- 1School of Health and Life Sciences, Teesside University, Middlesbrough, United Kingdom
- 2National Horizons Centre, Teesside University, Darlington, United Kingdom
- 3Vindolanda, Hexham, Northumberland, United Kingdom
- 4Faculty of Arts and Humanities, Classics Department, University of Western Ontario, London, ON, Canada
Introduction: Soil at the Roman site of Vindolanda (Northumberland, UK) provides excellent preservation of wooden artefacts including Roman writing tablets.
Methods: In this study we examined chemical and microbial signature changes within varied occupation contexts of archaeological soil. Analysis included investigating elemental composition, sterol biomarkers, bacterial diversity and community structures from excavation trenches at Vindolanda using pXRF, GC-MS and 16S rRNA gene amplicon sequencing. Samples were taken from varying depths starting at topsoil and working down through layers of Roman occupation including one cavalry stable floor, two infantry barracks and a cook house, and layers which contained Roman writing tablets.
Results and Discussion: The chemical results indicate that areas where wooden artefacts were found had increased soil moisture which was also correlated with specific chemical conditions including shifts in iron, sulphur and phosphorous concentration. Steroid biomarkers indicate the presence of faecal matter in layers, supporting occupation descriptions. Overall microbial diversity did not change across the depth profile but was correlated with soil moisture. Anaerobic soils associated with more optimal preservation differed to other soils with increases in Firmicutes, Proteobacteria, Campilobacterota and Bacteroidota observed. Microbial community structure and putative function as revealed by PICRUSt2 is linked to occupation usage rather than depth of samples with laminated floor layers differing from turf structures. Understanding the complex processes within archaeological soil can help us to understand dynamics of decomposition and preservation. In addition, the apparent preservation of the environmental microbial community as well as the artefacts themselves allows us to understand the microbial environments of the past, how they relate to the present and what this means for our changing environments in the future.
1 Introduction
The Roman Auxiliary fort of Vindolanda (Northumberland, UK) is of considerable interest to archaeologists and historians keen to learn more about life at the edges of the Roman Frontier. The samples within this study were taken from a trench uncovered as part of the ‘Frontier in Transition’ project. The trench intersected nine occupation periods of the site, each one defined by a comprehensive demolition and subsequent rebuild of the fort and settlement. The first six periods of occupation, from c 85-160 CE, were principally constructed with timber and the final three, c 160-300 CE, more substantially with stone (1). The best-preserved layers are largely restricted to the earliest phases of occupation, each associated with one of five consecutive timber forts occupied between c 85 and c 130 CE. The information and artefacts contained within these layers provide first-hand records of Roman communities based at Vindolanda and Hadrian’s Wall.
In June 2017, the archaeological team encountered a remarkably well-preserved and tightly packed deposit of documents that were a part of the archive of the first commanding officer at Vindolanda. The deposit was situated in a build-up of fill on a street outside the western perimeter of the first fort at the site, built c. 85 CE. This layer, and several layers above, were anaerobic in appearance. Above this layer turf fill is found, levelling foundations for timber buildings and barracks dating 90-105 CE with laminated floor material (1–3). Above this well-preserved stable block containing oak kickboards and packed clay, organic waste and water pipes dating 105-118 CE are found (4). The layers above were waterlogged and contained the remains of a timber frame Hadrianic cook house (120-130 CE). Levels closest to the surface have lower moisture content and contain remains of a large industrial oven and furnace (dating to the end of 2nd century) sitting on a bed of clay which caps the lower layers, and a 3rd century cobbled street between two stone barracks.
Initial reading of the writing tablets found in 2017 suggest that they came from the residence of the first commanding officer at Vindolanda, Julius Verecundus, who is known from other tablets discovered at Vindolanda (5–7) and were perhaps part of his archive. The new tablet evidence neatly confirms what has previously been proposed: that the initial garrison quartered in the period II fort was the now enlarged Cohors I Tungrorum, still commanded by Julius Verecundus (1, 8, 9).
Environmental conditions at Vindolanda allows remarkable preservation of artefacts (10, 11). The preservation of the occupation layers within the trench allows a unique opportunity to apply scientific investigation to archaeological soils, further understanding the location and allowing linkage between observations made by archaeologists, and artefacts uncovered, with measurable environmental variables. Within this study we aim to use portable X-ray fluorescence (pXRF) for inorganic elemental analysis, gas chromatography-mass spectrometry for detection of steroid biomarkers and volatile organic compounds, and 16S rRNA gene amplicon sequencing to investigate soil parameters associated with specific forms of occupation. This will allow further understanding of the site and detail a scientific toolkit which can support archaeological investigation.
Compared to alternative analytical techniques, pXRF is substantially faster and cheaper, achieves comparable sensitivity, and provides the non-destructive portability desired for archaeological applications (12). pXRF has been used for differentiating occupation layers, areas of preservation, and identifying historic land uses (13–18). Elemental analysis of preservation at Vindolanda has previously focused on P, S and Fe (10, 11). P is consistently regarded as the best marker for preservation due to its association with anthropogenic activities (13, 17, 19–21). Generally, a low S content encourages good preservation whereas a high S content encourages corrosion and poor preservation (13, 22–25). Fe content is mainly dependant upon soil weathering and formation processes due to leaching from rock or structural remains. The high mobility of Fe through soil also makes it difficult to interpret through discreet layers of preservation and occupation.
Steroid biomarkers are useful fecal indicators which can differentiate between herbivore, human and omnivore fecal matter (26). Differentiation means a wide range of animals including horse, deer, cow, dog and human could be linked to different occupation layers (27). Diagnostic ratios are based upon a specific class of sterols known as 5β-stanols, which are also indicative of good preservation in organic matter. Sterols undergo microbial degradation in soil leading to transformation, for example, coprostanol to epicoprostanol (28, 29) More recently further biomarkers have expanded the fecal database in which to distinguish sterol signatures from a wider range of animals (27, 30). Identifying steroid biomarkers provides an opportunity for further understanding of Roman activities in occupation layers.
DNA-based techniques allowing the profiling of microbial communities within a location have been used increasingly to describe various soil environments due to its efficiency, cost effective and sensitive nature (31). However, within an archaeological context this is commonly limited to studying presence of pathogens and ancestral microbiota (32). To our knowledge, other than our previous study (10) there are no other studies detailing microbial communities within a Roman archaeological site. Instead, previous studies have linked microbial presence to deterioration of already excavated archaeological sites from different historical eras (33, 34). This study aims to characterize the microbial community within layers of well-preserved Roman occupation.
In non-archaeological settings microbial diversity would be expected to change within a depth profile. Whilst the majority of microbiological studies focus on the top 25 cm of soil there are still complex communities present at depth (35–37). However, these communities are less abundant and diverse than communities within the initial layers of a profile and correspond to declining soil C and N availability (38). Microbial communities also change as a result of differing land use and soil type with studies typically comparing ecosystems and demonstrating change due to anthropogenic intervention (39–41). Previous studies have shown that historical land use, over decades, can still result in changed bacterial and fungal communities today and that altered microbial signatures can be observed when land use has shifted from one anthropogenic form to another (42–44). Hermans et al. (43) were able to use microbial community profiles to accurately predict land use in 85% of cases when examining the bacterial communities alone. This impact may be less evident at depths below 90 cm (36).
Within this study we will firstly examine if the microbial community and chemical signatures within the Vindolanda site exhibits a typical depth profile. We will then aim to correlate the findings observed with the occupation layer and occupational indicators. This will allow greater confidence in the archaeological record to be determined and increased understanding of dynamics within a complex site. We hypothesise that the environmental change brought about by differing occupation settings will have led to an altered microbial community at the time. Given the level of environmental and artefact preservation seen at Vindolanda, we predict that the altered microbial community, and other biomarkers such as sterols, will still be detectable today. Understanding such changes in microbial community composition as a result of past land use can allow us to gain a clearer picture of soil dynamics at the time.
2 Methods
2.1 Sampling and archaeological context
Soil was sampled from a single trench in November 2017 at Vindolanda (54.9911°N 2.3608°W). Soils were extracted from 11 rows in 3 columns, with 10 cm between each row when possible, Figure 1.
To allow statistical analysis of data the archaeological context of soils was summarized as follows: Layer 0 and layer 10 as ‘natural soil’ as these layers contain no Roman artefacts or evidence of occupation. Layer 1, 6 and 9 as ‘packing’ as these layers contain disturbed turf and packing from buildings/street structures above. Layers 2, 5 and 7 as ‘turf’ as these layers show turf fill for building foundations. Layers 3 and 4 as ‘floor’ as these layers contain laminated floor materials. Layer 8 as ‘silt’ as this contains hard packed silt and cobble. Samples which were in Layers 0-4 were observed to be anaerobic in appearance, Layers 5-6 were waterlogged and Layers 7-10 showed neither anaerobic nor waterlogged appearance and have been termed ‘dry’ soils for analysis, see Figure 1.
2.2 Soil moisture and pH
Soil moisture and pH levels were measured in duplicate using standard methods of 24-hour oven drying and mixing in water respectively. Briefly, soils were weighed before and after drying in an oven overnight at 105°C to determine the moisture content of the original sample using Eq.
Soils (10 g) were placed into plastic cups with distilled water added (20 ml) for a 1:2 proportion. The solution was stirred with a glass rod and settled for 30 min. pH values was analysed using an Advanced Jenway 3510 pH meter calibrated with commercial buffers of pH 4, 7 and 10. The electrode probe was rinsed between each sample. Further details are found in (10).
2.3 Total carbon and total nitrogen
Total carbon analysis was carried out using Vario TOC and total nitrogen was carried out on the rapid N exceed instrumentation (Elementar Corp., Germany). All analysis was carried out in triplicate. Low organic content soil standard was obtained from Elemental Microanalysis, UK.
