- 1Department of Agricultural and Environmental Sciences, College of Agriculture, Tennessee State University, Nashville, TN, United States
- 2Department of Microbiology and Plant Biology, University of Oklahoma, Norman, OK, United States
The effects of organic and conventional production systems on crop productivity have been greatly explored, but their effects on soil microbial processes were often neglected. A comparative field study of organic and conventional production systems was conducted at the Tennessee State University research farm to determine soil heterotrophic respiration and microbial biomass carbon. Leafy green vegetables were grown in a conventional production system in an open field, and they were grown in an organic production system, using three different row covers (agribon cloth, insect net, and plastic), and in an open field. Soil samples (0-15cm) were collected from the two production systems. Soil heterotrophic respiration rate (RH), microbial biomass carbon (MBC), and biomass-specific heterotrophic respiration rate (the inverse is used as a proxy for microbial carbon use efficiency) were quantified. The results showed that the conventional production system significantly increased RH relative to the organic system. Organic production system, however, significantly enhanced MBC and reduced biomass-specific respiration rate indicating an increase in carbon use efficiency. Although MBC remained unchanged among the row covers, insect net increased RH and biomass-specific heterotrophic respiration rate. Our results suggest that the organic production system not only promoted soil microbial abundance but also limited soil heterotrophic respiration to the atmosphere governed by the elevated carbon use efficiency.
Introduction
The soil microbial activity is an important soil quality parameter and is crucial for nutrient cycling in an agro-ecosystem (1, 2). Soil microbial biomass, the living component of the soil (3), and microbial respiration are often used to monitor changes in microbial activities in the soil (4, 5). Microbial respiration is the amount of CO2 carbon released to the atmosphere from the microbial decomposition of soil organic matter (6). The soil microbial activities are crucial for plant growth and development because nutrients taken by plants must be decomposed and mineralized by soil microorganisms from organic to inorganic form (2). Furthermore, soil microbes convert plant residues and organic matter into soil organic carbon (7). An understanding of soil microbial activities is important for the management of production systems (e.g., organic vs conventional). However, studies comparing changes in microbial activities between those two production systems are very limited.
A general conclusion is that organic production system can improve soil quality by enhancing soil organic carbon and stimulating soil microbial activities (8, 9). Organic matter is the main source of plant nutrients which is mostly supplied through organic manures, sewage waste, compost, cover crops, among others, and thus higher amounts of organic carbon are accumulated in the soil (10–12). Since soil microbial activities are directly related with the amount of carbon content in the soil (13, 14), differences in microbial activities are expected when two production systems are different in organic carbon contents (13). As such, organic production systems are expected to have stimulated microbial activities compared to conventional production system (15, 16). Nevertheless, inconsistent microbial responses to production systems are also observed. For instance, soil with higher organic matter had higher or no changes in soil respiration compared to the conventional system (17–19). Likewise, soil microbial biomass was increased or remained unchanged, likely associated with the different quality of organic inputs (20). These inconsistent responses signify the importance of further study comparing between two production systems for soil microbial activities.
In the current study, the effects of organic and conventional production systems on soil microbial activities were evaluated. Moreover, in the organic system, leafy greens were produced under row covers and in open field. Row covers have been widely used as mini tunnels by organic growers for leafy greens to protect crops from frost, insect pests, and for a season extension (21–23). Different types of row covers such as agribon cloth, insect net, and plastic are most commonly used. This study aims to determine soil heterotrophic respiration and microbial biomass carbon in organic and conventionally managed leafy green vegetable production systems. Additionally, this study seeks to understand the impacts of different row covers on soil heterotrophic respiration and microbial biomass carbon under organic production system. We hypothesized that the organic production system would increase soil heterotrophic respiration and microbial biomass associated with organic matter inputs. Furthermore, we hypothesized that row covers would enhance soil heterotrophic respiration and microbial biomass.
Materials and methods
Experimental design and layout
A field experiment was carried out in organic and conventional fields of Tennessee State University, Nashville, TN (Latitude 36° 10’ N Longitude 86° 49’ W) in March-May of 2019. The soil texture was sandy loam with a pH of 6.5 in organic and 6.0 in the conventional field. The soil carbon, total nitrogen, and C: N ratios were 1.64%, 0.16%, and 10.21, respectively in the organic field and 1.75%, 0.17%, and 10.05, respectively in the conventional field. The mean monthly temperatures were 49.0, 62.1, and 72.8°F and total precipitation were 3.6, 6.3, and 1.6 inches in March, April, and May, respectively.
