- 1Faculty of Engineering and Physical Sciences, University of Southampton, Southampton, United Kingdom
- 2National Oceanography Centre, European Way, Southampton, United Kingdom
- 3Air Quality Management Resource Centre, University of the West of England, Bristol, United Kingdom
- 4School of Geography and Environmental Science, Faculty of Environmental and Life Sciences, University of Southampton, Southampton, United Kingdom
Biosolids are the solid by-product of the wastewater treatment system. They are regularly applied to agricultural land in the UK to fertilize and increase crop yields, but they have been shown to contain high concentrations of microplastics. Here we sampled a selection of agricultural soils in the Southeast of England which had received or never received biosolid treatment. Sites were sampled on two occasions in the summer and winter. Microplastic (MP) numbers were high in both the biosolid treated fields (874 MP/kg) and the untreated fields (664 MP/kg) and a wide variety of polymers were found across sites. However, there was a lack of significant difference between treated and untreated soils. This suggests the influence of other microplastic sources e.g. agricultural plastic and general littering, and external conditions e.g. farm management and rainfall. Microplastic concentrations were higher in the summer suggesting that erosion, runoff, and wind transport may be removing microplastics from these systems. The dynamic nature of the agricultural soils may result in them becoming a vector for microplastics into the wider environment. The high variability in results seen here highlights the complexity of microplastic concentrations in heterogeneous agricultural soils. This study suggests that biosolids, whilst are likely a contributor, are not the sole source of microplastics in agricultural soils. Further research is required to determine source and sink dynamics in these systems. Understanding the sources of microplastic contamination in soils is imperative for future mitigation strategies to be effective.
1 Introduction
Microplastics (< 5 mm) are globally recognized as ubiquitous contaminants with the potential for long-lasting residence in the environment. As plastic demand continues to rise, with production rates already exceeding 360 Mt (1), it is inevitable that plastic pollution environment will also increase. This is especially true within the terrestrial environment, as estimates suggest that 80% of the plastic litter in the ocean originated on land (2). Meanwhile, there is growing evidence to suggest that terrestrial soils receive microplastics from varying sources and indications that they cause negative impacts on soil ecosystems. When added to soils, microplastics have the potential to alter their physical properties such as bulk density and water holding capacity, with consequences for plant growth (3).
Of particular concern are agricultural soils. They are considered to have high microplastic inputs from plasticulture, fertilizers, atmospheric deposition, irrigation, littering, and surface runoff (4). Managed land is likely to have elevated numbers of microplastics (5), likely due to primary anthropogenic activities, particularly agricultural practices. Of high concern amongst these practices is the use of organic fertilizer, including the application of composts (6), animal manures (7), and biosolids (8). Biosolids (also known as sewage sludge) are a by-product of wastewater treatment, which, in many countries are often applied to land as a fertilizer to improve agricultural yields by increasing essential elements such and nitrogen and phosphorous. Some countries (e.g. Switzerland) have placed restrictions on the use of biosolid application to land based on the potential impact of persistent pollutants (9, 10). However, in the UK, almost all biosolids produced are spread on agricultural land (11). Wastewater treatment systems are designed to primarily remove biochemical oxygen demand, suspended solids, and ammonia. The settling of suspended solids during the treatment process results in the generation of biosolids, which includes detectable concentrations of pharmaceutical compounds and metals that partition to the solids (12). With respect to microplastics, while wastewater treatment systems are reported to remove up to 99.8% of microplastics from final effluents, these microplastics instead end up in the solid fraction resulting in their incorporation into biosolids (13). Numbers of microplastics have been steadily increasing in biosolids since the 1950s (14) with current estimates suggesting millions of microplastic particles per kilogram of biosolid (13, 15, 16). Various sources have been suggested to contribute to these microplastics loads including washing of synthetic textiles (17), personal care products, industrial plastic particles and road runoff (including tire wear particles) (18)
Given these estimates, alongside high rates of biosolids applied to land which were reported in 2010 as 1,118,159 tonnes in the UK (19), microplastic quantities in soils where sludge is applied are expected to be high. To date, varying concentrations have been reported. While some studies have found biosolid augmented soils to have high microplastic concentrations, up to 10,400 microplastics per kilogram (MP/kg; 20), others have found lower numbers, up to 288 MP/kg, (21). Higher concentrations, > 2000 MP/kg have been observed in soils without previous biosolid treatment (22). Theoretical calculations of soil microplastic concentrations have been made in relation biosolid application (12, 23). In the UK alone, it is suggested that 2.7 × 1015 microplastics are applied to agricultural soils annually (13). However, there are few real-world data relating to microplastic contamination in soils. To date, only 9.2% of microplastic studies looking at soil and biosolid matrices focused specifically on biosolid amended soils (24).
To be able to target and mitigate microplastic contamination in agricultural soils, sources must first be defined and quantified. The aim of this study was therefore to measure and compare microplastic contamination in agricultural soils with and without biosolid amendment within a defined geographical region (River Test, catchment, southern UK). Based on observed contamination, recommendations for programs of measures and priorities for further research are made.
2 Methods
2.1 Study area
Study sites were chosen in the catchment area of the River Test, Hampshire, UK (Figure 1). The focus was on one catchment as this location comprises a well-defined landscape unit which allow for assessment of microplastics at an integrating scale (25). Characteristics of the catchment area (e.g., slopes and length of river, land use, and soil properties) may be used to inform the potential for diffuse pollution, including microplastics (23). The River Test is a designated SSSI (Site of Special Scientific Interest) chalk-bed river (26). It is 139 km in length, drains an area of 1269 km2 ( (27, 28) and feeds into Southampton water through the Solent estuary. Its catchment area is predominantly rural with high agricultural land use (29) and comprises of two main soil types, the Andover and Carstens associations (30). The Andover series is a chalky soil which tends to be shallow and silty with high calcareous content; while the Carstens series is majorly clay based, often with a high flint content. Both have a low carbon content, loamy texture, are freely draining, and commonly utilized for grassland and arable agriculture (30).
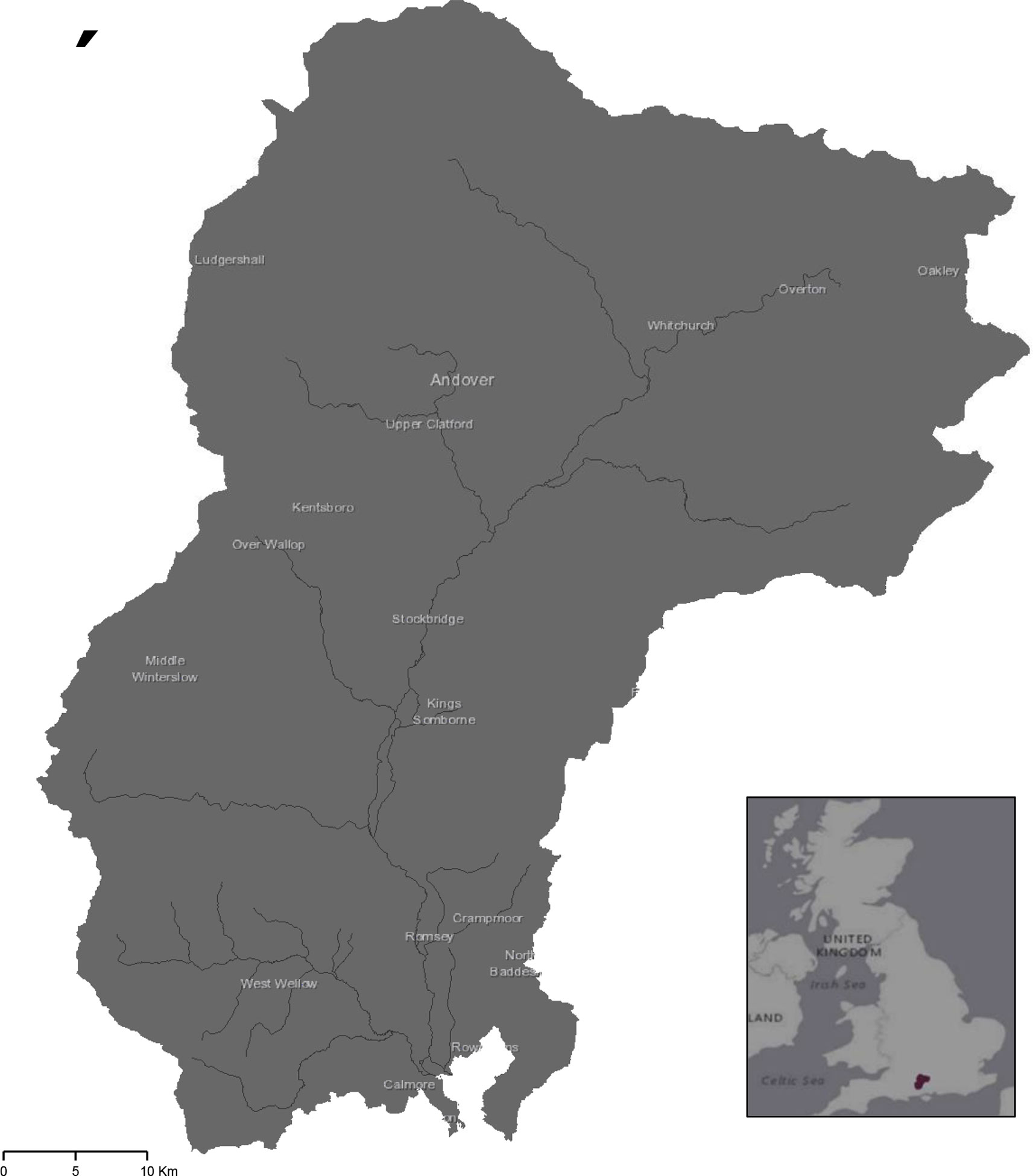
Figure 1 The River Test catchment area in Hampshire, UK from which samples were collected from 10 fields. Exact locations of farms are concealed for anonymity.