2.4 portable X-Ray fluorescence
Soils were analysed following the ex-situ method provided by (12). The dried soil samples were manually homogenised for 140 seconds with mortar and pestle and sieved to 2 mm. Soils were loaded into XRF sample cups (SPEX CertiPrep 3529) and closed with 5 µm polypropylene thin-film (SPEX SamplePrep 3520 window film). A Thermo Niton XL3t GOLDD+ pXRF with Ag anode (6-50 kV, 0-200 μA max X-ray tube) was warmed up and system-checked against the internal 1¼ Cr–½ Mo coupon prior to analysis. The pXRF was periodically reset and system-checked to account for drift. A blank standard and NIST 2709a standard (y=0.9674x -0.0089, r2 = 0.9998) were analysed at the start of scan sessions. The internal calibration factor was not altered. The NIST 2709a is a San Joaquin soil certified for most elements of interest in this research within the single calibrated sample. Soil samples were analysed using Mining mode (fundamental parameters), with 30-second scans for the main filter (50 kV, ≤50 μA), low filter (20 kV, ≤100 μA) and high filter (50 kV, ≤40 μA), and a 60-second scan for the light filter (6 kV, ≤200 μA). The “overall” number is the value of all detected elements combined and shows how much of the inorganic elemental portion of soil has been analyzed across all samples. The rest of the numbers are the detections of each element individually, provided as weight%.
2.5 Gas chromatography – mass spectrometry
2.5.1 Fecal steroid analysis
Total lipids were extracted from approximately 1 g of freeze-dried homogenised sediment using 5 ml DCM/MeOH (2:1 v/v) and using an ultrasonic bath for 30 min, this was repeated three times. Samples were centrifuged at 4 °C for 10 min at 4000 rpm to separate the solid pellet from the liquid fraction. The liquid fraction was removed and dried under a gentle stream of nitrogen gas. Following Bull et al. (45) extracts were saponified using 5M KOH in 90% MeOH, then separated into neutral and acid fractions using aminopropyl SPE columns (Phenomenex). The sterol fraction was derivatized by the addition of 50 µL pyridine and 50 µL N,O-Bis(trimethylsilyl) trifluoroacetaminde with 1% Trimethylchlorosilane (BSTFA + 1% TMCS), which was heated at 75°C for 30 min. GCMS analysis was preformed using a Waters TQ-GC. Chromatographic separation was performed on a Thames Restek (DB-5, 30 m x 0.25 mm ID x 0.25 µm). The following temperature parameters were used: 80°C to 265°C at 12°C min-1, then to 300°C at 10°C min-1, then held for 5 min. The analyzer was set to scan m/z 100-650. GCMS peaks were identified through comparison to standards and the NIST database.
2.5.2 Volatile organic compounds
Headspace Gas chromatography–mass spectrometry analysis was carried out using a TRACE GC 1300 gas chromatograph with ISQ quadrupole mass spectrometer (ThermoScientific Corporation). Chromatographic separation was performed on a ZB-1 (Phenomenex Ltd) fused-silica capillary column, (60 m x 0.25 mm x 1 µm). Oven temperature program was from 70°C (hold 2 min) to 300°C at 10°C min-1, hold for 5 min. The syringe temperature was 90°C, agitator was 60°C and the sample was held for 45 min in the agitator. The interface and the source temperatures were 250°C and 200°C, respectively. Electron impact mass spectra at 70 eV ionisation. The analyser was set to scan m/z 60-500. The mass spectrometer data acquisition was performed using Chromeleon 7.2.10. GCMS peaks were identified through comparison to standards and the NIST database and chemical standards.
2.6 DNA extraction
DNA was extracted from 0.25 g of each soil sample using the Qiagen DNeasy PowerSoil Kit following manufacturer’s instructions. DNA quality and yield were measured using NanoDrop 1000 spectrophotometer (V3.8.1, ThermoFisher) and agarose gel electrophoresis. DNA was stored at -80°C prior to analysis.
2.7 16S rRNA gene amplicon sequencing
The V4 region of the 16S rRNA gene was amplified using primers 515F (5’ GTGCCAGCMGCCGCGGTAA 3’) and 806R (5’ GGACTACHVGGGTWTCTAAT 3’). Resulting PCR products were subjected to pair-end 250 bp sequencing using Illumina NovaSeq 6000 platform (Illumina, San Diego, CA, USA) at Novogene (Beijing, PR China) at read depth of 30,000 tags per sample.
The raw sequencing reads were processed in FASTQ format and analyzed with DADA2 (version 1.16) and Bioconductor (Version 2) (46, 47). Reads were trimmed and filtered to remove data with a q score of <30. Forward and reverse paired reads were merged and clustered into Amplicon Sequence Variants (ASVs) (47). UCHIME was used to quality check and filter the FASTA formatted sequences for chimeras before assigning taxonomy using RDP14 reference database (48). Non-prokaryotic sequences (Eukaryota/Unclassified) were discarded.
2.8 Statistical analysis
Data were analysed in R 4.1.2 (49) using the car, DescTools and PMCMR packages (50–52). Data were analyzed using Kruskal-Wallis rank sum tests, followed by Nemenyi tests with Chi-Square distribution to identify significant differences between burial layers. Tukey box plots of the median and quartiles were produced using the ggplot2 package (53) to show the change in raw elemental concentration with increasing burial depth. The tidypaleo package in tidyverse (54) was used to produce stratigraphic plots of the moisture content and pH values against burial depth.
ASV tables and metadata were also analyzed using R. Samples were faceted to rarefy based on sequencing depth and ASV totaling less than 0.01% of the overall data set were removed, relative abundance data was compiled, and most abundant phyla and genera were sought. Stacked bar charts were created using ggplot (53) and reshape (55) and variation between groups was determined using significant differences identified using ANOVA and TukeyHSD tests within the readr package (56). Correlation was determined using Spearman Correlation analysis. NMDS with and without environmental variables were created using vegan version 2.6-2 and ggplot2 packages and Bray-Curtis distance matrixes. Significant affects were measured using ANOSIM. PICRUSt Heatmaps and dendrograms of the KEGG number were compiled using the base heatmap R package along with gplots (57). Taxa indicative of preservation were identified, and histograms generated following Linear Discriminant Analysis Effect Size (LEfSe) analysis completed in Galaxy (58).
2.9 Raw data
The raw sequencing data are accessible at the NCBI Sequence Read Archive (SRA) with the study accession number PRJNA928090.
3 Results
3.1 Environmental variables and inorganic measurements
pH values were comparable with previously published data from Vindolanda (10). Kruskal-Wallis tests showed no significant difference in pH level (χ2 = 84.79, df = 77, p = 0.25; Figure 2) between occupation layers. However, significant differences were observed between moisture content and different occupational layers (χ2 = 98, df = 32, p < 0.001; Figure 2).
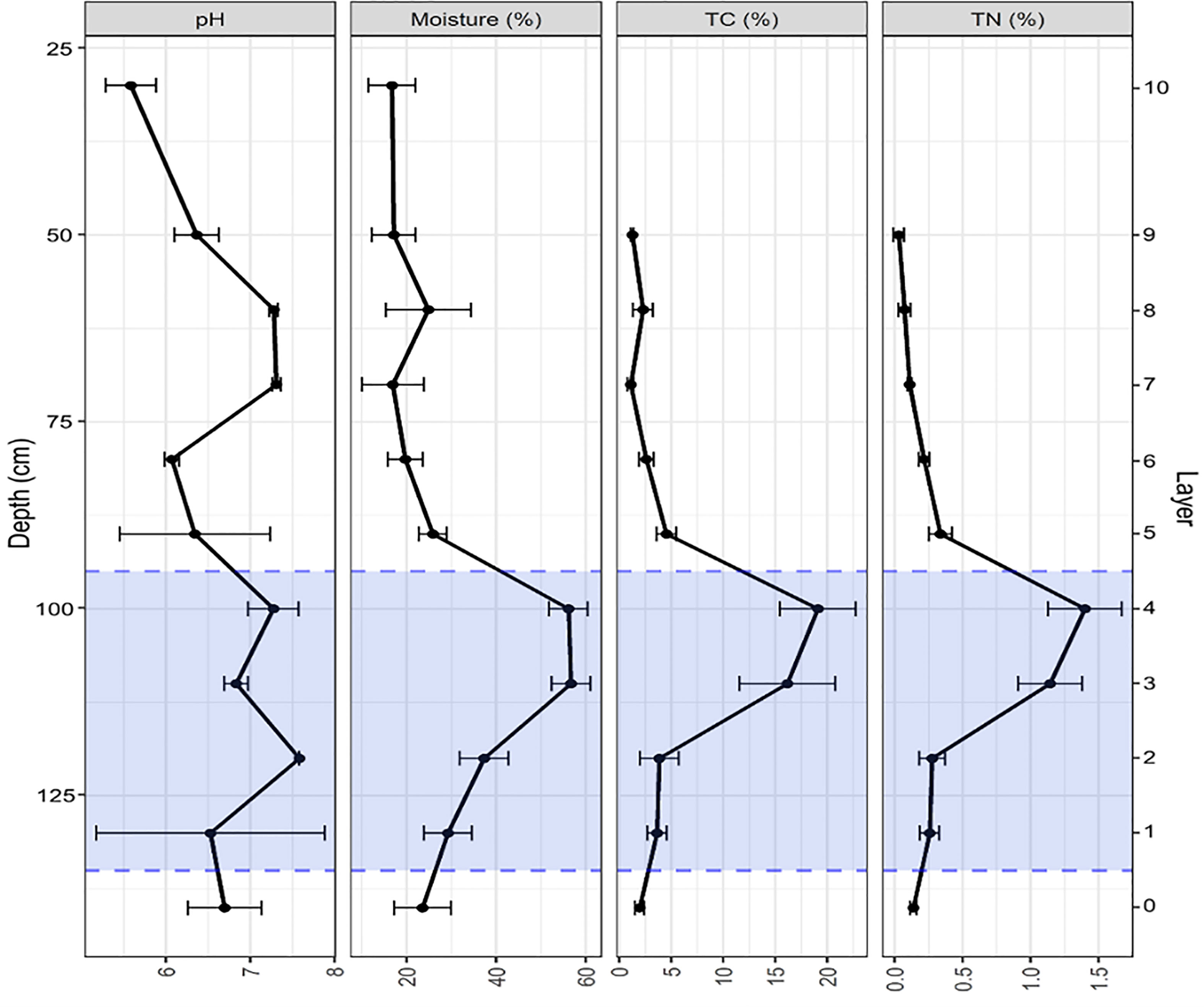
Figure 2 Summary of chemical data across layers. Error bars for pH, moisture, total nitrogen (TN), total carbon (TC) n = 2. The area in blue denotes the zone of vivianite formation observed (11).
