- 1Environmental Molecular Science Laboratory, Pacific Northwest National Laboratory, Richland, WA, United States
- 2Department of Biological Science, Bowling Green State University, Bowling Green, OH, United States
- 3School of Biological Sciences, Washington State University, Richland, WA, United States
- 4School of Biological Sciences, Washington State University, Vancouver, WA, United States
- 5School of the Environment, Washington State University, Vancouver, WA, United States
- 6Earth and Biological Sciences Directorate, Pacific Northwest National Laboratory, Richland, WA, United States
While significant progress has been made in understanding global carbon (C) cycling, the mechanisms regulating belowground C fluxes and storage are still uncertain. New molecular technologies have the power to elucidate these processes, yet we have no widespread standardized implementation of molecular techniques. To address this gap, we introduce the Molecular Observation Network (MONet), a decadal vision from the Environmental Molecular Sciences Laboratory (EMSL), to develop a national network for understanding the molecular composition, physical structure, and hydraulic and biological properties of soil and water. These data are essential for advancing the next generation of multiscale Earth systems models. In this paper, we discuss the 1000 Soils Pilot for MONet, including a description of standardized sampling materials and protocols and a use case to highlight the utility of molecular-level and microstructural measurements for assessing the impacts of wildfire on soil. While the 1000 Soils Pilot generated a plethora of data, we focus on assessments of soil organic matter (SOM) chemistry via Fourier-transform ion cyclotron resonance-mass spectrometry and microstructural properties via X-ray computed tomography to highlight the effects of recent fire history in forested ecosystems on belowground C cycling. We observed decreases in soil respiration, microbial biomass, and potential enzyme activity in soils with high frequency burns. Additionally, the nominal oxidation state of carbon in SOM increased with burn frequency in surface soils. This results in a quantifiable shift in the molecular signature of SOM and shows that wildfire may result in oxidation of SOM and structural changes to soil pore networks that persist into deeper soils.
Introduction
Soil organic matter (SOM) is a critical part of the global carbon (C) cycle. Belowground ecosystems contain more C than stored in terrestrial vegetation and the atmosphere combined (1–3), and SOM is the largest and most biologically active portion of soil C. SOM decomposition is regulated by a complex and interacting set of factors including soil structure, moisture distribution, temperature, pH, and nutrient status; collectively, these factors determine accessibility, bioavailability, and rate kinetics of SOM (4). Despite the importance of SOM in the global C cycle, the drivers of SOM decomposition from molecular to continental scales are not well understood.
Recent research suggests that standardized, spatially-resolved, and high-resolution data may be critical to reducing the uncertainty surrounding estimates of belowground C dynamics under future climate scenarios (5, 6). For example, pore-scale structural characterization could reveal spatial factors that inhibit SOM decomposition, and molecular chemistry may provide insight into SOM reactivity that cannot be observed from bulk measurements. As of yet, such information is lacking from soil databases (e.g., Web Soil Survey, International Soil Radiocarbon Database, and Soils Data Harmonization), and no widespread implementations of standardized high-resolution techniques are used to simultaneously investigate the myriad factors influencing SOM decomposition (7–10).
Consequently, soil C cycles are poorly represented in biogeochemical and Earth System Models (ESMs) that predict changes in the global climate (11). For example, most ESMs simulate changes in belowground C concentration within coarsely defined pools (i.e., agnostic of SOM composition) rather than representing chemical and microbial reactions within SOM pools (12–15). The depth of soil molecular and microphysical/chemical information presents a major limitation to improving models at regional and Continental United States (CONUS) scales, where impacts of climate change are manifested and can be understood.
Led by a team at the Environmental Molecular Science Laboratory (EMSL), a new decadal initiative to develop a CONUS-scale database of soil compositional, physical, and metagenomic measurements that are open and FAIR (Findable, Accessible, Interoperable and Reusable), termed the Molecular Observation Network (MONet) program (16, 17). The goal of MONet is to facilitate molecular data syntheses and modelling activities that can reduce uncertainty in soil C simulation at regional and CONUS scales. In this article, we describe the 1000 Soils Pilot for MONet, which focuses on understanding the drivers and fluxes of soil C cycles through standardized molecular measurements. It represents our initial goal to collect soil cores from 1000 locations.
To highlight the power of high-resolution measurements for understanding soil structure, molecular SOM composition, and SOM decomposition, we also present a use case from the 1000 Soils Pilot. As wildfires are becoming increasingly prevalent worldwide (18, 19) and have lasting impacts on ecosystem structure and function (18, 20–26), we focus our use case on a set of three locations with varied burned histories. We show that wildfire may result in oxidation of SOM and in structural changes to soil pore networks that persist into deeper soils, which are not investigated by typical sampling schema. These are important considerations in the management of forest ecosystem restoration post-wildfire and present an interesting avenue for more robust investigations as the MONet database expands.
Approach
Through partnerships with individual researchers and ecological networks (Figure 1A), the 1000 Soils Pilot facilitated the collection of 76 sets of soil cores using a standardized sampling kit and field protocols. Collaborators collected two 30-cm (3-inch dia.) replicate intact cores using a slide hammer soil corer (AMS, Inc., USA), as well as four 10-cm (2-inch dia.) soil cores using a wooden block and mallet. Other field-based measurements included soil temperature, moisture, and electrical conductivity, measured using a Teros 12 Probe and ZSC Bluetooth interface (Meter Group, Inc., USA). Soil infiltration rate was measured using the single-ring infiltrometer method (27, 28). A step-by-step sampling protocol is available in the SI and at protocols.io (DOI: 10.17504/protocols.io.5jyl8jr49g2w/v2). After sample collection, all data was generated by EMSL or EMSL-contracted vendors. All data from the 1000 Soils Pilot are available via Zenodo (https://zenodo.org/communities/emsl-monet; 29).