2.2 Sample collection
Ten arable fields were selected, five of which had historically been treated with biosolids and five that had never received biosolids. Fields were selected to minimize environmental variation between sites. Samples were taken from each field on two occasions during summer (August 2019) and winter (February 2020) seasons. The average rainfall in the month of the summer sampling occasion was 51mm in the South East of England, and 122mm in the month of the winter sampling occasion (31).
Environmental factors, including land use and farm management, were accounted for as much as possible when considering the samples to reduce the influence of confounding factors on observations and interpretation thereof. All selected fields were for arable use and had similar agricultural regimes with a typical crop rotation of winter and spring cereal crops and break crop (typically winter wheat, barley, or oilseed rape). Cultivation in all fields was done by using a minimum tillage method, meaning that soil is rotated only in the top layer (typically <10cm). In the treated fields, biosolids were all from the same supplier and had been applied once within the last six months using a spraying method. No significant plasticulture was used on any of the farms (i.e. plastic mulching or the use of plastic film and tunnel covers).
In anticipation of the likely variability within fields (32), four replicate samples were taken per field, per sampling occasion, to increase the precision of microplastic measurements. Samples were taken using a stainless-steel pot corer (3 cm diameter, 10 cm height). Each field was split into four quarters, excluding a 4 m buffer zone around the edge to exclude external influences (33), and a composite sample was taken for each quarter. Each composite sample was composed of 25 cores taken at random points in accordance with the Sludge (Use in Agriculture) Regulations for soil testing (34), and homogenized by thoroughly mixed in a stainless-steel bucket with a metal trowel. A subsample of approximately 300 mL was then removed and stored in a glass jar with a metal lid. Samples were subsequently stored in darkness at ambient temperature.
2.3 Site characterization
Environmental factors, such as soil and landscape characteristics, that could not be externally controlled were measured and accounted for in the analysis and interpretation. Soil and field characteristics, including organic matter content and soil particle size distribution, and site characteristics, including slope and distance from roads, were measured. For each field, a further composite sample was taken for these measurements. For each sampling occasion (n=20) equal proportions of the original four replicates were combined for analysis. Soil organic matter content was measured using loss-on-ignition (LOI) at 550°C. Soil particle size distribution was measured by sieving for the coarse fraction above 1 mm, and a Malvern Mastersizer 3000 granulometer for the <1 mm fraction. Additionally, these samples were characterized using X-ray fluorescence (XRF) for elemental composition. Metals that are commonly regulated for in biosolids (zinc, copper, nickel; 34) were measured as a comparison to microplastics, which are currently unregulated, using a Niton XL3t GOLDD+ Portable XRF analyzer.
Distance to roads and slope of fields were determined using ArcMap (version 10.8.0.12790). The slope of fields was determined using SRTM (Shuttle Radar Topography Mission, USGS) Digital Elevation data at 30 m resolution converted to slope using the Slope (Spatial Analyst) tool. The mean slope was determined within the field boundaries of each site using the Zonal statistics (Spatial Analyst) tool. Distance to roads was determined using the Generate Near Table (Analysis) tool to measure the minimum distance of a field to a major road based on the edge of field boundaries and open roads data (Ordnance Survey open roads, November 2021).
2.4 Sample processing
The microplastic extraction method was selected based on the soil characteristics according to Radford et al. (35). Samples were oven dried at 50°C for 7 days to obtain dry weight of soils. As the soil samples contained a relatively small percentage of organic matter, oil extraction was selected as a density separation technique to remove the inorganic fraction of the soils. A sub-sample of homogenized dry soil was taken and weighed for extraction (ranging from 14.4 to 35.1g D.W.). Sub-samples were placed into 250mL glass beakers, 50mL of MiliQ water was added and left to stand for 1 hour to allow soil dispersion and aid in breaking up soil aggregates. An aliquot of 10mL of canola oil was added to each sample and mixed with a stainless-steel spoon for 30 seconds to break up any agglomerates and ensure oil dispersion throughout the sample. Residues on the spoon were rinsed back into the sample with additional water. Each beaker was then filled up with water, leaving a 1 cm gap to the top of the beaker, covered with aluminum foil, and left overnight to for the inorganic particles to settle. The top layer of oil, containing organics and separated microplastics, was poured into a smaller 150 mL glass beaker, which was covered in aluminum foil and set aside. A second round of oil extraction was performed to maximize extraction efficiency by adding another 10 mL of canola oil and mixed again with a stainless-steel spoon for 30 seconds. Beakers were then filled up with water and left overnight as before. Again, the top layer of oil was poured into the same 150 mL beaker as previously to combine the two rounds of oil extraction. The entire contents of the 150 mL beaker were filtered using a vacuum pump over a 25 µm stainless steel filter to remove the oil fraction. The filter was placed into a 100 mL glass beaker and the filtering apparatus was rinsed with MiliQ water to collect any residues on the glassware.
In a fume cupboard, 30 mL of hydrogen peroxide (30% v/v) was then added to the samples, which were covered in aluminum foil and placed in a shaking incubator at 50°C, 100rpm overnight. The same 25 µm stainless steel filters were then rinsed off into the beaker using water and used to filter the contents of the beaker again using a vacuum pump to remove all remaining hydrogen peroxide. This time, the residues of the filter were rinsed, using minimal water, back into the beaker and the filter discarded. Each sample was then topped up with 30 mL of Decon90, chosen to remove any remaining oil residues that would impede later identification methods (36). After 48 hours, the Decon90 was filtered out using a vacuum pump over a 25 µm stainless steel filter and rinsed with water until no bubble formation occurred. The residues were separated out by size using 1 mm stainless steel mesh and rinsed with ethanol (50% v/v) into a 20 mL glass vial for storage, one containing >1 mm particles and one with <1 mm. Only small microplastics (<1 mm) were analyzed and will hereafter be referred to as microplastics.
2.5 Polymer identification
Polymers were identified using automated μFourier-transform infrared spectroscopy (FTIR) (PerkinElmer Spotlight 400). Sub-samples of processed soils were taken to control quality of filters and ensure overloading did not reduce spectrum quality. Filter areas were limited using a silicone washer (8 mm diameter), placed on to a silver filter on a vacuum filter set up. The <1mm vial for each sample was well mixed by pipetting up and down in the vial using a glass 10 mL pipette, before pipetting a subsample onto the silver filter (3 μm pore size, Sterlitech, Washington USA). The quantity of subsample was determined visually based on the amount of residual particles present in the initial sample and the subsequent amount of particles on the filter. This was quantified by weighing the vial before and after to determine the weight (and thus volume) of the subsample as per (13).