Kruskal-Wallis tests showed a significant interaction between overall concentration and occupation layer (χ2 = 60.30, df = 10, p < 0.001; Figure 3), with Kruskal-Wallis tests showing all elements except Mg were individually significantly different between layers. The combination of P, S and Fe content, with support by other elements, showed elemental concentration that supported the layers of preservation at Vindolanda. This supported the significant interactions between these three elements and decreasing burial layer (F10,88 = 117.21, p < 0.001 after log transformation).
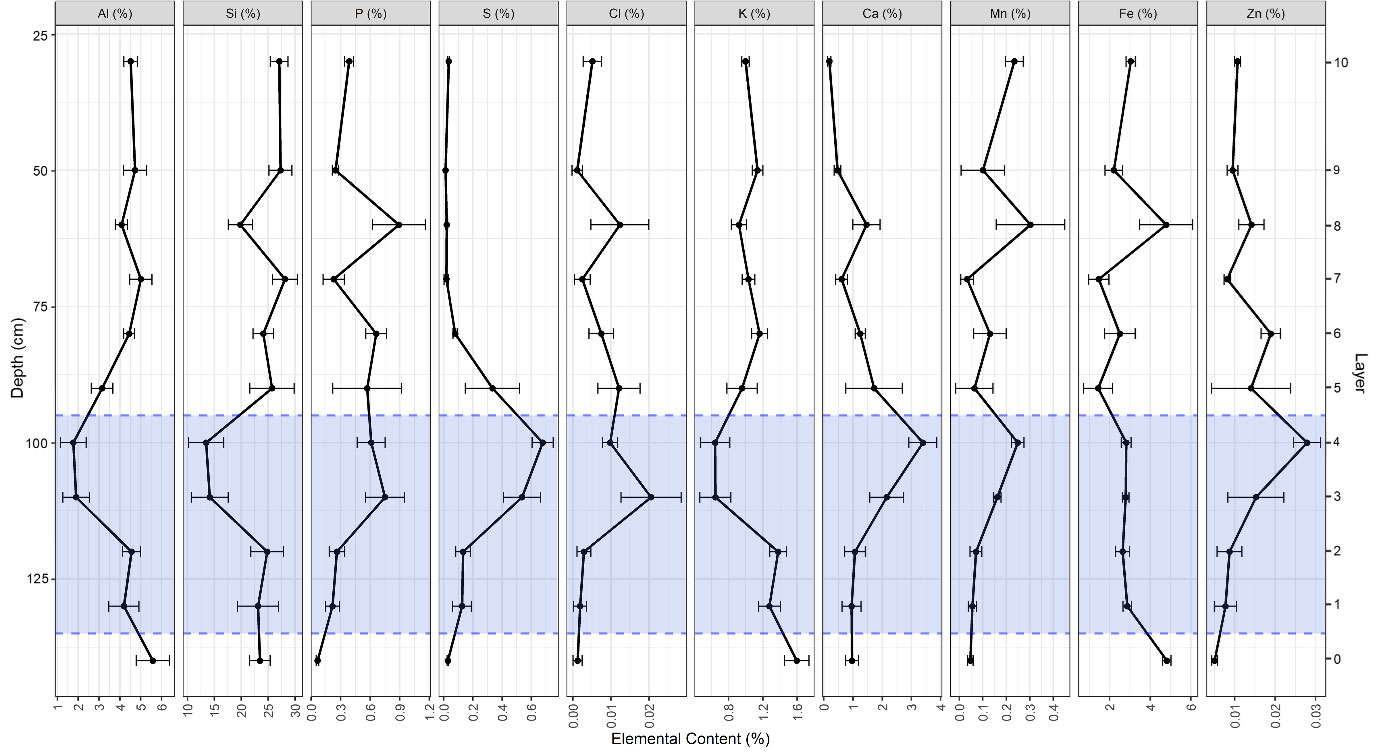
Figure 3 Elemental concentration of Al, Si, P, S, Cl, K, Ca, Mn, Fe and Zn. The area in blue denotes the zone of vivianite formation observed (11).
3.2 Fecal steroid analysis
Faecal sterols were determined from the occupation layers, confirming and identifying the presence of animals, such as horses in specific occupation layers. The total sterol composition differed between occupation layers. Period I evidence within layer 1 and 2 indicated faecal input (>0.7), with low percentages indicating herbivore faecal input. Layer 1 and 2 were contained within an anaerobic environment and display increased epi-5β-stanols (29). Layer 3 and 4, were dominated by period II to IV (92-120 CE), indicated as barracks and stable. However, the faecal input in layer 3 (period III) and 4 (period IV) were inconclusive, showing <0.7 for faecal matter content. It is interesting to consider layer 5 (period V, 120-130 CE) which is an extremely complex layer, the archaeological evidence suggests a cook house, where mixed animal faecal matter and animal fat may be present. Again, as expected in an anaerobic layer, the epi-5β-stanols were increased for this layer.
3.2 Alpha and Beta diversity using 16S rRNA gene amplicon sequencing
16S rRNA gene profiles showed microbial diversity, measured using Shannon Diversity index (H’), which did not significantly change across the soil profile with H’ varying between the lowest average value of 4.62 in layer 5 and the highest average value of 6.18 in layer 9. Shannon diversity significantly differed when comparing layers 0 and 9 with layers 1, 2 and 5 (Figure 4B). Diversity differed significantly when correlating H’ with Total C (ρ = -0.845 and P = 0.002) and H’ with moisture (ρ = -0.755 and P = 0.010).
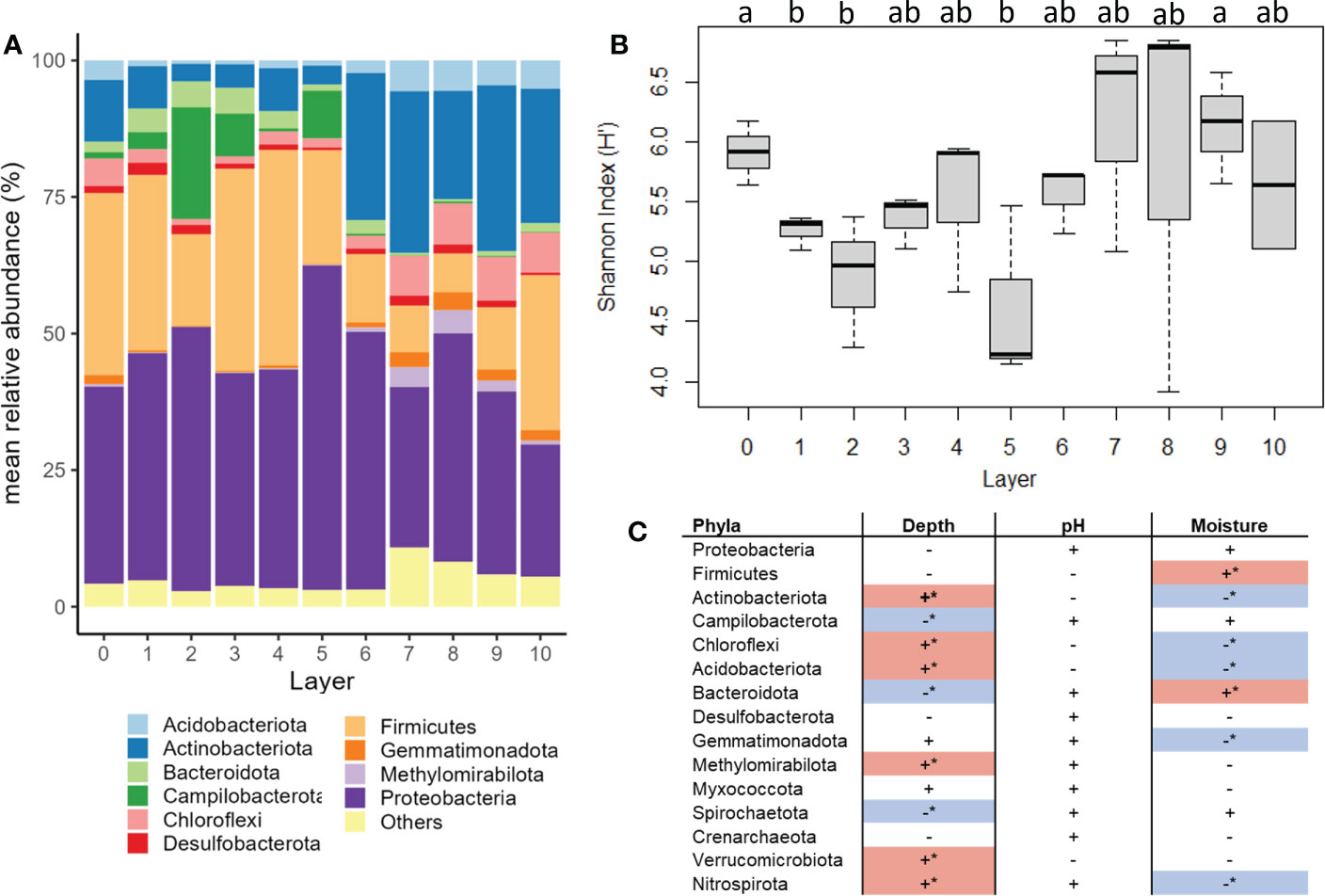
Figure 4 (A) Mean relative abundance of most abundant phyla across each of the site layers. (B) Changes in Shannon index with soil depth. Differences were compared using Wilcoxon test adjusted for false-discovery rate. A P value of <0.05 was considered statistically significant. Different letters above bars indicate significant differences between soil depths. Lines in boxes represent medians. The top and bottom of each box represent the first and the third quartiles, respectively. Whiskers indicate data ranges. (C) Spearman Correlation analysis showing correlation of relative abundance of most abundant phyla with depth, pH and moisture. Significant correlations are shown with an * and highlighted in red for positive correlation and blue for negative correlation.