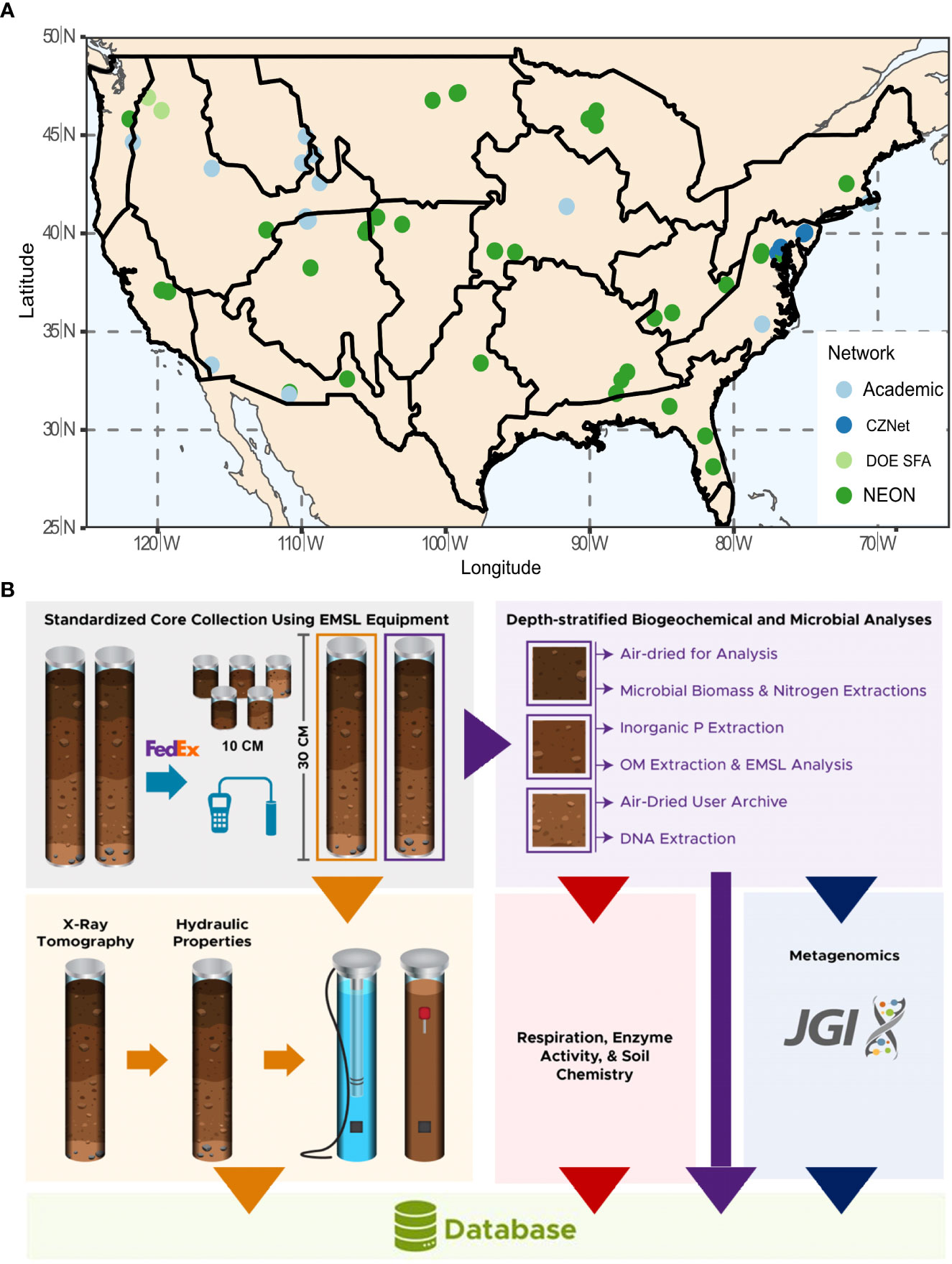
Figure 1 (A) Sites included in the 1000 Soils Pilot overlain on National Ecological Observatory Network (NEON) eco-domains. The color of the symbol indicates site affiliation as Academic (light blue), Critical Zone Network (CZNet; dark blue), Department of Energy Science Focus Area (DOE SFA; light green), and NEON (dark green). (B) Soil core analysis workflow.
Data generation overview
Collaborators shipped all cores to EMSL on ice within 24 hr of core collection for immediate processing. One 30-cm core was dedicated to a biotic workflow for time sensitive measurements, and the other 30-cm core was dedicated to an abiotic workflow for structural and hydraulic characterization (Figure 1B). To account for surface heterogeneity, three of the 10 cm cores were homogenized with the top 10 cm of the larger biotic core. A full list of data types generated by the 1000 Soils Pilot is available in SI Table 1.
Immediately on arrival (<48 hr), we processed the biotic core by separating it into 10 cm depth intervals, which were then sieved at 4 mm. The middle section was archived, and all analyses were conducted on homogenized top (0-10 cm) and bottom (deepest 10 cm) sections as described below. We measured microbial biomass C and N via chloroform fumigation (30–32). Aliquots of K2SO4 extractions from the initial (background) steps of microbial biomass measurements were archived at - 20° C for later analysis of NO3- via Cd-reduction method and NH4+ via spectroscopic determination (33, 34). Inorganic P was extracted from each soil section via Bray or Olsen extraction (depending on pH) and subsequent analysis via methods outlined in (35, 36). We collected sub-samples of soil for DNA extraction and subsequent metagenomic sequencing on the Illumina NovaSeq platform (stored at -80° C). We measured soil pH using a 1:1 soil to water ratio using a calibrated pH probe. Gravimetric water content was determined via oven drying at 60° C. Remaining soil from each section was air dried prior to the analyses below. β-glucosidase potential activity and respiration rates were measured using colorimetric assays and the CO2 burst method. Ion concentrations from 1:10 ammonium acetate extraction (K, Ca, Mg, Na) and 1:2 soil to diethylenetriaminepentaacetic acid (DPTA) extraction solution (Zn, Mg, Cu, Fe, B, SO42--S) extraction were measured with Inductively coupled plasma mass spectrometry (ICP-MS), while SOM concentration was determined by total organic carbon-total nitrogen (TOC-TN) analysis and composition from liquid chromatography mass spectrometry (LC-MS), and Fourier transform ion cyclotron resonance mass spectrometry (FTICR-MS) analysis. Details on SOM characterization are provided in the SI.