Filters were left to dry in a glass petri dish at room temperature for at least 24 hours prior to scanning. An area of 8.5 x 8.5 mm was scanned for each sample to cover the filter area. The filter was scanned with 2 × 8 linear arrays in reflectance mode with 2 scans per pixel at a pixel resolution of 25 μm and spectral resolution of 8 cm−1 in the range of 4000–700 cm−1. A background spectrum was collected on a clean space of the silver filter with the same settings at 90 scans per pixel prior to each analysis.
Spectral maps were processed and analyzed using siMPle software (37; available at www.siMPle-plastics.eu). The Aalborg University pipeline using raw, and first derivatives was used, with a minimum particle size of one pixel (25 µm). Particles were identified using the siMPle automated IR database (version 1.0.1) and classified by size and mass (as an estimate based on particle volume, polymer density, and an assumed ellipsoid 3-dimensional shape). Results are reported at microplastics per kilogram (MP/kg).
2.6 Quality control
Stringent quality control measures were taken throughout the experiment. In the field, only metal and wooden sampling equipment was used, and clothing was limited to natural fibers where possible during sample collection, processing, treatment, and analysis. In the laboratory, extractions were carried out in an ISO-5 clean laboratory and in a laminar flow cabinet (Felcon), where non-shreddable Tyvex suits (Dupont, IsoClean) were worn at all times, with the exception of digestions which were carried out in a separate laboratory in a fume hood wearing a cotton lab coat, for the purpose of health and safety. All processing equipment was glass or metal. Metal equipment (i.e., stainless steel spoons and aluminum foil) was furnaced at 500°C for 9 hours and all glassware was acid washed and rinsed thoroughly with Mili-Q water prior to use. All reagents were filtered prior to use over a GF/C glass-fiber filters (1.2 μm, Whatman GF-C) and all water used was Milli-Q. PTFE wash bottles were used to dispense Milli-Q and ethanol where required. All stainless steel and GF/C filters were furnaced at 500°C for 9 hours prior to use to remove any particulate contaminants. Sample analysis using FTIR was conducted in a separate laboratory where cotton lab coats were worn. The FTIR microscope was encased with a Spotlight atmospheric enclosure made of Plexiglas to limit atmospheric contamination. Ten procedural blanks were conducted alongside the samples using the same methods for extraction identification methods. These were used to correct all data for procedural contamination using limit of detection (LOD) values. The LOD value for each was calculated as 3.3 times the standard deviation and accounted for based on individual polymers (13).
Additionally, spiked samples were processed as positive controls to determine recovery efficiency of the microplastic extraction method. A stock solution was created using known concentrations of four types of microplastic fragments: PET (66 MP/mL, size: 34- 149 μm), PE (8 MP/mL, size: 30- 96 μm), PP (711 MP/mL, size: 66-140 μm), PVC (73 MP/mL, size: 99- 333 μm), dispersed in MiliQ water. Six replicates of one soil sample were spiked with this stock solution and were processed as per the soil extraction and identification method. Recovery rate was then calculated as a percentage of the concentrations of each microplastic type added to the sample.
2.7 Statistical analysis
All statistical analyses were conducted in R Studio (1.4.1106). Microplastic count and mass data were blank corrected using LOD values as per Horton et al. (13), whereby only data greater than the average + 3.3 SD of the blank samples were reported. Where required, data were checked for normality using Shapiro Wilk tests. Mixed models were used to analyze differences in microplastics counts, weights and average size across biosolid treatments and seasons. Data were converted to integers and a GLMM (Generalized Linear Mixed Model) was fitted to a Poisson distribution and log link. Field replicates were nested in each sampled field as a random effect to account for repeated measurements of the same field. All models included the two-way interaction between biosolid application and season, and Tukey’s post hoc tests were used to determine differences across treatments. Data were transformed when required to ensure model fit– mass data were cubed, and average size was square rooted. Residual distributions were checked to assess model fit.
For individual polymers, data were transformed to binary (presence or absence of polymer types) and a GLMM was fitted with a binary distribution. Again, field replicates were nested in field ID as a random and a two-way interaction between biosolid application and season was included. Shannon Diversity index was calculated for each field on each sampling occasion to determine polymer diversity (38). An average across the four replicates was taken per field and differences across treatments and sampling occasions were calculated using Kruskal Wallis tests as the data did not meet the requirements for parametric assessments. Additionally, a principal component analysis was applied to determine which polymers best accounted for the variability between treatments and seasons.
Co-variates were accounted for separately to rule out the influence of soil characteristics, including particle size distribution (as % clay particles), organic matter and metal content which were analyzed using a 2-way analysis of variance (ANOVA) or Kruskal-Wallis Rank Sum tests, depending on data distribution, to determine differences between seasons and treatments. Distance to roads and slope of field were analyzed using a Mann Whitney-U test and T-test, respectively, to determine differences between biosolid treatment groups. Factors that were not significant between treatments were excluded from the models to improve model accuracy.
3 Results
3.1 Quality control
There was minimal contamination within the blanks with a mean of 2.5 microplastics per sample. Five polymer types were found across the blank samples which were ‘Acrylates, Polyurethanes, and varnishes’ (APV), cellulose, ‘Ethylene-Vinyl-Acetate’ (EVA), polyester, and polypropylene. Polyester was the most prevalent with a mean of 1.5 microplastics per sample whereas cellulose was only found in one blank sample. The LOD (3.3 x the SD of the blank samples) for individual polymers therefore ranged from 1 to 10.11 microplastics per sample meaning that, for microplastics to be detected, quantities within one sample vial needed to exceed these values for individual polymers. The average recovery of microplastics across the four types of spiked microplastics was 42%.
3.2 Covariates
Covariate measurements are shown in Table 1. There was no difference in the amount of organic matter in the soils between the biosolid treatments or seasons (F (1, 16) =0.47, p = 0.505), the minimum organic matter content was 3.6% and the maximum was 8.9%. The particle size distribution (% clay particles, <0.4µm) did not vary between seasons or biosolid treatments (W=74, p=0.075; W=54, p=0.796, Mann Whitney-U tests) and ranged from 1.07 to 4.99%. Additionally, there were no differences in soil metal contents between biosolid treatments or seasons (zinc: seasons W=56.5, p=0.646, biosolid treatments W=29.5, p=0.126; copper: seasons W=66.5, p=0.197, biosolid treatments W=57.0, p=0.600) and nickel was below the LOD for all samples. Zinc concentrations ranged from 60 to 170 ppm across all samples while copper ranged from 0 to 40 ppm. The mean distance of sampling sites to roads was 33.78m and there was no difference between biosolid treatments (W=16, p=0.548, Mann Whitney-U test). The mean slope of sampling fields was 2.48° and there were no differences across groups (t (7.83) = -0.0837, p-0.935, T-test).
3.3 Microplastic quantities
Microplastics were found in all ten of the fields sampled on at least one occasion. There were only two instances where no plastic was found in fields, both on the summer sampling occasion. The highest number of microplastics was found in one of the biosolid treated fields with a mean of 1486 ( ± 1064 SE) MP/kg across the two sampling occasions. While another biosolid treated field had the lowest number of microplastics across the two sampling occasions with a mean of 202 ( ± 87 SE) MP/kg (Figure 2).