The field experiment was laid out in a completely randomized design with three replications both in organic and conventional fields. Leafy greens were planted on March 11, 2019. Collard (Brassica oleracea cv. acephala var. champion), kale (Brassica oleracea cv. sabellica var. red Russian), lettuce (Lactuca sativa var. coastal star) and swiss chard (Beta vulgaris var. ford hook giant) were grown in an organic management system under three different row covers: agribon cloth (Ag-19 made from high-quality spun-bonded polypropylene), insect net (0.35 mm mesh size), and plastic film (ultra-clear transparent, Johnny selected seeds Co., ME, USA) and without row cover (Open) representing control in an organic field. Same varieties of four leafy greens were also grown in a conventional field using chemical fertilizer. Row covers were not used in conventional system. Tested plants were applied with nitrogen, phosphorus, and potassium fertilizer at the rate of 5 g/plant in conventional system and compost 100 g/plant in organic system. The composition of chemical fertilizer has nitrogen, phosphorus, and potassium of 8:2:12. The composition of compost was 0.46% total nitrogen, 46.80% moisture, 7.93 pH, 1580 mg/kg total phosphorus, and 4290 mg/kg total potassium. Crops in conventional production systems were grown in open field. In the organic field, plants in the plots were immediately covered after planting with row covers which were supported by wire hoops 2’ above the ground.
Soil sampling
Soil samples were collected at 0-15 cm soil depth from both organic and conventional fields immediately after crop harvest in May 2019. Across the treatments, soil samples were collected from three spots per replication and thoroughly mixed within a plastic bag and composite sample was taken for analysis. The composite samples were transported to the laboratory in an ice pack cooler. Stones and roots were removed from the samples and sieved through a 2 mm screen and stored at 39.2°F before analysis.
Microbial biomass carbon
Soil subsample (5 g) was weighed in a 50 ml centrifuge tube and fumigated with 1 ml ethanol-free chloroform for 24 h in the fume hood. Another equivalent weight subsample was weighed but kept unfumigated. Twenty-five ml of 0.5M potassium sulfate (K2SO4) was added to fumigated and unfumigated subsamples and shaken on a mechanical shaker for 30 minutes. The extracts were filtered through Whatman #4 filter paper using a vacuum pump. The soil extracts (5 ml) and persulfate reagent (5 ml) were added to culture tubes for both fumigated and unfumigated and placed into a drying oven set at 85-90°C for 18 h (24). The tubes were removed from the oven and cooled to room temperature before analysis. Extractable organic carbon was measured using a total organic carbon and nitrogen analyzer (Shimadzu Corp., Kyoto, Japan). The microbial biomass carbon was determined by subtracting extractable organic carbon in the unfumigated samples from that in the fumigated samples. An extraction coefficient of 0.45 was used (25).
Soil heterotrophic respiration rate and biomass-specific heterotrophic respiration rate
The soil heterotrophic respiration rate was measured by using Picarro G2131-i analyzer (Picarro Inc., Santa Clara, CA, USA). Field soil subsamples, equivalent to 10 g, were put in 7.5 cm tall PVC cores (5 cm diameter). One side of the PVC core was sealed using glass fiber paper and was placed in a 1 L capacity mason jar lined with marbles at the bottom. The total CO2 concentration in the jar was measured by connecting the mason jars to a Picarro G2131-i analyzer. The amount of CO2 produced overtime was used to estimate the respiration rate based on dry soil weight. Biomass-specific heterotrophic respiration rate was calculated by dividing soil heterotrophic respiration rate by microbial biomass carbon and it was used to index microbial physiology (26). The inverse of biomass-specific heterotrophic respiration rate was used as a proxy for microbial carbon use efficiency.
Statistical analysis
Student’s t-test (5% confidence interval) was used to examine the effects of organic and conventional production systems on soil heterotrophic respiration rate, microbial biomass carbon, and biomass-specific heterotrophic respiration rate using SAS 9.4 (SAS, Inc., Cary, NC). One-way analysis of variance (ANOVA) in SAS 9.4 was used to examine the effects of row covers on soil heterotrophic respiration rate, microbial biomass carbon, and biomass-specific heterotrophic respiration rate. Data met the assumption of normal distribution and equal variance. When significant differences among the treatments were detected, a post hoc test with the Fisher’s least significant difference (LSD) was performed for the multiple comparisons. The significance level was set at P<0.05.
Results
Microbial biomass carbon
Microbial biomass carbon was increased by 163.6% in the soil collected from organic production system (2.9 mg-C gsoil-1) than conventional production system (1.1 mg-C gsoil-1; P<0.05; Figure 1A). Among the row covers used in the organic production system, there were no differences in MBC, which were 2.1, 2.7, 2.5, and 2.9 mg-C gsoil-1 in agribon cloth, insect net, plastic, and open, respectively (P=0.1510; Figure 1B).
