R, Pfirman S. Bridging barriers to advance global sustainability. Nat Sustain (2018) 1:324–32. doi: 10.1038/s41893-018-0085-1
30. Mathieu A, Baize D, Raoul C, Daniau C. Proposition de référentiels régionaux en éléments traces métalliques dans les sols: leur utilisation dans les évaluations des risques sanitaires. Environ Risques St. (2008) 7:112–22. doi: 10.1684/ers.2008.0142
31. Persson J, Kirchmann H. Carbon and nitrogen in arable soils as affected by supply of n fertilizers and organic manures. Agric Ecosyst Environ (1994) 51:249–55. doi: 10.1016/0167-8809(94)90048-5
32. Alloway BJ, Jackson AP, Morgan H. The accumulation of cadmium by vegetables grown on soils contaminated from a variety of sources. Sci Total Environ (1990) 91:223–36. doi: 10.1016/0048-9697(90)90300-J
33. Douay F, Pelfrêne A, Planque J, Fourrier H, Richard A, Roussel H, et al. Assessment of potential health risk for inhabitants living near a former lead smelter. part 1: metal concentrations in soils, agricultural crops, and homegrown vegetables. Environ Monit Assess (2013) 185:3665–80. doi: 10.1007/s10661-012-2818-3
34. Chabart M, Gourcy L, Braibant G, Ghestem JP, Perceval W. Origine des anomalies en sélénium dans les captages d’AEP du département de la marne. Première Approche (2006).
35. Brenot A, Blum A, Chery L, Pons A, Mascre C, Allier D, et al. Identification des zones à risques de fond géochimique élevé en éléments traces dans les cours d’eau et les eaux souterraines. Bassin Seine-Normandie (2007).
36. Manceau A, Merkulova M, Mathon O, Glatzel P, Murdzek M, Batanova V, et al. The mode of incorporation of as(-I) and se(-I) in natural pyrite revisited. ACS Earth Space Chem (2020) 4(3):379–90. doi: 10.1021/acsearthspacechem.9b00301
37. Pruvot C, Douay F, Hervé F, Waterlot C. Heavy metals in soil, crops and grass as a source of human exposure in the former mining areas. J Soils Sediments (2006) 6:215–20. doi: 10.1065/jss2006.10.186
38. McBride MB, Shayler HA, Spliethoff HM, Mitchell RG, Marquez-Bravo LG, Ferenz GS, et al. Concentrations of lead, cadmium and barium in urban garden grown vegetables: the impact of soil variables. Environ Pollut (2014) 194:254–61. doi: 10.1016/j.envpol.2014.07.036
39. Augustsson ALM, Uddh-Söderberg TE, Hogmalm KJ, Filipsson MEM. Metal uptake by homegrown vegetables - the relative importance in human health risk assessments at contaminated sites. Environ Res (2015) 138:181–90. doi: 10.1016/j.envres.2015.01.020
41. Sterckeman T, Thomine S. Mechanisms of cadmium accumulation in plants. Crit Rev Plant Sci (2020) 39:322–59. doi: 10.1080/07352689.2020.1792179
42. Degryse F, Smolders E, Parker DR. Partitioning of metals (Cd, Co, Cu, Ni, Pb, zn) in soils: concepts, methodologies, prediction and applications – a review. Eur J Soil Sci (2009) 60:590–612. doi: 10.1111/j.1365-2389.2009.01142.x
43. Rodriguez-Iruretagoiena A, Trebolazabala J, Martinez-Arkarazo I, De Diego A, Madariaga JM. Metals and metalloids in fruits of tomatoes (Solanum lycopersicum) and their cultivation soils in the Basque country: concentrations and accumulation trends. Food Chem (2015) 173:1083–9. doi: 10.1016/j.foodchem.2014.10.133
44. Machado RMA, Oliveira MRG. Tomato root distribution, yield and fruit quality under different subsurface drip irrigation regimes and depths. Irrigation Sci (2005) 24:80–96. doi: 10.1007/s00271-005-0002-z
45. Hamid Y, Tang L, Sohail MI, Cao X, Hussain B, Aziz MZ, et al. An explanation of soil amendments to reduce cadmium phytoavailability and transfer to food chain. Sci Total Environ (2019) 660:80e96. doi: 10.1016/j.scitotenv.2018.12.419
46. Defoe PP, Hettiarachchi GM, Benedict C, Martin S. Safety of gardening on lead- and arsenic-contaminated urban brownfields. J Environ Qual (2014) 43:2064–78. doi: 10.2134/jeq2014.03.0099
47. Attanayake CP, Hettiarachchi GM, Harms A, Presley D, Martin S, Pierzynski GM. Field evaluations on soil plant transfer of lead from an urban garden soil. J Environ Qual (2014) 43:475–87. doi: 10.2134/jeq2013.07.0273
Keywords: trace elements, urban agriculture, soil pollution, constructed Technosols, urban waste
Citation: Barbillon A, Lerch TZ, Araujo JHR, Manouchehri N, Robain H, Pando-Bahuon A, Cambier P, Nold F, Besançon S and Aubry C (2023) Recycling wastes to mitigate trace elements contamination in plants: a new horizon for urban agriculture in polluted soils. Front. Soil Sci. 3:1163356. doi: 10.3389/fsoil.2023.1163356
Received: 10 February 2023; Accepted: 02 May 2023;
Published: 19 May 2023.
Edited by:
Prafulla Kumar Sahoo, Central University of Punjab, IndiaReviewed by:
Rakesh Kumar, Independent researcher, Rajgir, IndiaSaloni Sachdeva, Jaypee Institute of Information Technology, India
Umakant Chaudhari, Central University of Punjab, India
Copyright © 2023 Barbillon, Lerch, Araujo, Manouchehri, Robain, Pando-Bahuon, Cambier, Nold, Besançon and Aubry. This is an open-access article distributed under the terms of the Creative Commons Attribution License (CC BY). The use, distribution or reproduction in other forums is permitted, provided the original author(s) and the copyright owner(s) are credited and that the original publication in this journal is cited, in accordance with accepted academic practice. No use, distribution or reproduction is permitted which does not comply with these terms.
*Correspondence: Anne Barbillon, YW5uZS5iYXJiaWxsb25AYWdyb3BhcmlzdGVjaC5mcg==