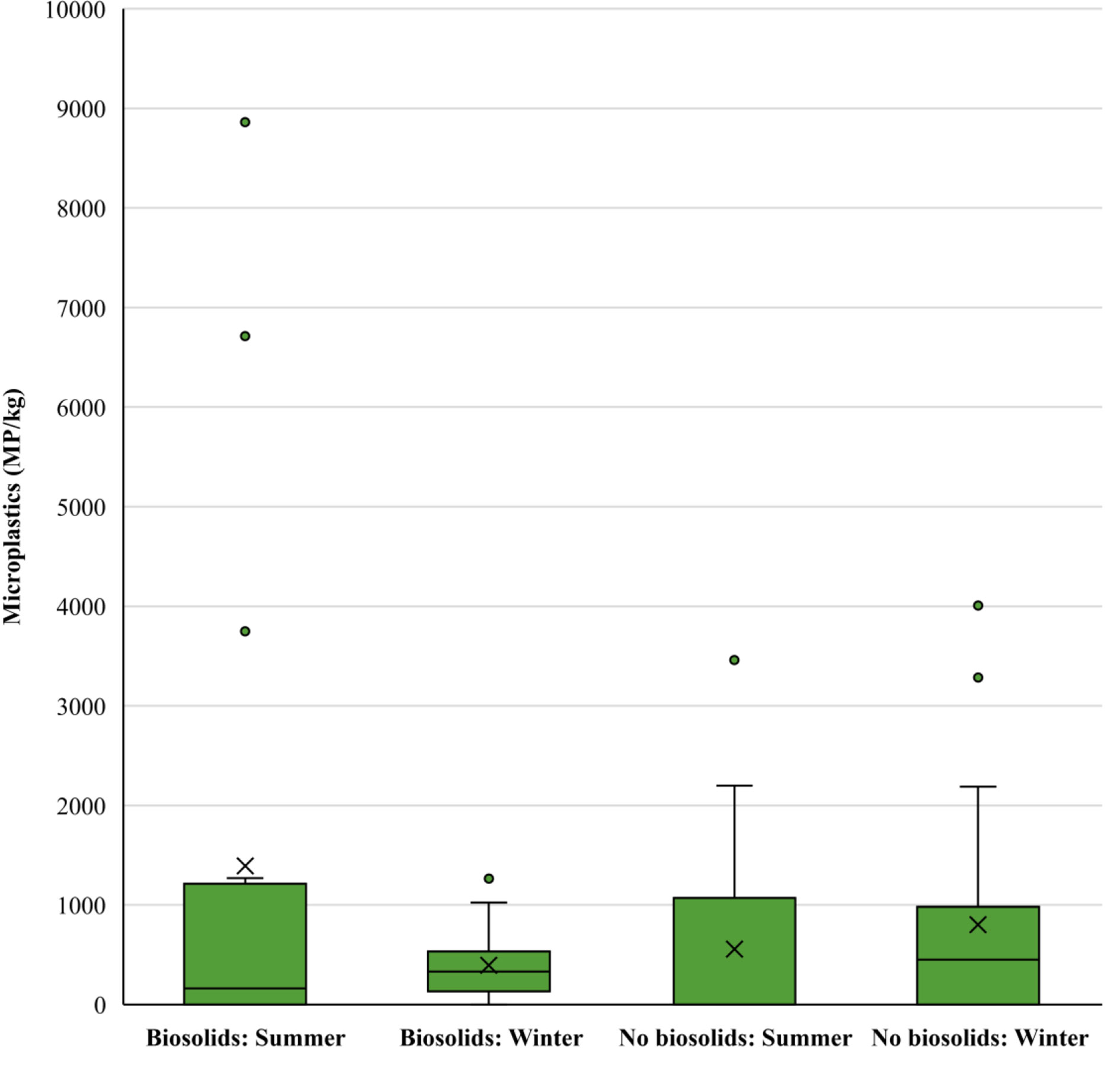
Figure 2 Microplastic concentrations in soils with and without biosolid application during summer (August 2019) and winter (February 2020) months shown as minimum, lower quartile, median, upper quartile and maximum values. Dots show values outside the interquartile range.
There were no differences in the overall number of microplastics in biosolid treated and untreated soils with means of 892( ± 289 SE) and 679 ( ± 165 SE) MP/kg, respectively (χ2 (1) = 0.68, p = 0.411). The same was true for the mean size of microplastics, which was 605.1( ± 423 SE) µm in the untreated soils and 291.2( ± 92 SE) µm the treated soils (χ2 (1) = 0.597, p = 0.441), and the mass of microplastics with means of 206846( ± 204162 SE) and 86612( ± 854814 SE) µg/kg, in the treated and untreated soils respectively (χ2 (1) = 0.013, p = 0.971).
Significant differences were evident in microplastic quantities and characteristics between summer and winter. There were more microplastics overall in the samples taken in the summer with a mean of 973( ± 302 SE) MP/kg compared with a winter with a mean of 579( ± 134 SE) MP/kg (χ2 (1) = 2232.04, p < 0.001). The soils treated with biosolids followed this trend and had significantly more microplastics in the summer (1391 ± 557 SE MP/kg) than the winter (394( ± 79 SE) MP/kg; Tukey Test: z =-98.88, p<0.001). However, the soils without biosolid treatment had significantly more plastic in the winter than the summer (Tukey Test: z = 29.87, p <0.001) with means of 803( ± 251 SE) and 556( ± 216 SE) MP/kg, respectively. Additionally, the size of microplastics was significantly larger overall in the winter where the mean was 482.4( ± 297.5 SE) µm compared to summer where the mean size was 338.7( ± 126.2 SE) µm (χ2 (1) = 441.32, p < 0.001). However, specifically within the treated soils the mean the mean microplastic size was smaller in the winter, with a mean of 179.1(± 58.9 SE) µm, compared to the summer with a mean of 481.7( ± 220.1 SE) µm (Tukey Test: z = 4.24, p <0.001; Figure 3).
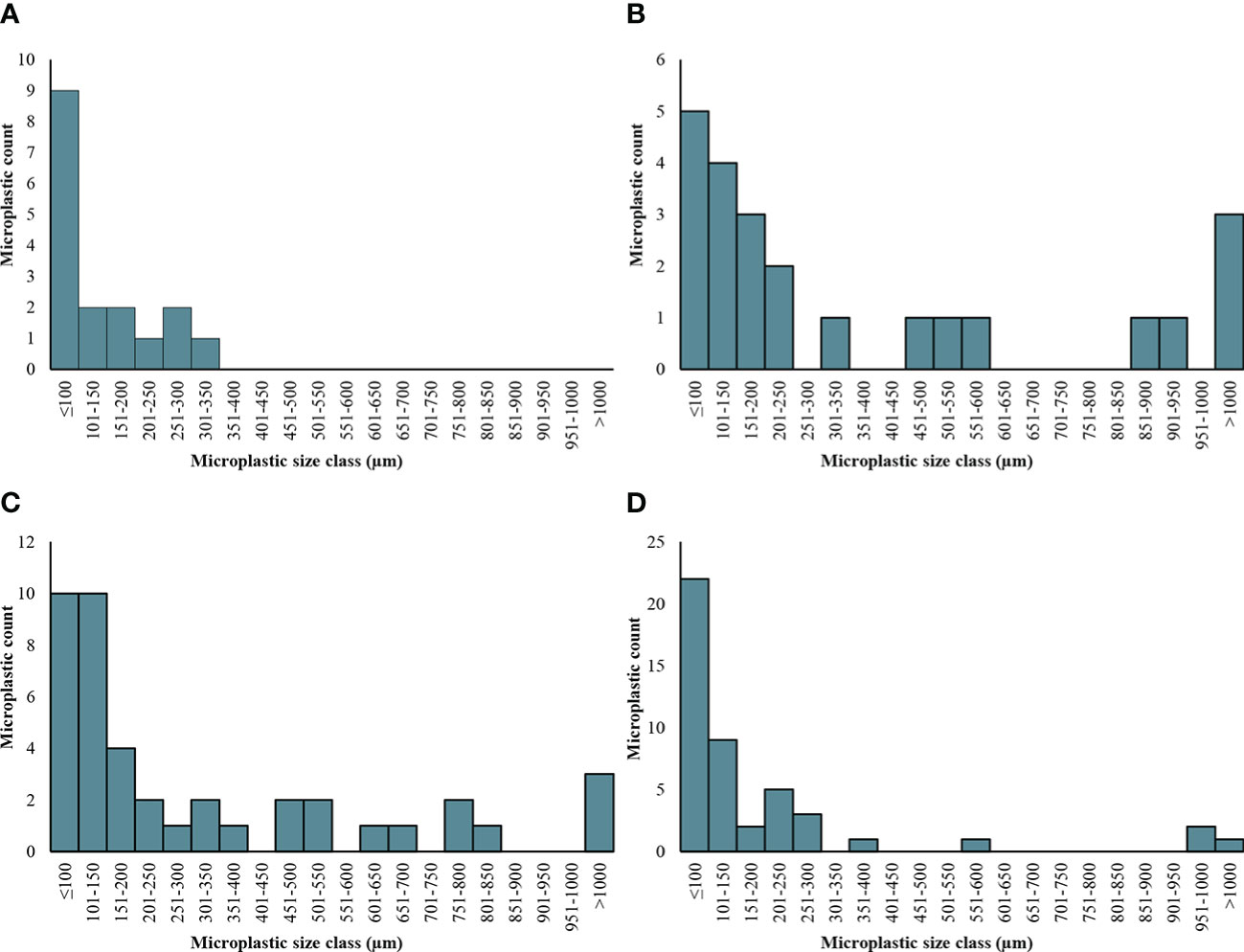
Figure 3 Size categories for microplastics found in soils with and without biosolid treatment in summer and winter [(A). No biosolids: summer; (B) Biosolids: summer; (C) No biosolids: winter; (D) Biosolids: winter]. Microplastic count represents the raw data and have not been blank corrected.