The abiotic core was used to determine soil structure and hydraulic properties. We used X-ray computed tomography (XCT) to measure soil pore size, connectivity, distribution, and volume, as detailed below. In addition, soil hydraulic properties were measured using a series of instruments by The Meter Group (KSat, Hyprop, WP4C, Meter Group, Inc., USA).
Detailed methods for data collection and analysis for XCT and FTICR-MS can be found in the SI.
Case study: impacts of wildfire regimes on soil; Warm Springs, Oregon
Importance of fire on ecosystems
As the frequency of wildfires continue to increase due to anthropogenic climate change and land management practices, it is critical to understand how fire disturbances affect belowground biogeochemistry (18, 37). Wildfires alter belowground processes that generally result in decreased structural stability, microbial respiration and biomass, and SOM concentration (20–22, 24, 25, 38, 39). For instance, Certini et al. (40) reported wildfire significantly decreased total soil C, which was associated with increased lignin degradation. Others have reported the selective loss of SOM oxygen containing functional groups and/or with less condensed structures in response to wildfire (38). Changes in SOM concentration and composition are coincident with structural and hydrologic changes including decreased water infiltration and increased soil erosion (23). Because belowground C storage and bioavailability are dramatically altered by wildfire, it is important to understand the nature of wildfire effects on SOM chemistry and accessibility. Below, we describe a case study to demonstrate the utility of high-resolution data for addressing this need.
Dataset description
Our primary intent in this paper is to stimulate discussion and interest in large-scale collaborative efforts by introducing MONet. To facilitate this discussion, we present initial results from three of the first cores in the 1000 Soils Pilot, collected in November 2021 in partnership with the Confederate Tribes of Warm Springs in Warm Springs, OR, USA. This region typically experiences cold, snowy winters followed by warm dry summers with a mean annual precipitation of 1778 mm and a mean annual precipitation of 8.3°C. Soil samples were collected from plots dominated by warm and moist type grand fir (A. grandis) and snowbrush (C. velutinus). The unburned (UB plots) had forb and shrub ground cover, mature living conifers, and surface litter present (>1mm) and had no recent burn history in the last 20 years. The moderate burn frequency (MB) plots contained shrubs and dead-standing trees but very little surface litter (<1 mm) and had moderate severity burns in 2014 and 2020. The high frequency burn (HB) plots consisted of a low shrub ground cover, no live or dead standing trees, and very little surface litter (<1 mm) and had moderate severity burns in 2003, 2014 and 2020.
This subset of data is intended as a proof of concept for the utility of high-resolution data in understanding impacts of fire burn histories on soil physio-chemical properties and microbial functions. Though the dataset is small and does not allow for robust statistics (n = 3), it provides a wealth of information that is unfeasible with traditional biogeochemical approaches. With these differences in mind, we compared FTICR-MS and XCT across burn histories and soil depth to show differences in molecular SOM composition and soil structure.
Soil biogeochemistry and microbial activity
We first compared soil biogeochemical properties including, β-glucosidase potential activity (BG), microbial biomass C (MBC) and N (MBN), and respiration at 24 and 96 hr for differences among soil sections and burn histories (Figures 2A, B). Elevated microbial biomass concentrations, respiration rates, and potential enzyme activities in the unburned site are consistent with increased biological availability when compared to the burned sites (39). We found the highest respiration rate at UB followed by the MB, with the lowest respiration at HB (Figure 2B). This is consistent with other literature, showing the sterilizing effects of high frequency fires on microbial respiration (26, 41). Across the first 24 hours after re-wet, the rate of CO2 produced was greater in the top 10 cm vs. the lowest 10 cm across all cores. However, after 96 hours the rate of CO2 production in soils from the bottom 10 cm was greater than the top 10 cm for all sites (39). Similarly, β-glucosidase potential activity and microbial biomass C and N were highest at UB (SI Figure 1A and Figure 2B respectively). Lower microbial activity at the burned sites may be related to chemical recalcitrance or physical protection of SOM related to the fires (26, 41). For all the sites, respiration increased between 24 and 96 hours in the bottom 10 cm, possibly reflecting less bioavailable SOM in deeper soils than in surface soils (42). Corresponding changes in soil chemistry are shown in SI Figure 2.