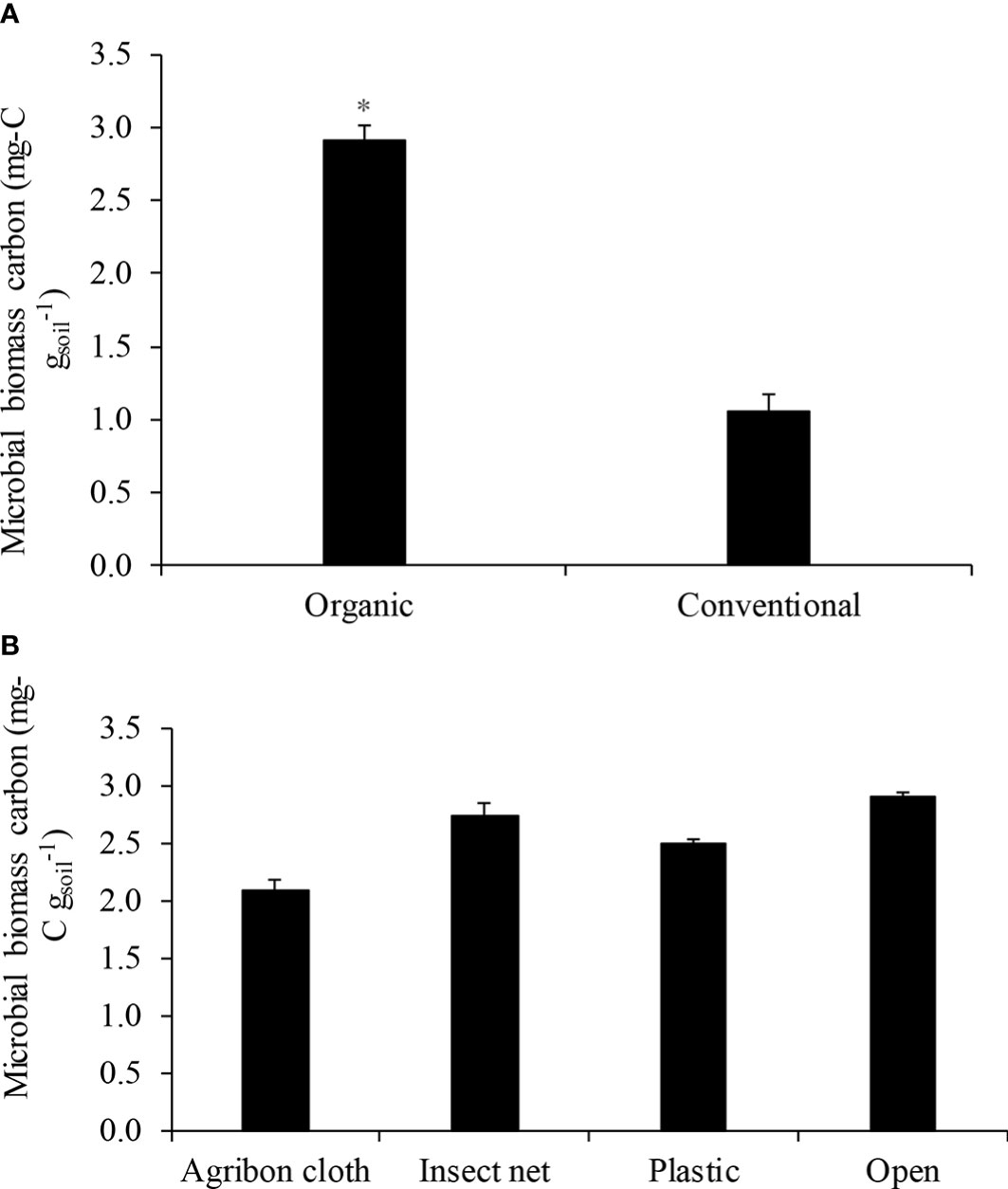
Figure 1 Mean ( ± SE) microbial biomass carbon (MBC) measured in (A) organic and conventional production systems; and (B) different row covers used in organic production system. Asterisk (*) sign indicates a significant difference.
Soil heterotrophic respiration rate and biomass-specific heterotrophic respiration rate
Conventional production system significantly increased soil heterotrophic respiration rate by 100.0% (0.4 µg CO2-C gsoil-1 h-1) compared to organic system (0.2 µg CO2-C gsoil-1 h-1; P<0.05; Figure 2A). Within the organic production system, RH was significantly higher in insect net than the other treatments (P<0.05; Figure 2B). RH was 0.4, 0.9, 0.3, and 0.2 µg CO2-C gsoil-1 h-1 in agribon cloth, insect net, plastic, and open, respectively. Biomass-specific heterotrophic respiration rate was 300.0% higher in the conventional production system (0.4 µg CO2-C mgmbc-1) compared to organic (0.07 µg CO2-C mgmbc-1; Figure 3A). Among different row covers used in organic system, insect net had higher specific respiration rate compared to open (P<0.05; Figure 3B). Biomass-specific respiration rate was 0.2, 0.4, 0.1, 0.07 µg CO2-C mgmbc-1 in agribon cloth, insect net, plastic, and open, respectively.