Overall, there was a higher mass of microplastics in the summer than the winter sampling occasion (χ2 (1) = 3.95, p=0.045). This overall difference was driven by the significantly higher mass of plastic in the summer month in the treated soils which had a mean of 412130( ± 408265 SE) µg/kg compared to the untreated which had a mean of 230( ± 112 SE) µg/kg (Tukey Test: z = - 2.98, p 0.011). When considering the treated vs untreated soils separately there was a higher mass of microplastics in the summer for treated soils (Tukey Test: z = -10.51, p <0.001). However, in line with the differences seen in microplastic numbers, there was more plastic, by mass, in the untreated soils in the winter than the summer (Tukey Test: z = 10.48, p <0.001)
3.4 Microplastic composition
Ten different polymer types were identified across the samples (Figure 4). The most common polymers were polypropylene and EVA. Polypropylene was found in 24 of the total 80 samples with an average of 122( ± 29 SE) MP/kg, whereas EVA was only found in 20 samples but in higher concentrations with a mean of 396( ± 150 SE) MP/kg. Nine of the polymer types were found in biosolid treated soils, whereas only 7 were found in the untreated soils. Polyethylene, chlorinated polyethylene, and polystyrene were the least common polymers found and were only present in the biosolid treated soils. Polyester was only found in the untreated soils. With the exception of polypropylene and cellulose, there were no significant differences in individual polymer types across the treatments and seasons. Polypropylene was found in more frequently in the winter months, occurring in all 10 of the sampling locations, whereas in the summer it was only found in four (χ2 (1) = 12.41, p <0.001). Similarly, cellulose was found in eight of the fields in winter but only three in the summer (χ2 (1) = 4.05, p 0.044).
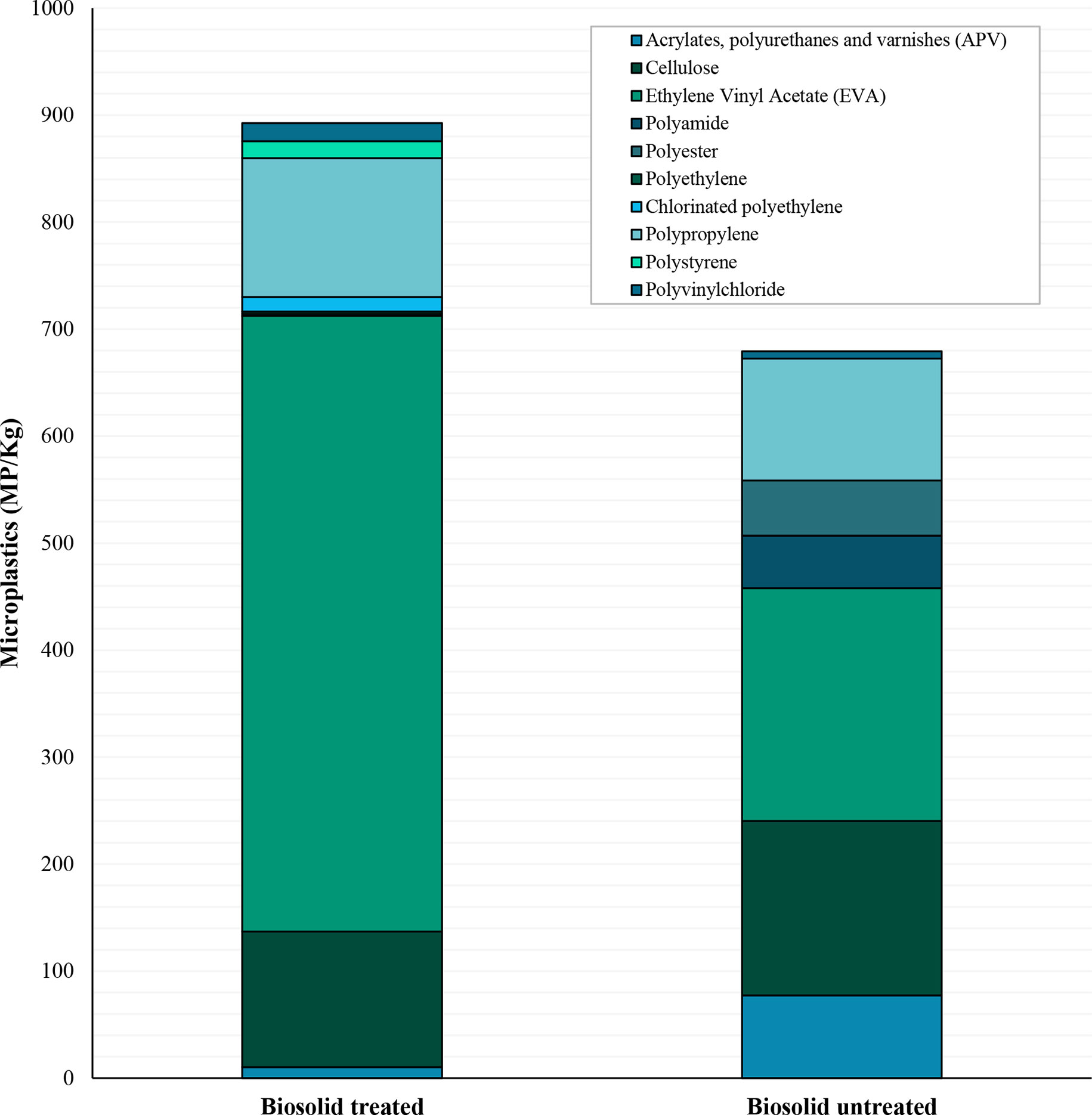
Figure 4 Polymer types of microplastics in soils with and without biosolid treatment. Counts are reported as number of microplastics of each polymer type per kilogram of soil and have been blank corrected using calculated LOD’s.
Diversity indices for polymers were very low across sites (mean 0.20 ± 0.04). Samples had uneven polymer distribution in terms of relative abundance of individual polymers and this diversity did not vary across treatments (χ2 (1) = 0.74, p =0.389, Kruskal Wallis test). However, polymer diversity was higher in the winter months with a mean of 0.29 (χ2 (1) = 6.90, p= 0.009, Kruskal Wallis test). Polyester, polyethylene, chlorinated polyethylene, and polystyrene were excluded from PCA analysis as they all only occurred once across all samples. The first principal component (PC1) explained 23.0% of the variance. Polypropylene, cellulose, and polyamide were all negatively correlated with this axis and made up the majority of the loadings. Principal component 2 (PC2) explained a further 17.9% of the variation in these data and was largely made up of APV and PVC. APV was negatively correlated with this axis and PVC was positively correlated. The untreated winter soils had the least amount of variation along this axis, suggesting low numbers of PVC and APV. Soils from different treatments and seasons cannot be clearly distinguished from each other based on polymer composition. Of the biosolid treated soils, in the summer PC2 looked to increase with PC1 whereas in the winter this was reversed and PC2 decreased with increasing PC1. However, there was an overall lack of differentiation between the composition of microplastics across treatments and seasons suggesting they cannot be clearly distinguished from each other based on polymer composition.
4 Discussion
The observations of this study suggest that biosolids are not the only source of microplastics in the agricultural soils investigated. While the mean number of microplastics was slightly higher in the biosolid treated fields (874 MP/kg), than the untreated fields (664 MP/kg), there were no significant differences found in the number of microplastics between the two groups. Despite this, overall concentrations of microplastics were relatively high, with a maximum of 1461 MP/kg, and were found across all the sampled fields on at least one occasion. These results correspond with observations recorded elsewhere. Biosolid amended soils in Chile were found to have microplastic concentrations in the range of 600 to 10,400 MP/kg (20). In contrast, lower values were found in central Spain where soils recently treated with biosolids had microplastic concentrations ranging from 138 to 288 MP/kg, and 31 to 120 MP/kg in control soils that had not received biosolids (21). Other studies indicate that biosolid application is a main driver of soil microplastic concentrations (20), however, the lack of difference between biosolid treated and untreated fields here suggest that they are not solely responsible for microplastic contamination in agricultural soils.