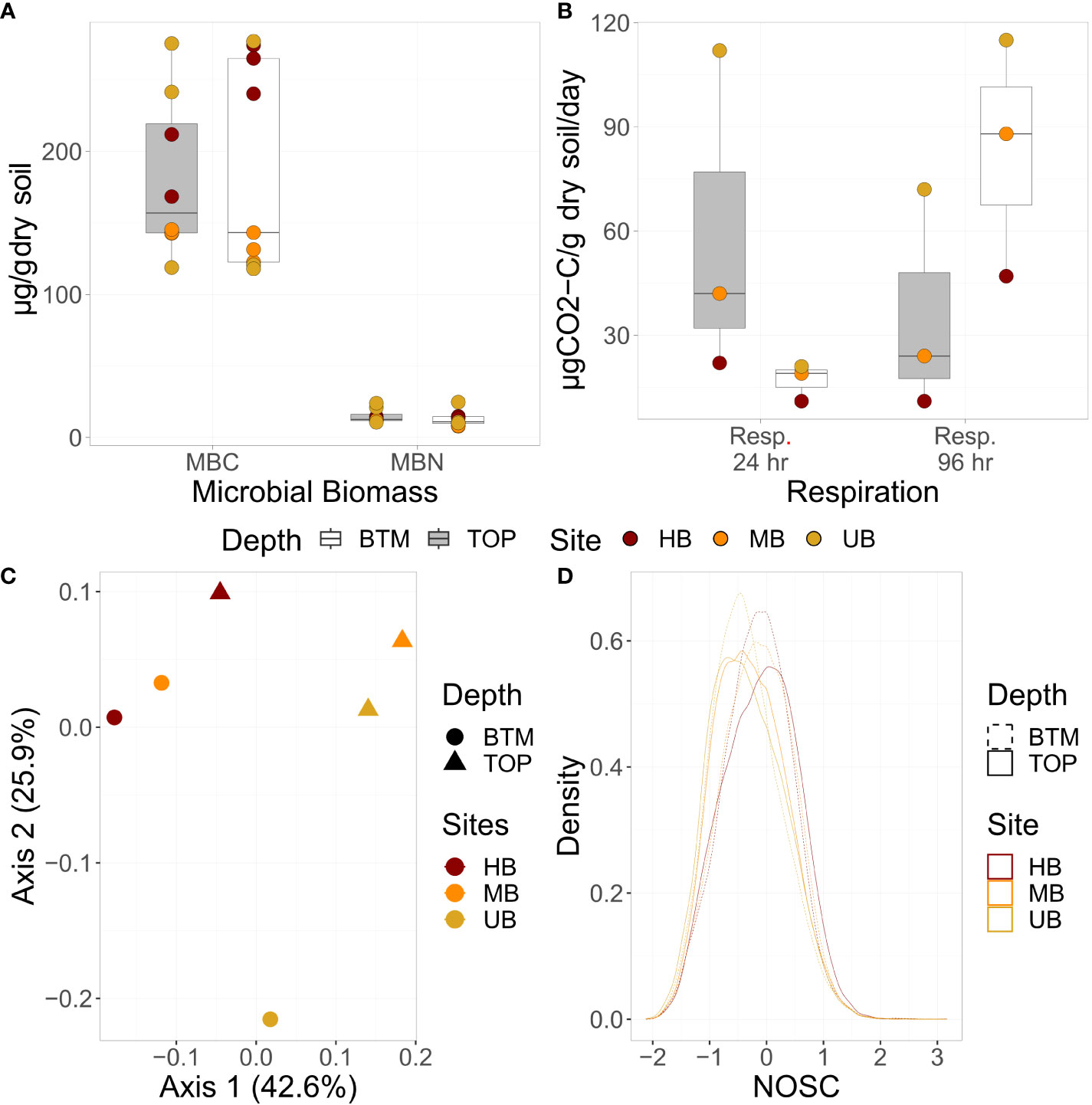
Figure 2 (A) Microbial biomass C (MBC) and N (MBN) concentrations, (B) respiration (24 and 96 hr; µg CO2-C/g dry soil/day), (C) SOM composition, and (D) NOSC of SOM profiles. (A, B) are boxplots where the median is represented by a line, the edges of the boxes reflect 25th and 75th percentile, and the end of the whiskers reflect the minimum and maximum. (C) shows a principal component analysis of SOM composition, and (D) displays a density curve of NOSC values for each sample. For all plots, UB, MB, and HB are denoted in yellow, orange, and red respectively. In (A, B) data from top and bottom sections are denoted by grey and white boxes respectively. In (C), section is denoted by shape, and in (D), section is denoted by dashed vs. solid lines.
X-ray computed tomography
To examine pore space heterogeneity across soil sections and burn histories, we used XCT to interrogate the top and bottom 10 cm of each soil core. We compared total porosity, pore connectivity, and mean pore volume between the top and bottom sections of cores from UB, MB, and HB. Wildfire can impact soil structure at least to 10 cm depth depending on its duration, frequency, and intensity (22). For UB and HB, we observed higher porosity and pore connectivity in the top sections when compared to the bottom sections. This is likely related to the higher abundance of root systems in the top 10 cm. However, there was minimal difference between total porosity or connectivity between the top and bottom section of the MB site, possibly due to a large root section resulting in a larger mean pore size (SI Figure 5 and SI Table 2). Across all cores, total porosity was more variable in the top than bottom section (8.82-20.08% vs 6.52-8.49%; SI Table 2). Pore connectivity was highest in the top section at UB (96.41%) and lowest in the bottom section at UB (70.12%); potentially indicating a homogenizing effect of wildfire on pore network structure at MB and HB. Overall, these data suggest that wildfire may decrease pore connectivity and total porosity in surface soils, likely contributing to increased hydrophobicity and soil erosion, while the deeper soils are more insulated from wildfire effects.
Soil organic matter composition
Soil organic matter is derived from chemical, physical, and biological processes that decompose plant and animal residues into microbial metabolites and other byproducts (1, 5, 43–45). This generates a heterogenous mixture of compounds in SOM pools. We used FTICR-MS to distinguish differences in SOM chemistry along depth profiles and across the three wildfire histories. There was a clear separation in water-extractable SOM composition between the top and bottom sections of each core as well as across burn regimes, as indicated by principal component analysis (Figure 2C). Additionally, the bottom sections exhibited more similar SOM pools (i.e., tighter clustering) than the top sections, possibly signifying stronger impacts of wildfire on surficial soils.