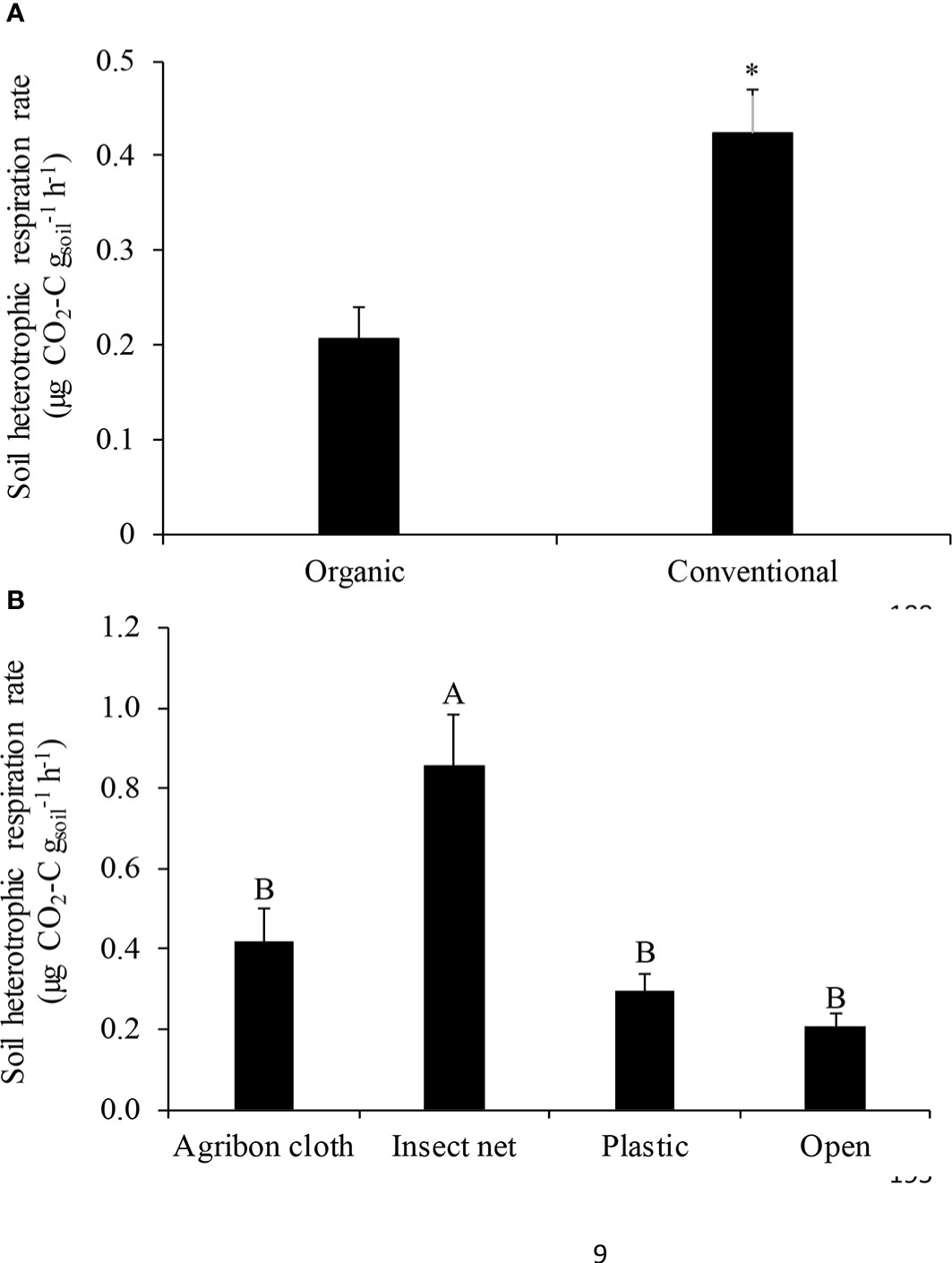
Figure 2 Mean ( ± SE) soil heterotrophic respiration rate measured in (A) organic and conventional production systems; and (B) different row covers used in organic production system. Asterisk (*) sign indicates a significant difference. Different letters in the column represent significant differences at p <0.05.