Phyla within the different layers varied (Figure 4A). When the most abundant phyla were compared between layers it was found that several phyla varied with depth with Actinobacteria, Chloroflexi, Acidobacteriota, Methylmirabilota, Verrucomicrobiota and Nitrospirota increasing significantly with depth and Campilobacterota, Bacteriodota and Spirochaetota decreasing significantly with depth (Figures 4A, C). Changes in pH did not correlate with relative abundance of phlya. Moisture availability within the samples, which was increased in anaerobic layers 3 and 4 particularly, was correlated positively with significantly increased Firmicutes and Bacteroidota and correlated negatively with significantly increased Actinobacteriota, Chloroflexi, Acidobacteriota, Gemmatimonadota and Nitrospirota (Figure 4C).
NMDS showed significant differences between the community profiles linked to soil type (anaerobic, waterlogged or dry) (P > 0.001), layer depth (P < 0.001), and occupation status (P = 0.022) (Figure 5A). The impact of environmental variables pH (P = 0.007), moisture (P = 0.003), S (P=0.023) and total C (P=0.023) were seen to impact significantly on microbial community structure with moisture and sulphur concentration S associating with anaerobic and dry soils (Figure 5B).
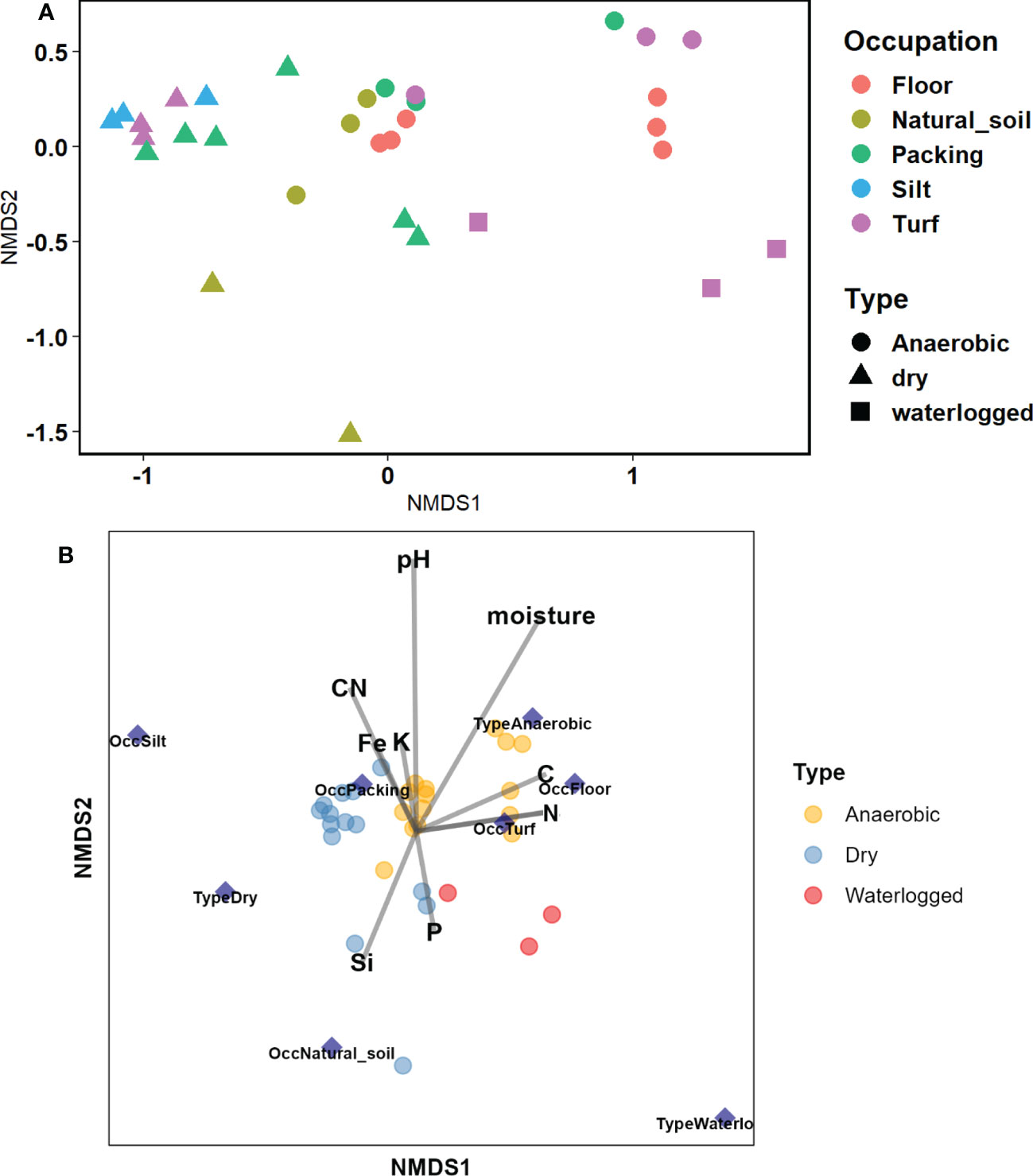
Figure 5 (A) NMDS using Bray distance matrices. Shape of points represents soil type and color of points represents occupation layer. (B) NMDS using Bray distance matrices with environmental variables overlayed. Points are colored based on soil types. Centroid shows center point for Occupation of soils (Occ) and soil type. Stress value = 0.1064.
3.3 Putative functional analysis using PICRUSt
PICRUSt was used to infer functionality from 16S rRNA gene profiles. The most abundant inferred KEGG matches did not show clustering based on sample depth (Figure 6) with layers 1, 2 and 5, the least diverse of the locations, showing the most marked difference. Clusters are observed separating layers 1, 2 and 5 (cluster 1) which are observed to contain buried turf, layers 3, 4 and 10 (cluster 2) which are the laminate and topsoil layers and layers 0, 6, 7, 8 and 9 (cluster 3) which contain clay. Cluster 1 showed increased likelihood of presence of putative proteins with KEGG Numbers K01995-k01999, protein associated with branched-chain amino acid transport, K00799 gluthathione S-transferase associated with xenobiotic metabolism, K02014, an iron complex transport system protein, K02029 an amino acid transport protein and K00249 an acyl-CoA dehydrogenase associated with lipid metabolism. Cluster 2 showed increased likelihood of presence of putative proteins with KEGG numbers K02031-K02035 peptide/nickel transport system proteins, K02025-K02027 multiple sugar transport system proteins, K02030 amino acid transport protein, K02003-K02004 ABC transport system proteins, K06147 ATP-binding cassette proteins, K03406 methyl accepting chemotaxis protein, K02015 iron complex transport protein and K02529 LacI transcription regulator protein. Cluster 3 showed increased likelihood of presence of putative proteins with KEGG numbers K02003-K02004 ABC transport system proteins, K00626 acetyl-coA C-acetyletransferase proteins, K01897 acyl-coA synthetase, K03704 cold shock proteins, K0915 glutamine synthetase proteins, K07165 transmembrane sensor protein and K02002 glycine betaine/proline transport system proteins.
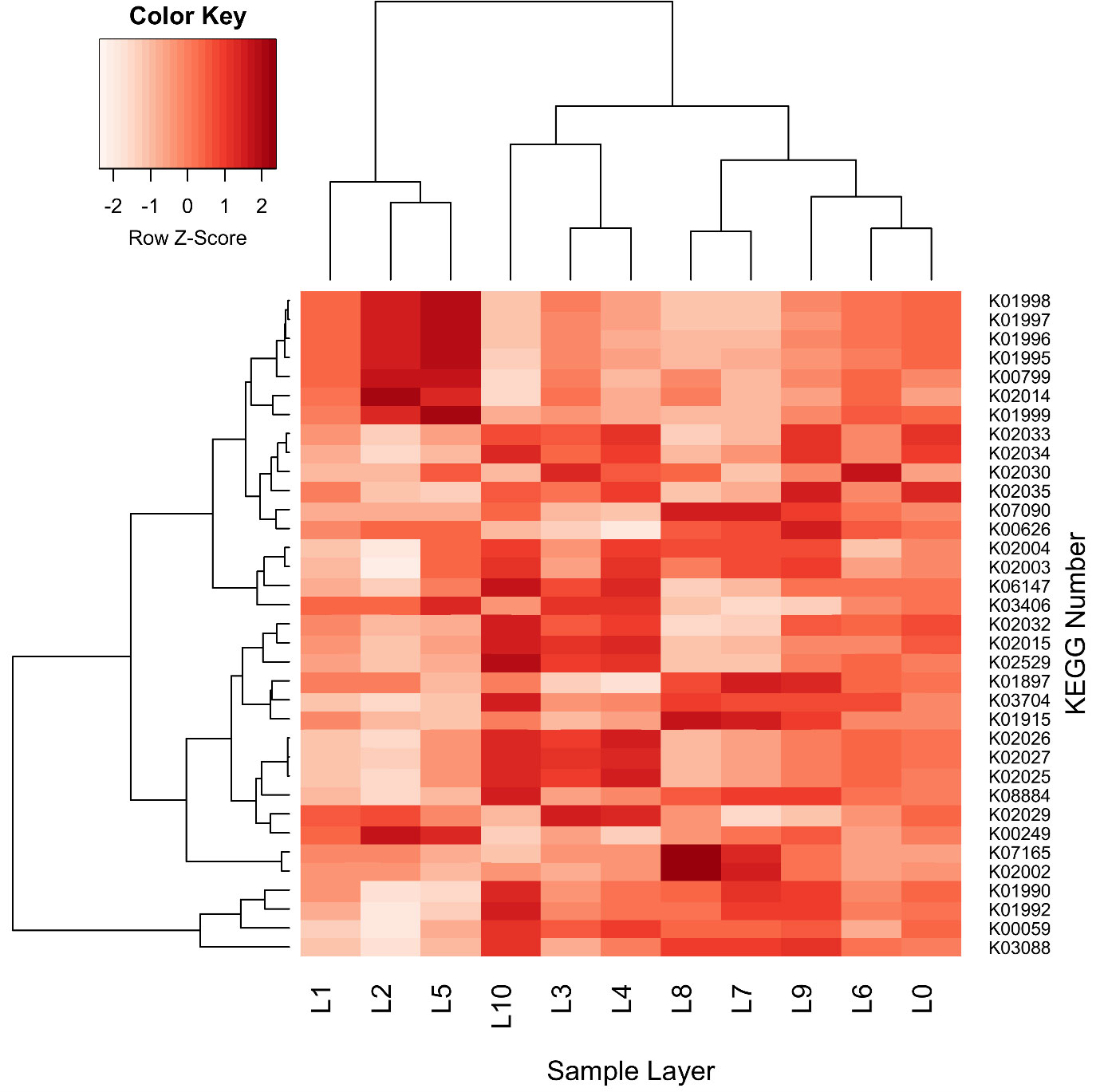
Figure 6 Heatmap showing most abundant likely KEGG numbered proteins associated with samples as inferred using PICRUSt. Soil layers are clustered into three groups: cluster 1, cluster 2 and cluster 3.