Since fire results in the oxidation of SOM to carbon dioxide (CO2), we hypothesized that the extent of SOM oxidation, as indicated by the nominal oxidation state of carbon (NOSC), would be related to burn regime. In the top section, the average NOSC of SOM increased with stronger burn regimes (-0.34, -0.29, and -0.11 respectively for UB, MB, and HB), indicating more oxidization, while there was a binary effect of burning on NOSC in the bottom section (Figure 2D). MB and HB had similar mean NOSC that was higher than UB (-0.16 vs -0.35). The presence of more oxidized SOM in burned soils, is likely related to the incomplete oxidation of SOM and the addition of charred materials from wildfire (46–48). While limited to three sites, changes in NOSC could be an indicator of previous fire exposure, with the NOSC becoming more oxidized with increased high frequency fire exposure that persists even into deeper soil horizons.
Inferred biochemical transformations and microbial metabolism of SOM
We also analyzed FTICR-MS spectra to understand potential biochemical transformations and microbial pathways involved in SOM decomposition. Commonly inferred biochemical transformations such as the addition of methyl chain (-CH2) groups were abundant at all sites and depths. We also note that one of the most abundant transformations observed was the conversion of carboxyl groups to CO2 (greater than 1400 occurrences at each site and depth). The transformation of carboxyl groups to CO2 likely results in combustion byproduct associated with incomplete oxidation of SOM during fire exposure. Within each core, the oxidation and/or addition of CO2 accounted for a greater proportion of transformations at depth when compared to the top section at the same site. This is potentially related to the translocation of water-soluble oxidized OM to deeper soil sections (49).
Additionally, we mapped compounds detected by FTICR-MS to microbial reference pathways in KEGG to infer mechanisms of SOM decomposition. Across all samples, nearly 50% of all mapped metabolites were detected in a set of eight metabolic pathways. These included ‘biosynthesis of secondary metabolites’ (map01110, 11.7%), ‘metabolic pathways’ (map01100, 8.4%), ‘biosynthesis of antibiotics’ (map01130, 7.5%), and ‘microbial metabolism in diverse environments’ (map01120, 4.9%) among other pathways (SI Table 4). Notably, the degradation of aromatic compounds (map012220) and the polycyclic aromatic hydrocarbons (PAH) degradation (map00624) were also among pathways with the highest number of mapped metabolites (16th and 19th most metabolites). The degradation of aromatic compounds was the most common pathway in the core with high frequency burns (HB). The degradation of PAHs was most common in the MB site; 1.61% of metabolites mapped to metabolic pathways in the moderate frequency burned core were associated with the degradation of PAHs in comparison to <1% of mapped metabolites in the unburned core.
Case study conclusions
Overall, our results were consistent with literature describing wildfire impacts on soils and provided greater understanding of molecular changes in soil organic matter composition and structure than is possible with typical measurements. In soils with recent fire history, we observed decreases in soil respiration, microbial biomass, and potential enzyme activity (20, 21, 24, 26, 39, 50). This was associated with shifts in SOM composition, with higher rates of oxidation apparent in surface soils. Deeper soils also showed increased NOSC as a function of previous fire history. By inferring biogeochemical transformations from SOM pool composition, we also show the possible conversion of carboxyl groups to CO2 that is likely related to the oxidizing effects of wildfire. Using metabolic pathway mapping, we observed that the degradation of aromatic compounds and polycyclic aromatic hydrocarbons may also be associated with wildfire. Finally, wildfire appeared to induce structural changes including decreased pore connectivity and total porosity in surface soils, possibly contributing to hydrophobicity and decreased soil infiltration which can result in greater soil erosion (20, 23). The combined molecular and microstructural changes observed in this use case provide important insight and considerations in the management and modelling of forest ecosystems post-wildfire. As the MONet database continues to expand, we hope to use this information to model wildfire impacts on belowground C cycling at the regional to CONUS scale.
Towards a CONUS-scale high resolution molecular SOM database
Earth system models currently rely on soil attributes such as soil texture, moisture, and C concentration to parameterize C fluxes. This highly simplified approach results in high levels of uncertainty at the pore to core scale. Molecular information has the potential to reduce this uncertainty through rapidly improving multiscale models (6, 12, 51–57).
To achieve these goals, MONet is developing a database of high-resolution soil organic matter, metagenomic, and structural information from soil cores across the CONUS. We collaborate with existing databases and networks, as well as with individual researchers, using standardized sample collection and high throughput processing pipelines, metadata documentation, and raw and processed open data publication. The focus of our data generation is on variables that are key to the parameterization of hierarchical multiscale models beginning with microbially explicit models and culminating in ESMs.
In this article, we present the 1000 Soils Pilot for MONet including a use case to highlight the utility of high-resolution measurements for understanding the impacts of wildfires on soils. While the statistical analysis is limited by the small sample number (n=3), we observed trends in soil biogeochemical values, soil structure, and SOM composition related to fire history and soil section that are consistent with existing literature. We are currently accepting requests for collaboration, and we anticipate that all data from the first set of 76 cores will be available in 2023. With the support and collaboration of the scientific community, we aim to facilitate the next generation of fundamental knowledge and model representations of soil C cycling, which in turn constrain uncertainties in the global climate.
Funding
Collection of soils from the Warm Springs Reservation was funded by a Washington State University, Vancouver Mini-Grant to SP and KM, and a Washington State University College of Arts and Sciences Seed Grant to SP, KM, TC, and EG. In addition, we would like to thank the tribal leaders from the Confederate Tribes of Warm Springs. This research was performed on a project award (https://dx.doi.org/10.46936/intm.proj.2021.60141/60000423) from the Environmental Molecular Sciences Laboratory, a DOE Office of Science User Facility sponsored by the Biological and Environmental Research program under Contract No. DE-AC05-76RL01830. A portion of this work was also supported by the U.S. Department of Energy (DOE), Office of Science (SC), Biological and Environmental Research (BER), Research Development and Partnership Pilot (RDPP), under Award Number(s) DE-SC0023150. This report was prepared as an account of work sponsored by an agency of the United States Government. Neither the United States Government nor any agency thereof, nor any of their employees, makes any warranty, express or implied, or assumes any legal liability or responsibility for the accuracy, completeness, or usefulness of any information, apparatus, product, or process disclosed, or represents that its use would not infringe privately owned rights. Reference herein to any specific commercial product, process, or service by trade name, trademark, manufacturer, or otherwise does not necessarily constitute or imply its endorsement, recommendation, or favoring by the United States Government or any agency thereof.