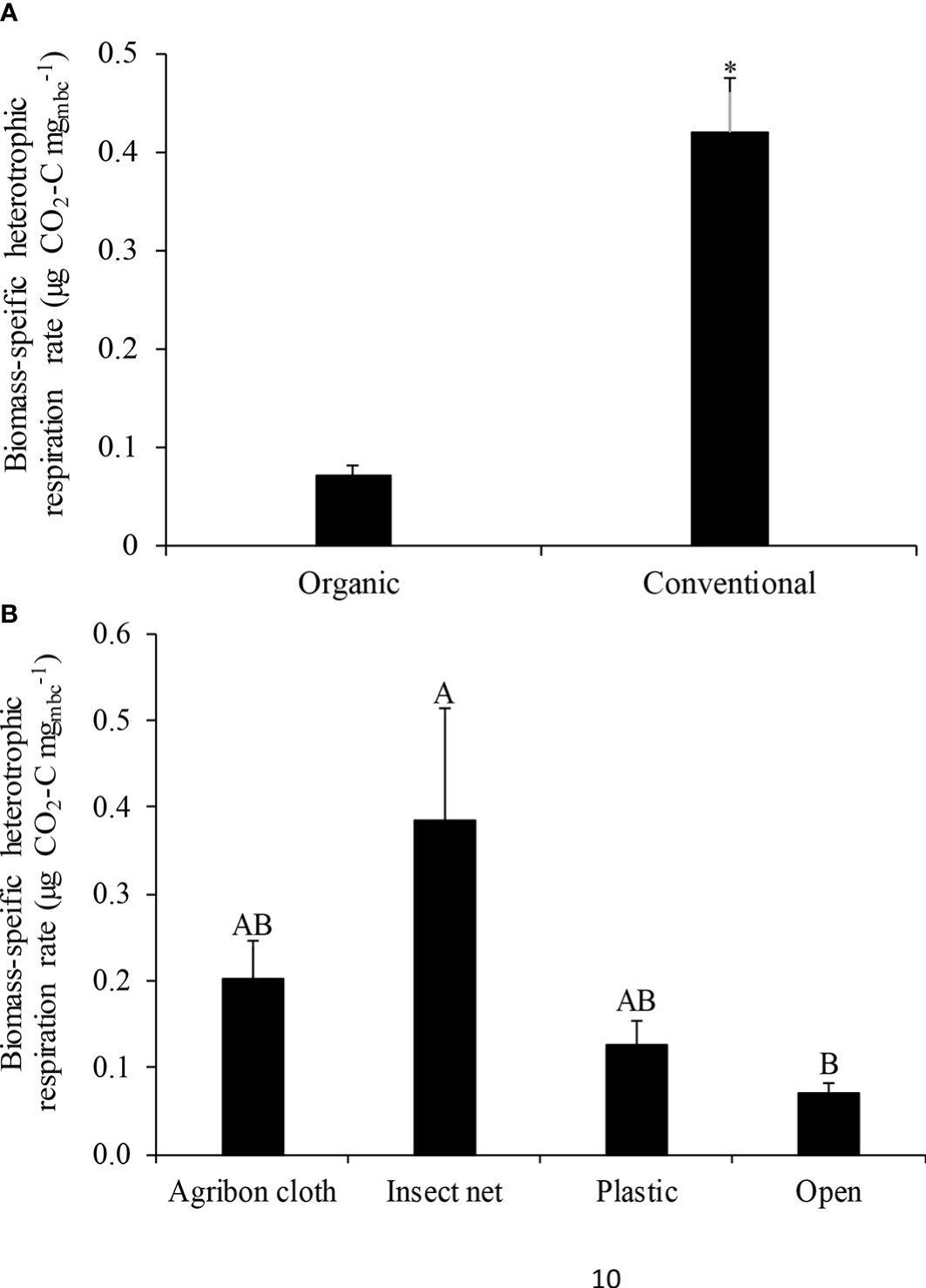
Figure 3 Mean ( ± SE) biomass-specific heterotrophic respiration measured in (A) organic and conventional production systems; and (B) different row covers used in organic production system. Asterisk (*) sign indicates a significant difference. Different letters in the column represent significant differences at p <0.05.
Discussion
As we hypothesized, the organic production system significantly stimulated MBC relative to the conventional production system but RH was significantly enhanced by the conventional system. Biomass-specific heterotrophic respiration rate was significantly higher in conventional system relative to organic system. Although row covers had no effects on MBC, RH and biomass-specific heterotrophic respiration rate were higher in insect net than other treatments. As such, our hypotheses were partially supported.