There is no doubt that biosolids contain extremely high quantities of microplastics as this has been reported on multiple occasions. Reports of microplastic concentrations in biosolids vary between studies but have been reported in the millions (per kg D.W.) in several studies with a highest value of 10,380,000 MP/kg reported to date (13, 15, 16). Despite these high numbers, overall indications from the present and previous studies are that numbers of microplastics are generally similar between biosolid-treated and untreated soils, although within both groups there is a great deal of variation within the data (24). For example, Crossman et al. (8), tested soils from three fields and showed that there was an increase in microplastic concentrations following biosolid application for two sites but not the third. This high variation suggests that there are other factors that contribute to the overall microplastic concentrations in soils.
This lack in difference found in microplastic concentrations between the two groups may have several explanations. Firstly, whilst the main aim of this study was to determine the influence of biosolids as a source, there are likely other sources of plastic inputs in these sites. These other sources may input at varying scales, meaning that fields without biosolid treatment may be exposed to high numbers of microplastics. One of the main additional sources to consider are agricultural plastics. Although sites with plastic mulching were avoided, other agricultural items such as plastic films (including silage wrap), nets, irrigation pipe, fertilizers sacks, pesticide cans (39) and polymer seed coatings (40) may be contributing. The most common polymer types found in this study were polypropylene and EVA. Polypropylene has been frequently found in both biosolids and soils (13, 33, 41); however, it is also often used in agricultural packaging, piping, sheeting, nets, and twines (39) and more niche uses such as tree guards which are often found in field margins (42). Additionally, EVA is a copolymer frequently used in agricultural films and has previously been reported in soils that have not received biosolid amendments (5). The lack of differentiation in polymer diversity between treatments also suggests that there is more to consider than the biosolid application. Although there were three polymer types which were only found in the biosolid treated soils (polyethylene, chlorinated polyethylene, and polystyrene), these polymers only occurred once across all samples suggesting that they are rare and therefore harder to sample and account for (43).
Another source that may be considered is atmospheric deposition, which has shown to account for 136.5 to 512.0 MP/m2/day, even in rural areas (44). This deposition will depend on wind direction and speeds (45) and is suggested to be responsible for horizontal migration of microplastics across geographical locations, even with the possibility of the microplastic load in biosolid treated soils to be distributed to non-treated areas (46). This deposition is likely to be impacted by proximity to urban areas (47) and distance to main roads (48). So, given that all sampled fields were within proximity to roads (<300m) there is high potential for microplastics to be entering these soils through such sources. Additionally, patterns of microplastic patchiness were shown in the present study, evident by the high variation in microplastic concentrations within sampled fields. This is a potential sign of fragmentation of larger plastic debris (32). Secondary sources such as the breakdown of larger plastic litter may contribute (49). The varied polymer composition and diversity found across all sites in the present study suggest a range of sources may be contributing to the overall microplastic contamination. It remains challenging to determine the exact origin of these microplastics, particularly from diffuse sources that are harder to identify (50).
In addition, the variation in the data may be attributable to individual farm management. Whilst all efforts were taken in this study to factor out influencing variables (crop rotations, cultivation methods, use of plasticulture), agricultural practices are complex and often farm-specific. Ploughing frequency and intensity, crop types and the use of fertilizers (inorganic and other organic e.g. animal slurry) may all impact the amount and distribution of microplastics (32). Although not considered in this study, the rate and frequency of biosolid application may also have an impact. Soils with historic biosolid treatment, as opposed to a recent one-off application, may have higher microplastic concentrations due to cumulative inputs (20, 21). The types of biosolids and associated methods of application may also have an impact on the overall microplastic distribution (51). For example, with the soils sampled here, spraying of sludge cake was implemented which generally leaves non-uniform distribution of biosolids and consequently the contained microplastics (see Supplementary Information, Figure S3). This may explain the patchiness in microplastic concentration and composition within the sampled fields.
Additionally, ploughing and tillage activity may influence microplastic behaviour in soils by further fragmenting plastics and incorporating them into the soil (22). As is common in modern UK farming practices, all farms sampled here used a minimum tillage approach, where only the top layer (<10cm) of soil is turned over. This approach has the potential to reduce the retention of microplastics in soils, particularly at depth, as water infiltration is reduced (52), suggesting the greater potential for microplastics to be washed off via surface runoff.
The outputs of materials from such agricultural soil systems may influence the number of microplastics found. It is known that large amounts microplastics are being applied in biosolids to these soils, but as this was not reflected in the soil microplastic concentrations, it suggests many of these microplastics are not staying where they are applied. Samples taken are a snapshot in time and outputs due to erosion, runoff and wind transport should be taken into account when considering overall microplastic concentrations, which may not be retained indefinitely. This was indicated by the difference in microplastic numbers between summer and winter samples. During the month of the summer sampling occasion, the total rainfall was 51 mm, and a mean of 967 MP/kg was found across all sites, whereas in the winter month, rainfall was much higher (122 mm) and the mean microplastic concentration was lower at 571 MP/kg. Rainfall and resultant surface runoff may cause microplastics to be removed from the soils (53). Previous studies have shown similar patterns of lower microplastic concentrations, with losses of 30-45% from soils, following heavy rainfall events (8). In addition, the resulting erosion of agricultural land is seasonal, with increased erosion prevalent in winter months (54). This will depend on the soil type as more dense soils are more susceptible to runoff and will therefore likely retain less plastic (8). The mean microplastic size found here was larger in the winter suggesting that smaller microplastics may be more susceptible to transport out of soils subjected to higher rainfall. This transport is also dependent on crop cover, as bare soils become saturated quickly and generate increased runoff. Given that crop cover is generally lower in the winter months after summer crops are harvested, this may also be influencing the output of microplastics from these soils. However, in the untreated soils there were more microplastics in the winter and significantly higher, although still relatively low, polymer diversity. This highlights the complexity of these systems and suggests the influence of external factors, for example different types of vegetation cover may influence microplastic concentrations and distributions in different ways (55). Moreover, inputs of microplastics from atmospheric deposition have been shown to increase during periods of high rainfall as a result of scavenging of particles into water droplets (56). This process may be particularly pronounced for light density polymers such as polypropylene, which was found more frequently in the winter month, that are more likely to be transported by wind. In addition, some reports suggest that surface runoff only contributes to a small proportion of mobilising microplastics (21), therefore this may depend on the polymer type, size, and morphology of the plastics, and requires further investigation. The influence of biota must also be considered as it has been shown that soil invertebrates such as earthworms are likely to incorporate microplastics into the soil (57). Similarly microbial communities have been shown to increase microplastic degradation in soils, particularly for biodegradable polymers (58).
Whilst the sampling strategy in this study aimed to gather representative samples across fields, it is possible that soil heterogeneity at field-scale may impact microplastic concentrations. Future studies may further consider this in relation to individual field and soil characteristics (e.g., slopes, presence of and proximity to hedgerows, soil grain size and density), especially as soil texture and organic carbon have been shown to influence the retention and transport of organic pollutants (59). It is important to consider that soils are a complex medium for which the development of microplastic extraction methods are currently in progress (35). Given that the spiked recoveries were generally low (42%), the values reported here may be an underestimate- in line with microplastic extraction methods generally which are estimated to be underestimated by 14% across all environmental media (60). Additionally, while the use of μFTIR allows for rapid detection of small microplastics, it is limited to the aperture size (10–20 μm) (61) and is therefore likely to underestimate fibres which generally have a diameter below this limit.
We now know that there are microplastics in agricultural soils at appreciable levels, but to reduce or prevent the input of these plastics we must first elucidate their sources. Additionally, it is important to understand further the behaviour of microplastics in soils, and their fate once they are transported out of agricultural systems; farm fields may act as a sink and a pathway for microplastic to move through the environment. In particular, this may have implications for surrounding waterbodies that may receive the exported microplastics. In a local context, the River Test estuary downstream of agricultural areas has been shown to have high numbers of microplastics present in the water (28) to which agricultural soils may be contributing. Future studies should focus on how microplastics move through terrestrial systems, ideally at a catchment level (25). Simultaneously, we need to know what impact these microplastics are having in soils, including effects on soil fauna, food security and crop yields (62).