Conflict of interest
The authors declare that the research was conducted in the absence of any commercial or financial relationships that could be construed as a potential conflict of interest.
Publisher’s note
All claims expressed in this article are solely those of the authors and do not necessarily represent those of their affiliated organizations, or those of the publisher, the editors and the reviewers. Any product that may be evaluated in this article, or claim that may be made by its manufacturer, is not guaranteed or endorsed by the publisher.
Author disclaimer
The views and opinions of authors expressed herein do not necessarily state or reflect those of the United States Government or any agency thereof.
Supplementary material
The Supplementary Material for this article can be found online at: https://www.frontiersin.org/articles/10.3389/fsoil.2023.1120425/full#supplementary-material
References
1. Jobbágy EG, Jackson RB. The vertical distribution of soil organic carbon and its relation to climate and vegetation. Ecol Appl (2000) 10(2):423–36. doi: 10.1890/1051-0761(2000)010[0423:TVDOSO]2.0.CO;2
2. Crowther TW, Todd-Brown KEO, Rowe CW, Wieder WR, Carey JC, Machmuller MB, et al. Quantifying global soil carbon losses in response to warming. Nature (2016) 540(7631):104–8. doi: 10.1038/nature20150
3. Crowther TW, Riggs C, Lind EM, Borer ET, Seabloom EW, Hobbie SE, et al. Sensitivity of global soil carbon stocks to combined nutrient enrichment. Ecol Lett (2019) 22(6):936–45. doi: 10.1111/ele.13258
4. Graham EB, Hofmockel KS. Ecological stoichiometry as a foundation for omics-enabled biogeochemical models of soil organic matter decomposition. Biogeochemistry (2022) 157(1):31–50. doi: 10.1007/s10533-021-00851-2
5. Lehmann J, Kleber M. The contentious nature of soil organic matter. Nature (2015) 528:60–8. doi: 10.1038/nature16069
6. Viscarra Rossel RA, Lee J, Behrens T, Luo Z, Baldock J, Richards A. Continental-scale soil carbon composition and vulnerability modulated by regional environmental controls. Nat Geosci (2019) 12(7):547–52. doi: 10.1038/s41561-019-0373-z
7. Bond-Lamberty B, Thomson A. Temperature-associated increases in the global soil respiration record. Nature (2010) 464(7288):579–82. doi: 10.1038/nature08930
8. NRCS, U.N.U, United States Department of Agriculture Natural Resource Conservation Service. Web soil survey. map generated over river highlands state park in Cromwell, Connecticut (2019). Available at: https://websoilsurvey.sc.egov.usda.gov/App/HomePage.
9. Lawrence CR, Beem-Miller J, Hoyt AM, Monroe G, Sierra CA, Stoner S, et al. An open-source database for the synthesis of soil radiocarbon data: international soil radiocarbon database (ISRaD) version 1.0. Earth System Sci Data (2020) 12(1):61–76. doi: 10.5194/essd-12-61-2020
10. Wieder WR, Pierson D, Earl S, Lajtha K, Baer SG, Ballantyne F, et al. SoDaH: the SOils DAta harmonization database, an open-source synthesis of soil data from research networks, version 1.0. Earth System Sci Data (2021) 13(5):1843–54. doi: 10.5194/essd-13-1843-2021
11. Bradford MA, Wieder WR, Bonan GB, Fierer N, Raymond PA, Crowther TW. Managing uncertainty in soil carbon feedbacks to climate change. Nat Climate Change (2016) 6(8):751–8. doi: 10.1038/nclimate3071
12. Rasmussen C, Heckman K, Wieder WR, Keiluweit M, Lawrence CR, Berhe AA, et al. Beyond clay: towards an improved set of variables for predicting soil organic matter content. Biogeochemistry (2018) 137(3):297–306. doi: 10.1007/s10533-018-0424-3
13. Malhotra A, Todd-Brown K, Nave LE, Batjes NH, Holmquist JR, Hoyt AM, et al. The landscape of soil carbon data: Emerging questions, synergies and databases. Prog Phys Geography: Earth Environ (2019) 43(5):707–19. doi: 10.1177/0309133319873309
14. Bond-Lamberty B, Christianson DS, Malhotra A, Pennington SC, Sihi D, Aghakouchak A, et al. COSORE: A community database for continuous soil respiration and other soil-atmosphere greenhouse gas flux data. Global Change Biol (2020) 26(12):7268–83. doi: 10.1111/gcb.15353
15. Waring BG, Sulman BN, Reed S, Smith AP, Averill C, Creamer CA, et al. From pools to flow: The PROMISE framework for new insights on soil carbon cycling in a changing world. Global Change Biol (2020) 26(12):6631–43. doi: 10.1111/gcb.15365
16. Wilkinson MD, Dumontier M, Aalbersberg IJ, Appleton G, Axton M, Baak A, et al. The FAIR guiding principles for scientific data management and stewardship. Sci Data (2016) 3(1):160018. doi: 10.1038/sdata.2016.18
17. Todd-Brown KEO, Abramoff RZ, Beem-Miller J, Blair HK, Earl S, Frederick KJ, et al. Reviews and syntheses: The promise of big diverse soil data, moving current practices towards future potential. Biogeosciences (2022) 19(14):3505–22. doi: 10.5194/bg-19-3505-2022
18. Abatzoglou JT, Williams AP. Impact of anthropogenic climate change on wildfire across western US forests. Proc Natl Acad Sci (2016) 113(42):11770–5. doi: 10.1073/pnas.1607171113
19. Aponte C, De Groot WJ, Wotton BM. Forest fires and climate change: causes, consequences and management options. Int J Wildland Fire (2016) 25(8). doi: 10.1071/WFv25n8_FO
20. Certini G. Effects of fire on properties of forest soils: a review. Oecologia (2005) 143(1):1–10. doi: 10.1007/s00442-004-1788-8
21. Neary DG, Ryan KC, DeBano LF. Wildland fire in ecosystems: effects of fire on soils and water. U.S. Department of Agriculture, Forest Service, Rocky Mountain Research Station (2005).