Soil microbial biomass regulates nutrient cycling in the soil and is strongly affected by production systems (i.e., organic vs conventional) (27). The increased microbial biomass and the activities increase the availability of soil nutrients to the plants (28). In our study, MBC was enhanced by the organic production system relative to the conventional production system. Our findings are in agreement with the previous studies (29–32). A meta-data analysis study found that organic agriculture can increase MBC by as much as 32% over conventional practices (33). The higher MBC under organic system was likely associated with the increased organic matter inputs (34). The application of organic matter also adds microbes because compost is rich in the microbial population (1). In contrast, lower microbial biomass in conventional farming practices was possibly due to lower organic carbon inputs (35, 36). The direct toxicity of chemical fertilizers and reduced pH can also lead to reduced MBC in conventional production system (37, 38). Moreover, biomass-specific heterotrophic soil respiration rate was low in organic system indicating higher microbial carbon use efficiency. This might be the other reason for higher MBC in the organic production system (36), suggesting that microbes allocated more carbon for their growth than for the maintenance in the organic system. We did not observe significant influence of row covers on MBC. The quantity of organic carbon inputs from the external source was the same for row covers and open, meaning that they received the same quantity of microbial loads. Although we observed higher crop production in row covers than in open (23), their effects were not observed in the soil microbial population. Since aboveground biomass was harvested, this is likely that the amount of carbon inputs from below-ground biomass was the same for all treatments. RH was higher in the conventional system possibly due to observed higher biomass-specific heterotrophic respiration (i.e., reduced carbon use efficiency). Microorganisms in organic management system have higher carbon use efficiency because they utilize carbon more efficiently in growth in favorable soil conditions (36). In the conventional system, microbes might have partitioned more carbon for maintenance than the growth. Our finding was supported by previous studies that reported elevated RH in conventional than in organic system (27, 32). Furthermore, reduced RH in the organic system is likely associated with the tendency of microbes to the formation of polysaccharides with the increase in soil organic carbon (39). Nevertheless, our finding is contradicted by several other studies that found higher RH in the organic system compared to the conventional system (1, 18, 40). These differences in responses to production systems are possibly associated with the quality of organic inputs and microbial community composition. Further long-duration studies may provide more evidence to reach conclusions. Moreover, we observed higher RH in insect net than in other row covers. Although the exact mechanism why respiration increased in insect net is not clear, we assumed that this is possibly associated with higher carbon inputs from plants (both aboveground and belowground). Also, the soil moisture in the insect net was about 17.0%, which was lower than soil moisture in other row covers (41). It is possible that RH is maximum at this moisture level and reduces with a further increase in soil moisture as observed by Parajuli (5).
Conclusions
The conventional production system stimulated CO2 respiratory carbon losses from the soil to the atmosphere compared to the organic system. The organic production system partitioned the majority of assimilated carbon for microbial growth leading to higher microbial biomass likely associated with increased carbon use efficiency. Thus, the increased microbial biomass and activity increase the availability of nutrients to the plants. Our results suggest that organic production system can promote the soil microbial population by increasing the carbon use efficiency, together with limiting the CO2 losses to the atmosphere from the soil.
Data availability statement
The original contributions presented in the study are included in the article/supplementary material. Further inquiries can be directed to the corresponding author.
Author contributions
KD, preparation of manuscript and data collection. DN, overall supervision, review of manuscript, and research investigator. MP, data collection and manuscript preparation. SJ, assistance in field and lab research. JL, experimental design and review. All authors contributed to the article and approved the submitted version.
Funding
Funding for the research received from the Tennessee State University (TSU) Cooperative Extension project to the corresponding author (DN).
Acknowledgments
We would like to thank TSU farm staff and the organic agriculture research team of Tennessee State University for helping with the field experiments and soil sampling.
Conflict of interest
The authors declare that the research was conducted in the absence of any commercial or financial relationships that could be construed as a potential conflict of interest.
Publisher’s note
All claims expressed in this article are solely those of the authors and do not necessarily represent those of their affiliated organizations, or those of the publisher, the editors and the reviewers. Any product that may be evaluated in this article, or claim that may be made by its manufacturer, is not guaranteed or endorsed by the publisher.
References
1. Masto RE, Chhonkar PK, Singh D, Patra AK. Changes in soil biological and biochemical characteristics in a long-term field trial on a sub-tropical inceptisol. Soil Biol Biochem (2006) 38(7):1577–82. doi: 10.1016/j.soilbio.2005.11.012
2. Brennan EB, Acosta-Martinez V. Cover cropping frequency is the main driver of soil microbial changes during six years of organic vegetable production. Soil Biol Biochem (2017) 109:188–204. doi: 10.1016/j.soilbio.2017.01.014
3. Sparling GP. The Soil Biomass. In: Vaughan D, Malcolm RE (eds) Soil Organic Matter and Biological Activity. Developments in Plant and Soil Sciences. (Dordrecht: Springer) (1985) p. 223–62. doi: 10.1007/978-94-009-5105-1_7
4. Horwath WR, Paul EA. Microbial biomass. In: Weaver RW, Angle JS, Bottomley PJ, Bezdicek DF, Smith MS, Tabatabai MA, et al Eds, Methods of soil analysis: Part 2 microbiological and biochemical properties, (Madison, WI, USA: Soil Science Society of America and American Society of Agronomy). (1994) 5:753–74. doi: 10.2136/sssabookser5.2.c36
5. Parajuli M. Responses of soil respiration and extracellular enzyme activities to manipulated precipitation regimes in a switchgrass mesocosm experiment. Tennessee State University (2020). ProQuest. Master's Thesis.