3.4 Microorganisms indicative of occupation layers
LEfSe analysis showed key phyla associated with occupation layers and soil type. At the Phyla level Gemmatimonadota, Desulfobacterota, Bacteroidota, Verrucomicrobiota, Planctomycetota, Patescibacteria, Acidobacteriota, and Crenarchaeota are all associated with dry soil within the upper layers. No Phyla were specifically associated with anaerobic or waterlogged layers. Significant genera within dry layers were the Bacteroidota Williamwhitmania and Acetobacteroides, Proteobacteria Aquaspirillum, Methylocystis and Sulfuricella and Desulfocapsa Desulfobacterota. Significant genera within anaerobic layers were the Firmicutes Erysipelothrix and Trichoccus, Proteobacteria Klebsiella, Comamonas and Herbaspirillum and Actinobacteriota Demequina (Supplementary Data Figure 2).
Specific Phyla associated with occupation layers were Bdellovibrionota, Bacteroidota and the Archaeal Crenarchaeota increased in silt within layer 8. Genera associated with occupation layers were Proteobacteria Vogesella, Rhodobacter and Comamonas associated with natural soil in layers 0 and 10, Proteobacteria Herbaspirillum and Archaeal Halobacterota Methanocella associated with floor layers 3 and 4 and Firmicutes ErysipelotrichaceaeUCG and Actinobacteriota Haloactinopolyspora associated with silt layer 8 (Figure 7).
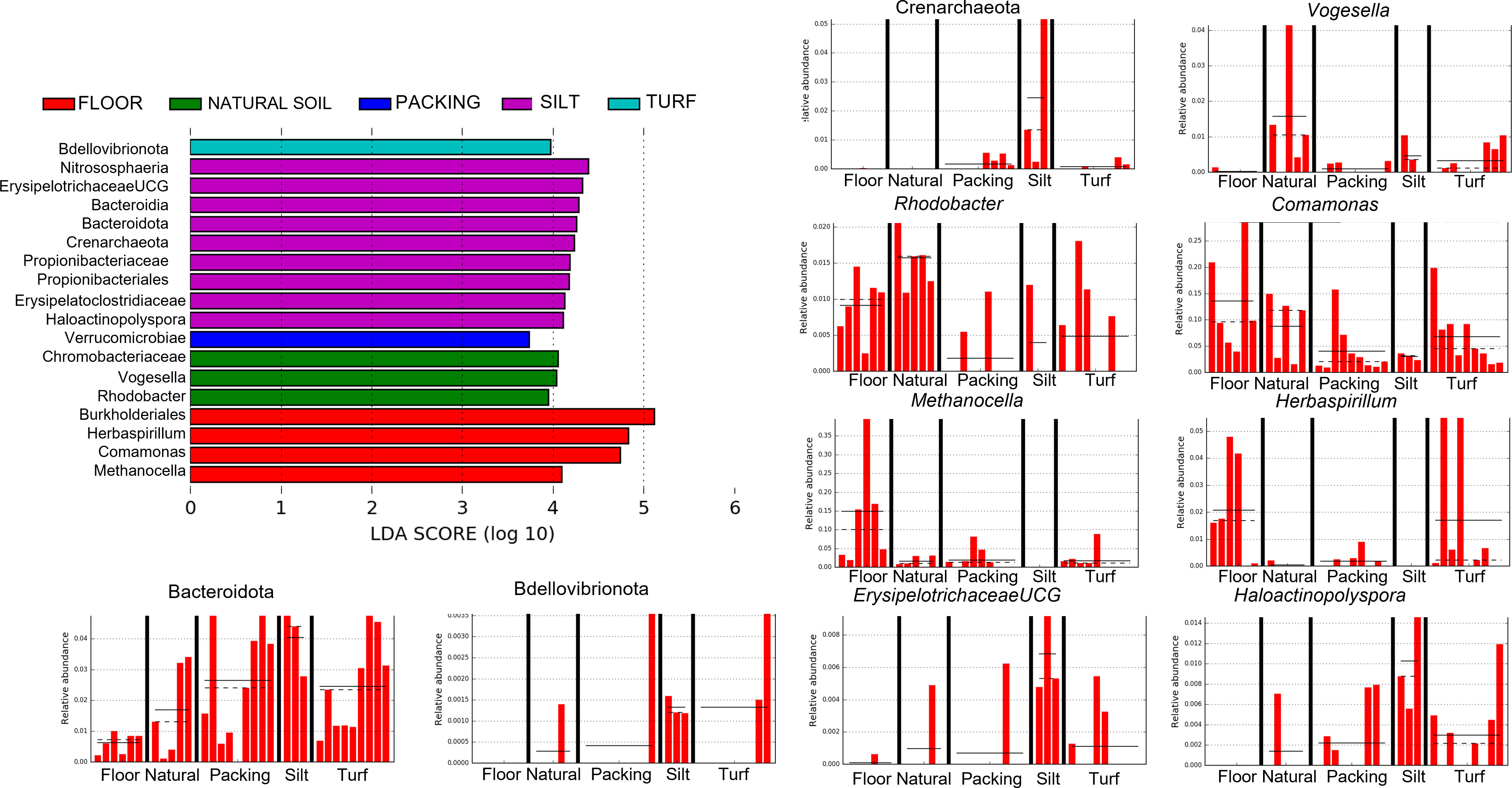
Figure 7 LEfSe analysis showing microbes indicative of occupational layers (top left). Relative abundance of significant Phyla and Genera shown in individual bar charts.
4 Discussion
4.1 Elemental distribution and preservation
The collection of artefacts and a wealth of anecdotal evidence from over 50 years of excavations at Vindolanda have reformed the knowledge of the Northern Frontier. These have survived due to complicated preservation processes that are not fully understood.
The pH level was stable throughout each layer however, significantly higher moisture content was observed with increasing depth. Elemental composition differed between the layers. In increased moisture layers, 3-5, there is increased S, decreased K and increased in Ca. Previously we have observed the same changes in S, K and Ca elemental composition associated with layers of good artifact preservation at Vindolanda (10). However, in layers 1 and 2, which contained the writing tablets, there is an observational change between all measured elements, the significance of this upon preservation, especially for wooden artefacts requires further investigation. Understanding this further could help determine a range of acceptable and measurable chemical parameters to show conditions which are favorable for wooden artefact preservation.
4.2 Fecal biomarkers and occupation
Triplicate sampling from Layers 1-2 (earliest Roman occupation) displayed significant concentration of fecal biomarkers (Table 1). There was evidence of horse fecal matter in layer 1 and 2, this correlated with visual excavation observations made by archaeologists at the site, which was backfill, debris, and an active path into the commander’s quarters. Furthermore, all samples were analyzed by headspace-gas chromatography-mass spectrometry (see Supplementary Data Figure 1). Interestingly, only layer 1 displayed significant concentrations of α-pinene and β-pinene. Pinenes are a class of monoterpenoids often found in plants and α-pinene and β-pinene vary in concentration within plants throughout the year (60) The detection and significant concentration of these monoterpenoids tentatively allows us to explore occupation seasonality. For example, the concentration of monoterpenoids varies with season, with many plants only displaying significant concentration of α-pinene in summer periods (61). Furthermore, the detection of significant monoterpenoids supports the archaeological evidence that this layer was the result of a short occupational series, rapidly abandoned and covered with clay. The sealing of this layer coupled with the wet anaerobic conditions, prevented the expected release of the volatile terpenoids. In layer 4, the excavation uncovered kick boards often associated with stables; however, fecal matter especially indicating horse fecal matter was not determined. Further work is being undertaken to exploit information from bile acids and alkanes in these layers.
4.3 Microbial community structure associated with depth
Understanding microbial activity is required for determining soil dynamics and preservation of artefacts at Vindolanda. The microbial community has been shown to change across a depth profile in a variety of different soil types (36, 62–65). This is comprehensively reviewed by (38). Typically, microbial richness decreases with depth (36, 37) with changes in community structure linked to nitrate availability (62), soil organic matter content (36) pH, and potassium availability (65).
Within this study we did not observe significant change in overall microbial diversity with depth. However, we observed increases in relative abundance of Actinobacteriota, Chloroflexi, Acidobacteriota, Verrucomicrobiota, Nitrospirota and Methylomirabilota with depth. Samples at shallower depths were more likely to contain Campilobacterota, Bacteriodota and Spirochaetota. This trend is similar to that observed by Prada-Salcedo et al. (66) across a depth profile within temperate European forests with the authors suggesting that the similar bacterial profiles observed at changing depth to be a result of the dominance of fungal communities within the ecosystem. Fungal communities were not measured in the current study but would have given increased insight into degradation capabilities within Vindolanda soil. Presumably the lack of microbial diversity change with depth observed here is due to more consistent soil nutrient availability within the layers. We assume that this is due to the preservation of the environments leading to increased nutrient availability than that usually observed in deeper soils.