22. Doerr SH, Shakesby RA, Blake WH, Chafer CJ, Humphreys GS, Wallbrink PJ. Effects of differing wildfire severities on soil wettability and implications for hydrological response. J Hydrology (2006) 319(1-4):295–311. doi: 10.1016/j.jhydrol.2005.06.038
23. Verma S, Jayakumar S. Impact of forest fire on physical, chemical and biological properties of soil: A review. Proc Int Acad Ecol Environ Sci (2012) 2(3):168.
24. Wang Q, Zhong M, Wang S. A meta-analysis on the response of microbial biomass, dissolved organic matter, respiration, and n mineralization in mineral soil to fire in forest ecosystems. For Ecol Manage (2012) 271:91–7. doi: 10.1016/j.foreco.2012.02.006
25. Matosziuk LM, Gallo A, Hatten J, Bladon KD, Ruud D, Bowman M, et al. Short-term effects of recent fire on the production and translocation of pyrogenic carbon in great smoky mountains national park. Front Forests Global Change (2020) 3. doi: 10.3389/ffgc.2020.00006
26. Lombao A, Barreiro A, Fontúrbel MT, Martín A, Carballas T, Díaz-Raviña M. Effect of repeated soil heating at different temperatures on microbial activity in two burned soils. Sci Total Environ (2021) 799:149440. doi: 10.1016/j.scitotenv.2021.149440
27. Klute A, Dirksen C. Hydraulic conductivity and diffusivity: Laboratory methods. In Methods of soil analysis (1986) (Madison, WI, USA; Soil Science Society of America, Inc.). pp. 687–734. doi: 10.2136/sssabookser5.1.2ed.c28
28. Dane JH, Hopmans JW Water retention and storage. In Methods of soil analysis. Part 4. SSSA Book Ser. 5. Eds Dane JH, Topp GC (Madison, WI, USA: Soil Science Society of America, Inc.), 671–796. doi: 10.2136/sssabookser5.4
29. Bowman MM, Heath AE, Varga T, Battu AK, Kew W, Chu RK, et al. 1000 Soils Pilot Dataset Environmental Molecular Science Laboratory. (2022). doi: 10.5281/zenodo.7406532
30. Brookes P, Landman A, Pruden G, Jenkinson D. Chloroform fumigation and the release of soil nitrogen: a rapid direct extraction method to measure microbial biomass nitrogen in soil. Soil Biol Biochem (1985) 17(6):837–42. doi: 10.1016/0038-0717(85)90144-0
31. Witt C, Gaunt JL, Galicia CC, Ottow JC, Neue H-U. A rapid chloroform-fumigation extraction method for measuring soil microbial biomass carbon and nitrogen in flooded rice soils. Biol Fertility Soils (2000) 30(5):510–9. doi: 10.1007/s003740050030
32. Zhao Q, Thompson AM, Callister SJ, Tfaily MM, Bell SL, Hobbie SE, et al. Dynamics of organic matter molecular composition under aerobic decomposition and their response to the nitrogen addition in grassland soils. Sci Total Environ (2022) 806:150514. doi: 10.1016/j.scitotenv.2021.150514
33. Bremner J.T. Inorganic forms of nitrogen. In Methods of Soil Analysis (1965) 9:1179–1237. doi: 10.2134/agronmonogr9.2.c33
34. Dahnke W, Johnson GV. Testing soils for available nitrogen. Soil testing Plant Anal (1990) 3:127–39. doi: 10.2136/sssabookser3.3ed.c6
35. Bray RH, Kurtz LT. Determination of total, organic, and available forms of phosphorus in soils. Soil Sci (1945) 59(1):39–46. doi: 10.1097/00010694-194501000-00006
36. Olsen S, Sommers L, Page A Phosphorus. In Page AL Methods of Soil Analysis Part 2 Chemical and Microbiological Properties (Madison: American Society of Agronomy, Soil Science Society of America) (1982), 403–30.