6. Raich JW, Schlesinger WH. The global carbon dioxide flux in soil respiration and its relationship to vegetation and climate. Tellus B (1992) 44(2):81–99. doi: 10.3402/tellusb.v44i2.15428
7. Chen G, Zhu H, Zhang Y. Soil microbial activities and carbon and nitrogen fixation. Res Microbiol (2003) 154(6):393–8. doi: 10.1016/S0923-2508(03)00082-2
8. Mondelaers K, Aertsens J, Van Huylenbroeck G. A meta-analysis of the differences in environmental impacts between organic and conventional farming. Br Food J (2009) 111(10):1098–119. doi: 10.1108/00070700910992925
9. Araújo ASF, Melo WJD. Soil microbial biomass in organic farming system. Ciec Rural (2010) 40:2419–26. doi: 10.1590/S0103-84782010001100029
10. Rosen CJ, Allan DL. Exploring the benefits of organic nutrient sources for crop production and soil quality. HortTechnology (2007) 17(4):422–30. doi: 10.21273/HORTTECH.17.4.422
11. Gattinger A, Muller A, Haeni M, Skinner C, Fliessbach A, Buchmann N, et al. Enhanced top soil carbon stocks under organic farming. Proc Natl Acad Sci (2012) 109(44):18226–31. doi: 10.1073/pnas.1209429109
12. Novara A, Pulido M, Rodrigo-Comino J, Di Prima S, Smith P, Gristina L, et al. Long-term organic farming on a citrus plantation results in soil organic carbon recovery. Cuadernos Investigación Geográfica (2019) 45(1):271–86. doi: 10.18172/cig.3794
13. Iovieno P, Morra L, Leone A, Pagano L, Alfani A. Effect of organic and mineral fertilizers on soil respiration and enzyme activities of two Mediterranean horticultural soils. Biol Fertility Soils (2009) 45(5):555–61. doi: 10.1007/s00374-009-0365-z
14. Zhao Y, Wu S, Bol R, Bughio MA, Wu W, Hu Y, et al. Intensive organic vegetable production increases soil organic carbon but with a lower carbon conversion efficiency than integrated management. J Plant Nutr Soil Sci (2020) 183(2):155–68. doi: 10.1002/jpln.201900308
15. Alvarez CR, Alvarez R, Grigera MS, Lavado RS. Associations between organic matter fractions and the active soil microbial biomass. Soil Biol Biochem (1998) 30(6):767–73. doi: 10.1016/S0038-0717(97)00168-5
16. Santos VB, Araújo ASF, Leite LFC, Nunes LAPL, Melo WJ. Soil microbial biomass and organic matter fractions during transition from conventional to organic farming systems. Geoderma (2012) 170:227–31. doi: 10.1016/j.geoderma.2011.11.007
17. Leskovar D, Othman Y, Dong X. Strip tillage improves soil biological activity, fruit yield and sugar content of triploid watermelon. Soil tillage Res (2016) 163:266–73. doi: 10.1016/j.still.2016.06.007
18. Leskovar D, Othman YA. Organic and conventional farming differentially influenced soil respiration, physiology, growth and head quality of artichoke cultivars. J Soil Sci Plant Nutr (2018) 18(3):865–80. doi: 10.4067/S0718-95162018005002502
19. Mátyás B, Andrade MEC, Chida NCY, Velasco CMT, Morales DEG, Montero GNM, et al. Comparing organic versus conventional soil management on soil respiration. F1000Research (2018) 7:258. doi: 10.12688/f1000research.13852.1
20. Gunapala N, Scow KM. Dynamics of soil microbial biomass and activity in conventional and organic farming systems. Soil Biol Biochem (1998) 30(6):805–16. doi: 10.1016/S0038-0717(97)00162-4
21. Otto RF, Gimenez C, Castilla N. Microclimatic modifications under polypropylene protection for horticultural crops in córdoba, Spain. Horticultura Bras (2000) 18(3):204–11. doi: 10.1590/S0102-05362000000300013
22. Hochmuth GJ, Hochmuth RC, Kostewicz SR, Stall WM. Row covers for commercial vegetable culture in Florida. University of Florida, Institute of Food and Agricultural Sciences, Florida Cooperative Extension Service (2005) Circular 728.