In general, the soils at Vindolanda have found to be low in Acidobacteria (10). Global reference soil standards investigated by Janssen (67) and Dunbar et al. (68) show abundances of Acidobacteria between 10 and 50%, Acidobacteria are often correlated with pH variation which is shown to be relatively stable within this study. It would be expected that aerobic copiotrophic microbes such as Proteobacteria, Bacteroidota and Arthrobacter would be more associated with shallower soils due to increased oxygen availability and decreased soil compaction. The presence of these microbes would usually promote increased microbial transformation of nitrogen, carbon and sulphur (65).
We have observed increased relative abundance of Firmicutes and Bacteroidota within the anaerobic preservation layers at Vindolanda previously (10). However, in the same study we associated reductions in Actinobacteriota with soils showing increased preservation, a trend which is not observed here. Actinobacteria function and survive more efficiently than other phyla in resource-limited environments (69). Within the soils associated with optimal preservation (layers 2-5) we continue to see microbes such as Verrucomicrobiota which have a diverse range of carbohydrate degrading abilities (70) and their presence shows the potential for methane cycling and nitrite reduction within the environment (71). The increase in Nitrospirota shows potential for further nitrite oxidation and interaction with sulphur species (72).
As well as microbial richness bacterial and archaeal Shannon diversity index has also been shown to decrease with depth (64) often correlated with decreasing soil organic carbon. Within this study we did not see this correlation. However, this is possibly due to the high clay content in the upper layers. Typically, clay content and compaction increased with depth (73) impacting on soil physical characteristics and microbial diversity (64). Whilst the H’ did not correlate with depth here it did show significant correlation with moisture availability and total C possibly as a result of the various occupation layers of the site.
4.4 Occupation layer significantly impacted on microbial community structure
To allow for analysis occupation layers were broadly grouped depending on their characteristics. Different occupation layers showed significant clustering within NMDS which is not as a result of depth due to the differing occupation types being distributed throughout the profile. This was also observed within putative functional analysis carried out using PICRUSt and LEfSe. This could suggest that the occupation layer itself is impacting on the microbial community structure. The putative proteins shown to be increased in cluster 1 associated with turf layers show increased processes which are associated with plant health. Amino acid uptake has been demonstrated to increase in turf soil as the plants demand for nitrogen increases (74, 75). Iron complex transport has also been demonstrated by microbes associated with turf as they provide Fe to the plant root (76). Further analysis of microbes associated with preserved turf samples could begin to show how past cultures managed their land to ensure good growth.
ABC transporters are commonly found across all forms of life with soil microbes utilizing them to transport a range of compounds across their membranes (77). The varied dominance of difference ABC and sugar transporters within clusters 2 and 3 corresponding to laminate and clay layers could show the microbes responding to the varying nutrients available within the different layers. When adding lignin containing material like that of the laminate observed at Vindolanda to microbial communities Zhu et al. (78) found increased ABC transport and methyl accepting chemotaxis proteins similar to those found in this study. These results suggest that the preserved wooden artifacts at Vindolanda are impacting on the microbial community within the soil. As wooden artefacts are so well preserved at Vindolanda it would be interesting to compare these bacterial communities, and the fungal communities usually associated with wood degradation, within the preservation layers to adjacent, non-preserving soil.
There are few examples of microbial sequencing within archaeological sites. McNamara et al. (79) examined six samples from one room of the Mayan acropolis and found microbial communities associated with the surface and interior of the limestone ruins. Zanardini et al. (80) examined triplicate samples of two locations of one exfoliated stone window at Portchester Castle and found bacterial and archaeal communities within the stone pores. Both McNamara et al. (79), and Zanardini et al. (80), proposed the role of the microbes identified in degradation and weathering of the stone artefacts. Sun et al. (81) and Wang et al. (34), analyzed the microbial community of of soil samples found within the Liangzhu City archaeological site. Both studies found microbial communities taken from site locations differed to control samples and discussed the potential role of microbes within preservation and degradation of the artefacts. The work within the current studies supports these findings and shows that the presence of archaeological remains has an impact on the microbial communities present at a given site. What is unknown is the link such microbes have on the preservation and degradation of the artefacts themselves. Understanding this further could allow the identification of key taxa which could be seen as favorable or detrimental to archaeological preservation. Monitoring such taxa against changing climatic conditions could allow indications of environmental change leading to increased deterioration of artefacts in situ and the need for conservation action to be taken.
Our previous work at Vindolanda (10) showed marked differences in microbial profiles associated with occupation compared to a lack of occupation. Margesin et al. (69) showed that microbial profiles shifted related to locations within a 6th century archaeological site buried at 90 cm depth. Here samples associated with a fireplace differed to those within areas used for food waste disposal. Expanding the combined approach of chemical and microbial mapping will be invaluable for future research.
Within this study we found phyla and genera associated with specific occupation layers. Herbaspirillum and Methanocella were increased within laminate floor layers. Methanocella are methanogenic and usually associated with rice-field soils (82). The anaerobic conditions at Vindolanda could favour the growth of such microbes allowing methane production within the soils. Herbaspirillum is a nitrogen fixing bacteria known to colonise plant material and find conditions of increased phosphorus favourable (83).
We have identified Vogosella, seen here to be increased within the natural soil layers, previously at Vindolanda. Previously, Vogosella were associated with areas of good preservation (10). The environmental conditions allowing preservation of artefacts are allowing proliferation of different microbes at different layers. Identifying microbes which are indicative of areas of preservation and occupational contexts will allow further understanding of processes occurring in the soil.
Studies of archaeological sites questions whether we are observing a microbial community from the present which are still responding to the artefacts and environmental conditions they are surrounded by or are we observing a microbial community which is representative of activities associated with the past and the period in which the occupation was taking place. The former seems the most intuitive. However, other studies have detected microbes which are assumed to be associated with the artefacts at time of burial. Some recent studies have aimed to identify ancient microbes with energy-starved prokaryotes being shown to exist for thousands of years within marine sediments (84). The authors attributed this action to the production of chemical protectants which allowed biomolecular stabilization. It is thought that certain metabolic pathways, particularly those associated with sulfate reduction, fermentation and methanogenesis may still be detectable over long time frames and have environmental impact on nutrient cycling and gas release today (85). Live yeast cultures have been isolated from ancient vessels dating to Egyptian and Bronze age (86). Weyrich et al. (87) detected Methanobrevibacter within the teeth of a 40,000 year old Neanderthal which were thought to have colonized at the time. When different aged Siberian permafrosts were studied ageing between 33,000 and 1.1 ma younger sediments were found to be dominated with Actinobacteria and Proteobacteria and older sediments with spore-forming firmicutes potentially confirming the resilience of certain microbes over others (85). Tentatively, the linkage of microbial communities to occupation type within this study may show that signatures of the community present at the time of occupation may still be evident. Regardless, at Vindolanda the level of preservation observed within the occupation layers allows the original environmental conditions needed to support microbial communities to be preserved. This results in a microbial community which is does not simply result from depth profile change.
5 Conclusion
We observed varying environmental and microbial signatures within the soil profile. Whilst some of these responded to depth and soil type trends showing correlation with occupation were also observed. Further investigation of archaeological soils could allow us to understand the relationship between soils and the artefacts that reside within them. Utilizing surviving chemical and microbial indicators in the soil increases the confidence archaeologists have in their understanding of the design and function of spaces allowing further sense checking and opening up new avenues of investigation. Understanding microbial and chemical pathways can improve excavation practices and aid our knowledge of preservation processes upon artefacts. Crucially, further work understanding how changing environmental conditions, for example those associated with climate change, impact on the activity of key microbes associated with artefacts preservation within the ground could allow for timing of excavation and storage of artefacts to be optimized. Finally, if we can use ancient soils to see glimpses of microbes from the past this may allow us to understand nutrient cycling and processes happening at the time. This allows for a more detailed picture of past environmental conditions to be gleaned and allows us opportunities to understand how our environments may change in the future.
Data availability statement
The data presented in the study are deposited in the NCBI SRA repository, accession number PRJNA928090.
Author contributions
GT and CO wrote the first draft of the manuscript and conducted sample collection and data analysis. RW, HH, AC prepared and analyzed the elemental and biomarker samples. AB was involved in the fieldwork and sample collection. AB and AM drafted the text relating to the writing tablets. All authors contributed to the article and approved the submitted version.
Acknowledgments
The authors thank The Vindolanda Trust for providing access to the archaeological samples. There are no conflicts of interest to declare. The data that support the findings of this study are available in the additional supporting information.
Conflict of interest
The authors declare that the research was conducted in the absence of any commercial or financial relationships that could be construed as a potential conflict of interest.
Publisher’s note
All claims expressed in this article are solely those of the authors and do not necessarily represent those of their affiliated organizations, or those of the publisher, the editors and the reviewers. Any product that may be evaluated in this article, or claim that may be made by its manufacturer, is not guaranteed or endorsed by the publisher.
Supplementary material
The Supplementary Material for this article can be found online at: https://www.frontiersin.org/articles/10.3389/fsoil.2023.1129040/full#supplementary-material
References
1. Birley R. Vindolanda: Everyday life on rome’s northern frontier. Gloucestershire: Amberley Publishing (2009).
3. Birley A, Blake J. Vindolanda: The excavations of 2003/2004. Bardon Mill: The Vindolanda Trust Publications (2005).
5. Bowman AK, Thomas JD. A military strength report from vindolanda. J Roman Stud (1991) 81:62–73. doi: 10.2307/300489
6. Birley E, Birley R, Birley A. Vindolanda: Volume II: The early wooden forts. Bardon Mill: Roman Army Museum Publications. (1993).