37. Huffman MS, Madritch MD. Soil microbial response following wildfires in thermic oak-pine forests. Biol Fertility Soils (2018) 54(8):985–97. doi: 10.1007/s00374-018-1322-5
38. González-Pérez JA, González-Vila FJ, Almendros G, Knicker H. The effect of fire on soil organic matter–a review. Environ Int (2004) 30(6):855–70. doi: 10.1016/j.envint.2004.02.003
39. Fairbanks D, Shepard C, Murphy M, Rasmussen C, Chorover J, Rich V, et al. Depth and topographic controls on microbial activity in a recently burned sub-alpine catchment. Soil Biol Biochem (2020) 148:107844. doi: 10.1016/j.soilbio.2020.107844
40. Certini G, Nocentini C, Knicker H, Arfaioli P, Rumpel C. Wildfire effects on soil organic matter quantity and quality in two fire-prone Mediterranean pine forests. Geoderma (2011) 167-168:148–55. doi: 10.1016/j.geoderma.2011.09.005
41. Ajwa HA, Dell CJ, Rice CW. Changes in enzyme activities and microbial biomass of tallgrass prairie soil as related to burning and nitrogen fertilization. Soil Biol Biochem (1999) 31(5):769–77. doi: 10.1016/S0038-0717(98)00177-1
42. Fang C, Moncrieff JB. The variation of soil microbial respiration with depth in relation to soil carbon composition. Plant Soil (2005) 268(1):243–53. doi: 10.1007/s11104-004-0278-4
43. Tarnocai C, Canadell JG, Schuur EAG, Kuhry P, Mazhitova G, Zimov S. Soil organic carbon pools in the northern circumpolar permafrost region. Global Biogeochemical Cycles (2009) 23(2). doi: 10.1029/2008GB003327
44. Schmidt MWI, Torn MS, Abiven S, Dittmar T, Guggenberger G, Janssens IA, et al. Persistence of soil organic matter as an ecosystem property. Nature (2011) 478:49–56. doi: 10.1038/nature10386
45. Köchy M, Hiederer R, Freibauer A. Global distribution of soil organic carbon – part 1: Masses and frequency distributions of SOC stocks for the tropics, permafrost regions, wetlands, and the world. Soil (2015) 1(1):351–65. doi: 10.5194/soil-1-367-2015
46. Schmidt MWI, Noack AG. Black carbon in soils and sediments: analysis, distribution, implications, and current challenges. Global Biogeochemical cycles (2000) 14(3):777–93. doi: 10.1029/1999GB001208
47. Watson JG, Chow JC, Chen LWA. Summary of organic and elemental Carbon/Black carbon analysis methods and intercomparisons. Aerosol Air Qual Res (2005) 5(1):65–102. doi: 10.4209/aaqr.2005.06.0006
48. Velasco-Molina M, Berns AE, Macías F, Knicker H. Biochemically altered charcoal residues as an important source of soil organic matter in subsoils of fire-affected subtropical regions. Geoderma (2016) 262:62–70. doi: 10.1016/j.geoderma.2015.08.016
49. Hockaday WC, Grannas AM, Kim S, Hatcher PG. The transformation and mobility of charcoal in a fire-impacted watershed. Geochimica Cosmochimica Acta (2007) 71(14):3432–45. doi: 10.1016/j.gca.2007.02.023
50. Guénon R, Vennetier M, Dupuy N, Roussos S, Pailler A, Gros R. Trends in recovery of Mediterranean soil chemical properties and microbial activities after infrequent and frequent wildfires. Land Degradation Dev (2013) 24(2):115–28. doi: 10.1002/ldr.1109
51. Blankinship JC, Berhe AA, Crow SE, Druhan JL, Heckman KA, Keiluweit M, et al. Improving understanding of soil organic matter dynamics by triangulating theories, measurements, and models. Biogeochemistry (2018) 140(1):1–13. doi: 10.1007/s10533-018-0478-2
52. Kramer MG, Chadwick OA. Climate-driven thresholds in reactive mineral retention of soil carbon at the global scale. Nat Climate Change (2018) 8(12):1104–8. doi: 10.1038/s41558-018-0341-4
53. Quesada CA, Paz C, Oblitas Mendoza E, Phillips OL, Saiz G, Lloyd J. Variations in soil chemical and physical properties explain basin-wide Amazon forest soil carbon concentrations. Soil (2020) 6(1):53–88. doi: 10.5194/soil-6-53-2020
54. Feng S, Lauvaux T, Williams CA, Davis KJ, Zhou Y, Baker I, et al. Joint CO2 mole fraction and flux analysis confirms missing processes in CASA terrestrial carbon uptake over north America. Global Biogeochemical Cycles (2021) 35(7). doi: 10.1029/2020GB006914
55. Nave LE, Bowman M, Gallo A, Hatten JA, Heckman KA, Matosziuk L, et al. Patterns and predictors of soil organic carbon storage across a continental-scale network. Biogeochemistry (2021) 156:75–96. doi: 10.1007/s10533-020-00745-9
56. Von Fromm SF, Hoyt AM, Lange M, Acquah GE, Aynekulu E, Berhe AA, et al. Continental-scale controls on soil organic carbon across sub-Saharan Africa. Soil (2021) 7(1):305–32. doi: 10.5194/soil-7-305-2021
Keywords: soil organic matter, X-ray computed tomography (XCT), Fourier transform ion cyclotron resonance mass spectrometry, FTICR-MS, open science, molecular observation network (MONet)
Citation: Bowman MM, Heath AE, Varga T, Battu AK, Chu RK, Toyoda J, Cheeke TE, Porter SS, Moffett KB, LeTendre B, Qafoku O, Bargar JR, Mans DM, Hess NJ and Graham EB (2023) One thousand soils for molecular understanding of belowground carbon cycling. Front. Soil Sci. 3:1120425. doi: 10.3389/fsoil.2023.1120425
Received: 09 December 2022; Accepted: 14 March 2023;
Published: 14 April 2023.
Edited by:
Tongbin Zhu, Chinese Academy of Geological Sciences, ChinaReviewed by:
Mario Guevara, University of California, Riverside, United StatesYongjie Yu, Nanjing University of Information Science and Technology, China
Zongbao Liu, Guangxi Normal University, China
Copyright © 2023 Bowman, Heath, Varga, Battu, Chu, Toyoda, Cheeke, Porter, Moffett, LeTendre, Qafoku, Bargar, Mans, Hess and Graham. This is an open-access article distributed under the terms of the Creative Commons Attribution License (CC BY). The use, distribution or reproduction in other forums is permitted, provided the original author(s) and the copyright owner(s) are credited and that the original publication in this journal is cited, in accordance with accepted academic practice. No use, distribution or reproduction is permitted which does not comply with these terms.
*Correspondence: Emily B. Graham, ZW1pbHkuZ3JhaGFtQHBubmwuZ292