5 Conclusions
Understanding the sources of microplastic contamination in soils is imperative for future mitigation strategies to be effective. Overall, this study suggests that biosolids, whilst are likely a contributor, are not the sole source of microplastics in agricultural soils. The variability in results seen here highlights the complexity of determining microplastic concentrations in heterogeneous agricultural soils’ and given the variety of microplastic types found here suggests that multiple sources may be contributing. Additionally, the difference in microplastic concentrations between seasons here suggests that the dynamic nature of the agricultural soil environment may result in soils being a vector for microplastics into the wider environment, so further research is required to determine source and sink dynamics.
Data availability statement
The raw data supporting the conclusions of this article will be made available by the authors, without undue reservation.
Author contributions
FR contributed to conceptualization, methodology, formal analysis, investigation and, writing. AH, MH, PS and IW contributed to conceptualization, review, editing, and supervision. All authors contributed to the article and approved the submitted version.
Funding
This work was supported by Engineering and Physical Sciences Research Council funded Centre for Doctoral Training in Sustainable Infrastructure Systems (‘Managing emerging pollutants in wastewater systems’, grant number EP/L01582X/1), and Southern Water.
Acknowledgments
The authors would like to thank Harold Makant of Natural England for assisting with fining study sites and the landowners for their cooperation with soil sampling throughout the study.
Conflict of interest
The authors declare that the research was conducted in the absence of any commercial or financial relationships that could be construed as a potential conflict of interest.
The authors declare that this study received funding from Southern Water. The funder was not involved in the study design, collection, analysis, interpretation of data, the writing of this article, or the decision to submit it for publication.
Publisher’s note
All claims expressed in this article are solely those of the authors and do not necessarily represent those of their affiliated organizations, or those of the publisher, the editors and the reviewers. Any product that may be evaluated in this article, or claim that may be made by its manufacturer, is not guaranteed or endorsed by the publisher.
Supplementary material
The Supplementary Material for this article can be found online at: https://www.frontiersin.org/articles/10.3389/fsoil.2022.941837/full#supplementary-material
References
1. Plastics Europe. Plastics the facts 2021 (2021). Available at: https://plasticseurope.org/knowledge-hub/plastics-the-facts-2021/ (Accessed 21 April 2022).
2. Andrady AL. Microplastics in the marine environment. Mar pollut Bull (2011) 62(8):1596–605. doi: 10.1016/j.marpolbul.2011.05.030
3. de Souza Machado AA, Lau CW, Kloas W, Bergmann J, Bachelier JB, Faltin E, et al. “Microplastics can change soil properties and affect plant performance,” Environ Sci Technol (2019) 53(10):6044–52. doi: 10.1021/acs.est.9b01339
4. Zhu F, Zhu C, Wang C, Gu C. Occurrence and ecological impacts of microplastics in soil systems: A review. Bull Environ Contamination Toxicol (2019) 102:741–9. doi: 10.1007/s00128-019-02623-z
5. Corradini F, Casado F, Leiva V, Huerta-Lwanga E, Geissen V. Microplastics occurrence and frequency in soils under different land uses on a regional scale. Sci Total Environ (2021) 752:141917. doi: 10.1016/j.scitotenv.2020.141917
6. Watteau F, Dignac MF, Bouchard A, Revallier A, Houot S. Microplastic detection in soil amended with municipal solid waste composts as revealed by transmission electronic microscopy and Pyrolysis/GC/MS. Front Sustain Food Syst (2018) 2:2571–581X. doi: 10.3389/fsufs.2018.00081
7. Yang J, Li R, Zhou Q, Li L, Li Y, Tu C, et al. Abundance and morphology of microplastics in an agricultural soil following long-term repeated application of pig manure. Environ pollut (2021) 272:116028. doi: 10.1016/j.envpol.2020.116028
8. Crossman J, Hurley RR, Futter M, Nizzetto L. Transfer and transport of microplastics from biosolids to agricultural soils and the wider environment. Sci Total Environ (2020) 724:138334. doi: 10.1016/j.scitotenv.2020.138334
9. Collivignarelli MC, Abbà A, Frattarola A, Miino MC, Padovani S, Katsoyiannis I, et al. Legislation for the reuse of biosolids on agricultural land in Europe: Overview. Sustainabil (Switzerland) (2019) 11(21):6015. MDPI. doi: 10.3390/su11216015
10. Racek J, Sevcik J, Chorazy T, Kucerik J, Hlavinek P. Biochar – recovery material from pyrolysis of sewage sludge: A review. Waste Biomass Valorization (2020) 11:3677–709. doi: 10.1007/s12649-019-00679-w
11. Liu J, Liu S, Smith SR. A contemporary and historical analysis of the trace element composition of sewage sludge in the United Kingdom. Water Environ J (2021) 35(3):892–901. doi: 10.1111/wej.12677
12. Mohajerani A, Karabatak B. Microplastics and pollutants in biosolids have contaminated agricultural soils: An analytical study and a proposal to cease the use of biosolids in farmlands and utilise them in sustainable bricks. Waste Manage (2020) 107:252–65. doi: 10.1016/j.wasman.2020.04.021
13. Horton AA, Cross RK, Read DS, Jürgens MD, Ball HL, Svendsen C, et al. Semi-automated analysis of microplastics in complex wastewater samples. Environ pollut (2021) 268(A):115841. doi: 10.1016/j.envpol.2020.115841
14. Okoffo ED, Donner E, McGrath SP, Tscharke BJ, O’Brien JW, O’Brien S, et al. Plastics in biosolids from 1950 to 2016: A function of global plastic production and consumption. Water Res (2021) 201:117367. doi: 10.1016/j.watres.2021.117367
15. Cunsolo S, Williams J, Hale M, Read DS, Couceiro F. Optimising sample preparation for FTIR-based microplastic analysis in wastewater and sludge samples: multiple digestions. Anal Bioanal Chem (2021) 413:3789–99. doi: 10.1007/s00216-021-03331-6
16. Salmi P, Ryymin K, Karjalainen AK, Mikola A, Uurasjärvi E, Talvitie J. Particle balance and return loops for microplastics in a tertiary-level wastewater treatment plant. Water Sci Technol (2021) 84(1):89–100. doi: 10.2166/wst.2021.209
17. Napper IE, Thompson RC. Release of synthetic microplastic plastic fibres from domestic washing machines: effects of fabric type and washing conditions. Mar pollut Bull (2016) 112(1–2):39–45. doi: 10.1016/j.marpolbul.2016.09.025
18. Ngo PL, Pramanik BK, Shah K, Roychand R. Pathway, classification and removal efficiency of microplastics in wastewater treatment plants. Environ pollut (2019) 255:113326. doi: 10.1016/j.envpol.2019.113326
19. Ofwat. Water 2020: Regulatory framework for wholesale markets and the 2019 price review (2015). Available at: https://www.ofwat.gov.uk/consultation/water-2020-consultation/ (Accessed 21 April 2022).
20. Corradini F, Meza P, Eguiluz R, Casado F, Huerta-Lwanga E, Geissen V. Evidence of microplastic accumulation in agricultural soils from sewage sludge disposal. Sci Total Environ (2019) 671:411–20. doi: 10.1016/j.scitotenv.2019.03.368
21. Schell T, Hurley R, Buenaventura NT, Mauri P. V., Nizzetto L, Rico A, et al. Fate of microplastics in agricultural soils amended with sewage sludge: Is surface water runoff a relevant environmental pathway? Environ pollut (2022) 293:118520. doi: 10.1016/j.envpol.2021.118520
22. van den Berg P, Huerta-Lwanga E, Corradini F, Geissen V. Sewage sludge application as a vehicle for microplastics in eastern Spanish agricultural soils. Environ pollut (2020) 261:114198. doi: 10.1016/j.envpol.2020.114198
23. Nizzetto L, Futter M, Langaas S. Are agricultural soils dumps for microplastics of urban origin?” (2016). Environ Sci Technol (2016) 50(20):10777–9. doi: 10.1021/acs.est.6b04140
24. Ziajahromi S, Leusch FDL. Systematic assessment of data quality and quality assurance/quality control (QA/QC) of current research on microplastics in biosolids and agricultural soils. Environ pollut (2022) 294:118629. doi: 10.1016/j.envpol.2021.118629
25. Windsor FM, Durance I, Horton AA, Thompson RC, Tyler CR, Ormerod SJ. A catchment-scale perspective of plastic pollution. Global Change Biol (2019) 25(4):1207–21. doi: 10.1111/gcb.14572
26. Homewood JM, Purdie DA, Shaw PJ. Influence of sewage inputs and fish farm effluents on dissolved nitrogen species in a chalk river. Water Air Soil Pollut: Focus (2005) 4(6):117–25. doi: 10.1007/s11267-005-3021-0
27. Moore A, Ives S, Mead TA, Talks L. The migratory behaviour of wild Atlantic salmon (Salmo salar l.) smolts in the river test and Southampton water, southern England. In: Advances in invertebrates and fish telemetry. (Dordrecht, Netherlands: Springer) (1998). p. 295–304.