23. Dhakal K, Nandwani D. Evaluation of row covers for yield performance of the leafy green vegetables in organic management system. Organic Agric (2020) 10:27–33. doi: 10.1007/s13165-020-00298-z
24. Vance ED, Brookes PC, Jenkinson DS. An extraction method for measuring soil microbial biomass c. Soil Biol Biochem (1987) 19(6):703–7. doi: 10.1016/0038-0717(87)90052-6
25. Joergensen RG. The fumigation-extraction method to estimate soil microbial biomass: calibration of the kEC value. Soil Biol Biochem (1996) 28(1):25–31. doi: 10.1016/0038-0717(95)00102-6
26. Bradford MA, Davies CA, Frey SD, Maddox TR, Melillo JM, Mohan JE, et al. Thermal adaptation of soil microbial respiration to elevated temperature. Ecol Lett (2008) 11(12):1316–27. doi: 10.1111/j.1461-0248.2008.01251.x
27. Araújo ASF, Leite LF, Santos VB, Carneiro RF. Soil microbial activity in conventional and organic agricultural systems. Sustainability (2009) 1(2):268–76. doi: 10.3390/su1020268
28. Tu C, Ristaino JB, Hu S. Soil microbial biomass and activity in organic tomato farming systems: Effects of organic inputs and straw mulching. Soil Biol Biochem (2006) 38(2):247–55. doi: 10.1016/j.soilbio.2005.05.002
29. Peacock AG, Mullen MD, Ringelberg DB, Tyler DD, Hedrick DB, Gale PM, et al. Soil microbial community responses to dairy manure or ammonium nitrate applications. Soil Biol Biochem (2001) 33(7-8):1011–9. doi: 10.1016/S0038-0717(01)00004-9
30. Martini EA, Buyer JS, Bryant DC, Hartz TK, Denison RF. Yield increases during the organic transition: improving soil quality or increasing experience? Field Crops Res (2004) 86(2-3):255–66. doi: 10.1016/j.fcr.2003.09.002
31. Kaur K, Kapoor KK, Gupta AP. Impact of organic manures with and without mineral fertilizers on soil chemical and biological properties under tropical conditions. J Plant Nutr Soil Sci (2005) 168(1):117–22. doi: 10.1002/jpln.200421442
32. Araújo ASF, Santos VB, Monteiro RTR. Responses of soil microbial biomass and activity for practices of organic and conventional farming systems in piauí state, Brazil. Eur J Soil Biol (2008) 44(2):225–30. doi: 10.1016/j.ejsobi.2007.06.001
33. Lori M, Symnaczik S, Mäder P, De Deyn G, Gattinger A. Organic farming enhances soil microbial abundance and activity-a meta-analysis and meta-regression. PLoS One (2017) 12(7):e0180442. doi: 10.1371/journal.pone.0180442
34. Okur N, Altindİşlİ A, Çengel M, Göçmez S, Kayikçioğlu HH. Microbial biomass and enzyme activity in vineyard soils under organic and conventional farming systems. Turkish J Agric Forestry (2009) 33(4):413–23. doi: 10.3906/tar-0806-23
35. Wardle DA. A comparative assessment of factors which influence microbial biomass carbon and nitrogen levels in soil. Biol Rev (1992) 67(3):321–58. doi: 10.1111/j.1469-185X.1992.tb00728.x
36. Fließbach A, MaÈder P. Microbial biomass and size-density fractions differ between soils of organic and conventional agricultural systems. Soil Biol Biochem (2000) 32(6):757–68. doi: 10.1016/S0038-0717(99)00197-2
37. Hopkins DW, Shiel RS. Size and activity of soil microbial communities in long-term experimental grassland plots treated with manure and inorganic fertilizers. Biol Fertility Soils (1996) 22(1):66–70. doi: 10.1007/BF00384434
38. Bittman S, Forge TA, Kowalenko CG. Responses of the bacterial and fungal biomass in a grassland soil to multi-year applications of dairy manure slurry and fertilizer. Soil Biol Biochem (2005) 37(4):613–23. doi: 10.1016/j.soilbio.2004.07.038
39. Sher Y, Baker NR, Herman D, Fossum C, Hale L, Zhang X, et al. Microbial extracellular polysaccharide production and aggregate stability controlled by switchgrass (Panicum virgatum) root biomass and soil water potential. Soil Biol Biochem (2020) 143:107742. doi: 10.1016/j.soilbio.2020.107742
40. Ajwa HA, Tabatabai MA. Decomposition of different organic materials in soils. Biol Fertility Soils (1994) 18(3):175–82. doi: 10.1007/BF00647664
Keywords: biomass-specific soil respiration, conventional, organic, row covers, soil biological process
Citation: Dhakal K, Parajuli M, Jian S, Li J and Nandwani D (2022) Responses of soil heterotrophic respiration and microbial biomass to organic and conventional production systems. Front. Soil Sci. 2:999139. doi: 10.3389/fsoil.2022.999139
Received: 20 July 2022; Accepted: 25 August 2022;
Published: 12 September 2022.
Edited by:
Hassan Ragab El-Ramady, Kafrelsheikh University, EgyptReviewed by:
Alaa El-Dein Omara, Agricultural Research Center (Egypt), EgyptTamer Elsakhawy, Agricultural Research Center (Egypt), Egypt
Copyright © 2022 Dhakal, Parajuli, Jian, Li and Nandwani. This is an open-access article distributed under the terms of the Creative Commons Attribution License (CC BY). The use, distribution or reproduction in other forums is permitted, provided the original author(s) and the copyright owner(s) are credited and that the original publication in this journal is cited, in accordance with accepted academic practice. No use, distribution or reproduction is permitted which does not comply with these terms.
*Correspondence: Dilip Nandwani, ZG5hbmR3YW5AdG5zdGF0ZS5lZHU=