7. Bowman AK. Life and letters on the Roman frontier: Vindolanda and its people. London: British Museum Press (1994).
9. Birley A R. Roman Roadworks near vindolanda and the cohors I tungrorum. Britiannia (2017) 48. doi: 10.1017/S0068113X16000532
10. Orr CH, Williams R, Halldórsdóttir HH, Birley A, Greene E, Nelson A, et al. Unique chemical parameters and microbial activity lead to increased archaeological preservation at the Roman frontier site of vindolanda, UK. Sci Rep (2021) 11(1). doi: 10.1038/s41598-021-94853-7
11. Taylor G, Hefford RJW, Birley A, Huntley JP. Identifying the “blue substance” at the Roman site of vindolanda, Northumberland. J Archaeological Sci.: Rep (2019) 24:582–7. doi: 10.1016/j.jasrep.2019.01.030
12. Williams R, Taylor G, Orr C. pXRF method development for elemental analysis of archaeological soil. Archaeometry (2020) 62(6):1145–63. doi: 10.1111/arcm.12583
13. Williams R, Errickson D, Taylor G. Mapping an archaeological site: Interpreting portable X-ray fluorescence (pXRF) soil analysis at boroughgate, skelton, UK. J Archaeological Sci.: Rep (2021) 38. doi: 10.1016/j.jasrep.2021.103109
14. Smejda L, Hejcman M, Horak J, Shai I. Ancient settlement activities as important sources of nutrients (P, K, s, zn and Cu) in Eastern Mediterranean ecosystems – the case of biblical tel burna, Israel. Catena (2017) 156:62–73. doi: 10.1016/j.catena.2017.03.024
15. Fleisher J, Sulas F. Deciphering public spaces in urban contexts: Geophysical survey, multi-element soil analysis, and artifact distributions at the 15th-16th-century AD Swahili settlement of songo mnara, Tanzania. J Archaeological Sci. (2015) 55:55–70. doi: 10.1016/j.jas.2014.12.020
16. Cannell RJS, Gustavsen L, Kristiansen M, Nau E. Delineating an unmarked graveyard by high-resolution GPR and pXRF prospection: The medieval church site of furulund in Norway. J Comput Appl Archaeol. (2018) 1(1):1–18. doi: 10.5334/jcaa.9
17. Migliavacca M, Pizzeghello D, Ertani A, Nardi S. Chemical analyses of archaeological sediments identified the ancient activity areas of an iron age building at rotzo (Vicenza, Italy). Quaternary Int (2013) 289:101–12. doi: 10.1016/j.quaint.2012.07.016
18. Middleton WD. Identifying chemical activity residues on prehistoric house floors: A methodology and rationale for multi-elemental characterization of a mild acid extract of anthropogenic sediments. Archaeometry (2004) 46(1):47–65. doi: 10.1111/j.1475-4754.2004.00143.x
19. Nielsen NH, Kristiansen SM. Identifying ancient manuring: Traditional phosphate vs. multi-element analysis of archaeological soil. J Archaeological Sci. (2014) 42(1):390–8. doi: 10.1016/j.jas.2013.11.013
20. Gall M J. It’s elemental! a case study in the use of multi-element geochemical analysis as an aid in locating cultural features at the foundation site. Northeast Historical Archaeol. (2012) 41(1). doi: 10.22191/neha/vol41/iss1/6
21. Holliday VT, Gartner WG. Methods of soil p analysis in archaeology. J Archaeological Sci. (2007) 34(2):301–33. doi: 10.1016/j.jas.2006.05.004
22. High K, Milner N, Panter I, Penkman KEH. Apatite for destruction: Investigating bone degradation due to high acidity at star Carr. J Archaeological Sci. (2015) 59:159–68. doi: 10.1016/j.jas.2015.04.001
23. Kibblewhite M, Tóth G, Hermann T. Predicting the preservation of cultural artefacts and buried materials in soil. Sci Total Environment (2015) 529:249–63. doi: 10.1016/j.scitotenv.2015.04.036
24. Caple C. Towards a benign reburial environment: the chemistry of the burial environment. Conserv Manage Archaeological Sites (2004) 6(3/4):155–66. doi: 10.1179/135050304793137801
25. Tiedje JM, Sexstone AJ, Parkin TB, Revsbech NP. Anaerobic processes in soil. Plant Soil (1984) 76(1-3):197–212. doi: 10.1007/BF02205580
26. Bull ID, Simpson IA, Dockrill SJ, Evershed RP. Organic geochemical evidence for the origin of ancient anthropogenic soil deposits at tofts ness, sanday, Orkney. Organic Geochem. (1999) 30(7):535–56. doi: 10.1016/S0146-6380(99)00020-0
27. Harrault L, Milek K, Jardé E, Jeanneau L, Derrien M, Anderson DG. Faecal biomarkers can distinguish specific mammalian species in modern and past environments. PloS One (2019) 14(2). doi: 10.1371/journal.pone.0211119
28. Prost K, Birk JJ, Lehndorff E, Gerlach R, Amelung W. Steroid biomarkers revisited - improved source identification of faecal remains in archaeological soil material. PloS One (2017) 12(1). doi: 10.1371/journal.pone.0164882
29. McCalley DV, Cooke M, Nickless G. Effect of sewage treatment on faecal sterols. Water Res (1981) 15(8):1019–25. doi: 10.1016/0043-1354(81)90211-6
30. Mackay H, Davies KL, Robertson J, Roy L, Bull ID, Whitehouse NJ, et al. Characterising life in settlements and structures: Incorporating faecal lipid biomarkers within a multiproxy case study of a wetland village. J Archaeological Sci (2020) 121. doi: 10.1016/j.jas.2020.105202
31. Thomsen PF, Willerslev E. Environmental DNA - an emerging tool in conservation for monitoring past and present biodiversity. Biol Conserv (2015) 183:4–18. doi: 10.1016/j.biocon.2014.11.019
32. Warinner C, Herbig A, Mann A, Fellows Yates JA, Weiß CL, Burbano HA, et al. A robust framework for microbial archaeology. Annu Rev Genomics Hum Genetics: Annu Rev Inc. (2017) 18:321–56. doi: 10.1146/annurev-genom-091416-035526
33. Li T, Cai Y, Ma Q. Microbial diversity on the surface of historical monuments in lingyan temple, jinan, China. Microb Ecol (2022) 85:76–86. doi: 10.1007/s00248-021-01955-w
34. Wang Y, Huang W, Han Y, Huang X, Wang C, Ma K, et al. Microbial diversity of archaeological ruins of liangzhu city and its correlation with environmental factors. Int Biodeterioration Biodegradation (2022) 175. doi: 10.1016/j.ibiod.2022.105501
35. Zhao H, Zheng W, Zhang S, Gao W, Fan Y. Soil microbial community variation with time and soil depth in Eurasian steppe (Inner Mongolia, China). Ann Microbiol (2021) 71(1). doi: 10.1186/s13213-021-01633-9
36. Hao J, Chai YN, Lopes LD, Ordóñez RA, Wright EE, Archontoulis S, et al. The effects of soil depth on the structure of microbial communities in agricultural soils in Iowa (United states). Appl Environ Microbiol (2021) 87(4). doi: 10.1128/AEM.02673-20
37. Fierer N, Schimel JP, Holden PA. Variations in microbial community composition through two soil depth profiles. Soil Biol Biochem (2003) 35(1):167–76. doi: 10.1016/S0038-0717(02)00251-1
38. Naylor D, McClure R, Jansson J. Trends in microbial community composition and function by soil depth. Microorg (2022) 10(3). doi: 10.3390/microorganisms10030540
39. Romdhane S, Spor A, Banerjee S, Breuil MC, Bru D, Chabbi A, et al. Land-use intensification differentially affects bacterial, fungal and protist communities and decreases microbiome network complexity. Environ Microbiomes (2022) 17(1). doi: 10.1186/s40793-021-00396-9
40. Madegwa YM, Uchida Y. Land use and season drive changes in soil microbial communities and related functions in agricultural soils. Environ DNA. (2021) 3(6):1214–28. doi: 10.1002/edn3.244
41. Kuramae EE, Yergeau E, Wong LC, Pijl AS, Van Veen JA, Kowalchuk GA. Soil characteristics more strongly influence soil bacterial communities than land-use type. FEMS Microbiol Ecol (2012) 79(1):12–24. doi: 10.1111/j.1574-6941.2011.01192.x
42. Osburn ED, Aylward FO, Barrett JE. Historical land use has long-term effects on microbial community assembly processes in forest soils. ISME Commun (2021) 1(48). doi: 10.1038/s43705-021-00051-x
43. Hermans SM, Buckley HL, Case BS, Curran-Cournane F, Taylor M, Lear G. Using soil bacterial communities to predict physico-chemical variables and soil quality. Microbiome (2020) 8(1). doi: 10.1186/s40168-020-00858-1
44. Jangid K, Williams MA, Franzluebbers AJ, Schmidt TM, Coleman DC, Whitman WB. Land-use history has a stronger impact on soil microbial community composition than aboveground vegetation and soil properties. Soil Biol Biochem (2011) 43(10):2184–93. doi: 10.1016/j.soilbio.2011.06.022
45. Bull ID, Betancourt PP, Evershed RP. An organic geochemical investigation of the practice of manuring at a Minoan site on pseira island, Crete. GeoarchaeolInt J (2001) 16(2):223–42. doi: 10.1002/1520-6548(200102)16:2<223::AID-GEA1002>3.0.CO;2-7
46. Callahan BJ, Sankaran K, Fukuyama JA, McMurdie PJ, Holmes SP. Bioconductor workflow for microbiome data analysis: From raw reads to community analyses [version 1; referees: 3 approved]. F1000 Res (2016) 5. doi: 10.12688/F1000RESEARCH.8986.1
47. Callahan BJ, McMurdie PJ, Rosen MJ, Han AW, Johnson AJA, Holmes SP. DADA2: High-resolution sample inference from illumina amplicon data. Nat Methods (2016) 13(7):581–3. doi: 10.1038/nmeth.3869
48. Callahan B. he RDP and GreenGenes taxonomic training sets formatted for DADA2 [Data set]. (2016). [Online]. [Accessed 2022]. doi: 10.5281/zenodo.158955
49. Team RC. R: A language and environment for statistical computing. Vienna: R Foundation for Statistical Computing (2020).
51. Pohlert T. The pairwise multiple comparison of mean ranks package (PMCMR). (2015). [Online]. [Accessed 2022].
54. Wickham H, Averick M, Bryan J, Chang W, McGowan L, Francois R, et al. Welcome to the tidyverse. J Open Source Software (2019) 4(43):1686.
55. Wickham H. Reshaping data with the reshape package. J Stat Software (2007) 21(12). doi: 10.18637/jss.v021.i12
56. Wickham H, Hester J, Francois R. Readr: read rectangular text data (2018). Available at: https://cran.r-project.org/web/packages/ggplot2/citation.html.
57. Warnes GR, Bolker B, Bonebakker L, Gentleman R, Huber W, Liaw A, et al. Gplots:Various r programming tools for plotting data. R package 3.1.3 (2022). Available at: https://cran.r-project.org/package=gplots.
58. Segata N, Izard J, Waldron L, Gevers D, Miropolsky L, Garrett WS, et al. Metagenomic biomarker discovery and explanation. Genome Biol (2011) 12(6). doi: 10.1186/gb-2011-12-6-r60
59. Leeming R, Ball A, Ashbolt N, Nichols P. Using faecal sterols from humans and animals to distinguish faecal pollution in receiving waters. Water Res (1996) 30(12):2893–900. doi: 10.1016/S0043-1354(96)00011-5
60. Hakola H, Tarvainen V, Laurila T, Hiltunen V, Hellén H, Keronen P. Seasonal variation of VOC concentrations above a boreal coniferous forest. Atmospheric Environment (2003) 37(12):1623–34. doi: 10.1016/S1352-2310(03)00014-1
61. Hernandes C, Taleb-Contini SH, Bartolomeu ACD, Bertoni BW, Fran?a SC, Pereira AMS. Chemical composition and antifungal activity of the essential oils of schinus weinmannifolius collected in the spring and winter. Nat Pro Commun (2014) 9(9):1383–6. doi: 10.1177/1934578X1400900940
62. Böer SI, Hedtkamp SIC, Van Beusekom JEE, Fuhrman JA, Boetius A, Ramette A. Time- and sediment depth-related variations in bacterial diversity and community structure in subtidal sands. ISME J (2009) 3(7):780–91. doi: 10.1038/ismej.2009.29
63. He S, Guo L, Niu M, Miao F, Jiao S, Hu T, et al. Ecological diversity and co-occurrence patterns of bacterial community through soil profile in response to long-term switchgrass cultivation. Sci Rep (2017) 7(1). doi: 10.1038/s41598-017-03778-7
64. Brewer TE, Aronson EL, Arogyaswamy K, Billings SA, Botthoff JK, Campbell AN, et al. Ecological and genomic attributes of novel bacterial taxa that thrive in subsurface soil horizons. mBio (2019) 10(5). doi: 10.1128/mBio.01318-19
65. Yan H, Yang F, Gao J, Peng Z, Chen W. Subsoil microbial community responses to air exposure and legume growth depend on soil properties across different depths. Sci Rep (2019) 9(1). doi: 10.1038/s41598-019-55089-8
66. Prada-Salcedo LD, Prada-Salcedo JP, Heintz-Buschart A, Buscot F, Goldmann K. Effects of tree composition and soil depth on structure and functionality of belowground microbial communities in temperate European forests. Front Microbiol (2022) 13. doi: 10.3389/fmicb.2022.920618
67. Janssen PH. Identifying the dominant soil bacterial taxa in libraries of 16S rRNA and 16S rRNA genes. Appl Environ Microbiol (2006) 72(3):1719–28. doi: 10.1128/AEM.72.3.1719-1728.2006
68. Dunbar J, Barns SM, Ticknor LO, Kuske CR. Empirical and theoretical bacterial diversity in four Arizona soils. Appl Environ Microbiol (2002) 68(6):3035–45. doi: 10.1128/AEM.68.6.3035-3045.2002
69. Margesin R, Siles JA, Cajthaml T, Öhlinger B, Kistler E. Microbiology meets archaeology: Soil microbial communities reveal different human activities at archaic Monte iato (Sixth century BC). Microb Ecol (2017) 73(4):925–38. doi: 10.1007/s00248-016-0904-8
70. Orellana LH, Francis TB, Ferraro M, Hehemann JH, Fuchs BM, Amann RI. Verrucomicrobiota are specialist consumers of sulfated methyl pentoses during diatom blooms. ISME J (2022) 16(3):630–41. doi: 10.1038/s41396-021-01105-7
71. Dunfield PF, Yuryev A, Senin P, Smirnova AV, Stott MB, Hou S, et al. Methane oxidation by an extremely acidophilic bacterium of the phylum verrucomicrobia. Nature (2007) 450(7171):879–82. doi: 10.1038/nature06411
72. Umezawa K, Kojima H, Kato Y, Fukui M. Dissulfurispira thermophila gen. nov., sp. nov., a thermophilic chemolithoautotroph growing by sulfur disproportionation, and proposal of novel taxa in the phylum nitrospirota to reclassify the genus thermodesulfovibrio. Syst Appl Microbiol (2021) 44(2). doi: 10.1016/j.syapm.2021.126184
73. Franzluebbers AJ, Stuedemann JA, Wilkinson SR. Bermudagrass management in the southern piedmont USA: I. soil and surface residue carbon and sulfur. Soil Sci Soc Am J (2001) 65(3):834–41. doi: 10.2136/sssaj2001.653834x
74. Tian Y, Yu M, Xu F, Ouyang S, Xu X, Gao Q, et al. Uptake of amino acids and inorganic nitrogen by two dominant temperate grasses. Rhizosphere (2020) 14.
75. Henry HAL, Jefferies RL. Plant amino acid uptake, soluble n turnover and microbial n capture in soils of a grazed arctic salt marsh. J Ecol (2003) 91:627–36.
76. Morrissey J, Guerinot ML. Iron uptake and transport in plants: The good, the bad, and the ionome. Chem Rev (2009) 109:4553–67.
77. Davies AL, Harrault L, Milek K, McClymont EL, Dallimer M, Hamilton A, et al. A multiproxy approach to long-term herbivore grazing dynamics in peatlands based on pollen, coprophilous fungi and faecal biomarkers. Palaeogeogr Palaeoclimatol Palaeoecol (2022) 598.
78. Zhu D, Zhang P, Xie C, Zhang W, Sun J, Qian WJ, et al. Biodegradation of alkaline lignin by bacillus ligniniphilus L1. Biotechnol Biofuels (2017) 10.
79. McNamara CJ, Perry Iv TD, Bearce KA, Hernandez-Duque G, Mitchell R. Epilithic and endolithic bacterial communities in limestone from a Maya archaeological site. Microb Ecol (2006) 51(1):51–64. doi: 10.1007/s00248-005-0200-5
80. Zanardini E, May E, Inkpen R, Cappitelli F, Murrell JC, Purdy KJ. Diversity of archaeal and bacterial communities on exfoliated sandstone from portchester castle (UK). Int Biodeterioration Biodegradation (2016) 109:78–87. doi: 10.1016/j.ibiod.2015.12.021
81. Sun F, Bakr N, Dang T, Pham V, Weindorf DC, Jiang Z, et al. Enhanced soil profile visualization using portable X-ray fluorescence (PXRF) spectrometry. Geoderma (2020) 358. doi: 10.1016/j.geoderma.2019.113997
82. Lü Z, Lu Y. Methanocella conradii sp. nov., a thermophilic, obligate hydrogenotrophic methanogen, isolated from chinese rice field soil. PloS One (2012) 7.
83. Grillo-Puertas M, Villegas JM, Pankievicz VCS, Tadra-Sfeir MZ, Teles Mota FJ, Hebert EM, et al. Transcriptional responses of herbaspirillum seropedicae to environmental phosphate concentration. Front Microbiol (2021) 12.
84. Bird JT, Tague ED, Zinke L, Schmidt JM, Steen AD, Reese B, et al. Uncultured microbial phyla suggest mechanisms for multi-thousand-year subsistence in baltic sea sediments. mBio (2019) 10(2). doi: 10.1128/mBio.02376-18
85. Liang R, Lau M, Vishnivetskay T, Lloyd KG, Wang W, Wiggins J, et al. Predominance of anaerobic, spore-forming bacteria in metabolically active microbial communities from ancient Siberian permafrost. Appl Environ Microbiol (2019) 85(15). doi: 10.1128/AEM.00560-19
86. Aouizerat T, Gutman I, Paz Y, Maeir AM, Gadot Y, Gelman D, et al. Isolation and characterization of live yeast cells from ancient vessels as a tool in bio-archaeology. mBio (2019) 10(2). doi: 10.1128/mBio.00388-19
Keywords: Vindolanda, preservation, writing tablets, biomarkers, sterols, bacterial diversity
Citation: Taylor G, Williams R, Halldórsdóttir HH, Carter A, Birley A, Meyer A and Orr CH (2023) Archaeological soil from Roman occupational layers can be differentiated by microbial and chemical signatures. Front. Soil Sci. 3:1129040. doi: 10.3389/fsoil.2023.1129040
Received: 21 December 2022; Accepted: 30 January 2023;
Published: 14 February 2023.
Edited by:
Richa Raghuwanshi, Banaras Hindu University, IndiaReviewed by:
Sandhya Mishra, Key Laboratory of Tropical Forest Ecology (CAS), ChinaJay Prakash Verma, Banaras Hindu University, India
Amrita Saxena, Indian Institute of Science (IISc), India
Copyright © 2023 Taylor, Williams, Halldórsdóttir, Carter, Birley, Meyer and Orr. This is an open-access article distributed under the terms of the Creative Commons Attribution License (CC BY). The use, distribution or reproduction in other forums is permitted, provided the original author(s) and the copyright owner(s) are credited and that the original publication in this journal is cited, in accordance with accepted academic practice. No use, distribution or reproduction is permitted which does not comply with these terms.
*Correspondence: Caroline Hayley Orr, Yy5vcnJAdGVlcy5hYy51aw==