28. Gallagher A, Rees A, Rowe R, Stevens J, Wright P. Microplastics in the solent estuarine complex, UK: An initial assessment. Mar pollut Bull (2016) 102(2):243–9. doi: 10.1016/j.marpolbul.2015.04.002
29. National Rivers Authority. “River test catchment management plan: phase 1.” technical report. Bristol: NRA (1991).
30. Cranfield University. The soils guid (2020). Available at: https://www.cranfield.ac.uk/themes/environment-and-agrifood/landis/soils-guide (Accessed on 21 April 2022).
31. Environment Agency. The national archives, weekly rainfall and river flow reports for England (2020). Available at: https://www.gov.uk/government/publications/weekly-rainfall-and-river-flow-reports-for-england (Accessed 21 April 2022).
32. Harms IK, Diekötter T, Troegel S, Lenz M. Amount, distribution and composition of large microplastics in typical agricultural soils in northern Germany. Sci Total Environ (2021) 758:143615. doi: 10.1016/j.scitotenv.2020.143615
33. Piehl S, Leibner A, Löder MGJ, Dris R, Bogner C, Laforsch C. Identification and quantification of macro- and microplastics on an agricultural farmland,”. Sci Rep (2018) 8(1):pp.1–9. doi: 10.1038/s41598-018-36172-y
34. Public Health England and Wales. The sludge (Use in agriculture) regulations 1989 (1989). Available at: http://www.legislation.gov.uk/uksi/1989/1263/made.
35. Radford F, Zapata-Restrepo LM, Horton AA, Hudson MD, Shaw PJ, Williams ID. Developing a systematic method for extraction of microplastics in soils. Anal Methods (2021) 13(14):1695–705. doi: 10.1039/D0AY02086A
36. Stead JL, Cundy AB, Hudson MD, Thompson CEL, Williams ID, Russell AE, et al. Identification of tidal trapping of microplastics in a temperate salt marsh system using sea surface microlayer sampling. Sci Rep (2020) 10(1):14147. doi: 10.1038/s41598-020-70306-5
37. Primpke S, Cross RK, Mintenig SM, Simon M, Vianello A, Gerdts G, et al. Toward the systematic identification of microplastics in the environment: Evaluation of a new independent software tool (siMPle) for spectroscopic analysis. Appl Spectrosc (2020) 74(9):1127–38. doi: 10.1177/0003702820917760
38. Sun X, Wang T, Chen B, Booth AM, Liu S, Wang R, et al. Factors influencing the occurrence and distribution of microplastics in coastal sediments: From source to sink. Journal of hazardous materials. Hazard Mat (2021) 410:124982. doi: 10.1016/j.jhazmat.2020.124982
39. Scarascia-Mugnozza G, Sica C, Russo G. Plastic materials in European agriculture: actual use and perspectives. J Agric Eng (2011) 42(3):15–28. doi: 10.4081/jae.2011.3.15
40. Clayton GW, Harker KN, O’Donovan JT, Blackshaw RE, Dosdall L, Stevenson FC, et al. Polymer seed coating of early-and late-fall-seeded herbicide-tolerant canola (Brassica napus l.) cultivars. Can J Plant Sci (2004) 84(4):971–9. doi: 10.4141/P03-185
41. Liu M, Lu S, Song Y, Lei L, Hu J, Lv W, et al. Microplastic and mesoplastic pollution in farmland soils in suburbs of shanghai, China. Environ pollut (2018) 242:855–62. doi: 10.1016/j.envpol.2018.07.051
42. Chau C, Paulillo A, Lu N, Miodownik M, Lettieri P. The environmental performance of protecting seedlings with plastic tree shelters for afforestation in temperate oceanic regions: A UK case study. Sci Total Environ (2021) 791:148239. doi: 10.1016/j.scitotenv.2021.148239
43. Cunningham RB, Lindenmayer DB. Modeling count data of rare species: some statistical issues. Ecology (2005) 86(5):1135–42. doi: 10.1890/04-0589
44. Klein M, Fischer EK. Microplastic abundance in atmospheric deposition within the metropolitan area of Hamburg, Germany. Sci Total Environ (2019) 685:96–103. doi: 10.1016/j.scitotenv.2019.05.405
45. Allen S, Allen D, Phoenix VR, le Roux G, Durántez Jiménez P, Simonneau A, et al. Atmospheric transport and deposition of microplastics in a remote mountain catchment. Nat Geosci (2019) 12(5):339–44. doi: 10.1038/s41561-019-0335-5
46. Tagg AS, Brandes E, Fischer F, Fischer D, Brandt J, Labrenz M. Agricultural application of microplastic-rich sewage sludge leads to further uncontrolled contamination. Sci Total Environ (2022) 806:150611. doi: 10.1016/j.scitotenv.2021.150611
47. Koutnik VS, Leonard J, Alkidim S, DePrima FJ, Ravi S, Hoek E, et al. Distribution of microplastics in soil and freshwater environments: Global analysis and framework for transport modeling. Environ pollut (2021) 274:116552. doi: 10.1016/j.envpol.2021.116552
48. Fakour H, Lo SL, Yoashi NT, Massao AM, Lema NN, Mkhontfo FB, et al. Quantification and analysis of microplastics in farmland soils: Characterization, sources, and pathways. Agric (Switzerland) (2021) 11(4):330. doi: 10.3390/agriculture11040330
49. Bläsing M, Amelung W. Plastics in soil: Analytical methods and possible sources. Sci Total Environ (2018) 612:422–35. doi: 10.1016/j.scitotenv.2017.08.086
50. Campanale C, Galafassi S, Savino I, Massarelli C, Ancona V, Volta P, et al. Microplastics pollution in the terrestrial environments: Poorly known diffuse sources and implications for plants. Sci Total Environ (2022) 805:150431. doi: 10.1016/j.scitotenv.2021.150431
51. Yang J, Li L, Li R, Xu L, Shen Y, Li S, et al. Microplastics in an agricultural soil following repeated application of three types of sewage sludge: A field study. Environ pollut (2021) 289:117943. doi: 10.1016/j.envpol.2021.117943
52. Mirzavand J, Moradi-Talebbeigi R. Relationships between field management, soil compaction, and crop productivity. Arch Agron Soil Sci (2021) 67(5):675–86. doi: 10.1080/03650340.2020.1749267
53. Kim S-K, Kim J-S, Lee H, Lee H-J. Abundance and characteristics of microplastics in soils with different agricultural practices: Importance of sources with internal origin and environmental fate. J Hazardous Mater (2021) 403:123997. doi: 10.1016/j.jhazmat.2020.123997
54. Boardman J, Burt T, Foster I. Monitoring soil erosion on agricultural land: results and implications for the rother valley, West Sussex, UK. Earth Surface Processes Landforms (2020) 45(15):3931–42. doi: 10.1002/esp.5011
55. Ding L, Wang X, Ouyang Z, Chen Y, Wang X, Liu D, et al. The occurrence of microplastic in mu us sand land soils in northwest China: Different soil types, vegetation cover and restoration years. J Hazardous Mater (2021) 403:123982. doi: 10.1016/j.jhazmat.2020.123982
56. Dris R, Gasperi J, Saad M, Mirande C, Tassin B. Synthetic fibers in atmospheric fallout: A source of microplastics in the environment? Mar pollut Bull (2016) 104(1–2):290–3. doi: 10.1016/j.marpolbul.2016.01.006
57. Lwanga EH, Gertsen H, Gooren H, Peters P, Salánki T, van der Ploeg M, et al. Incorporation of microplastics from litter into burrows of lumbricus terrestris. Environ pollut (2017) 220:523–31. doi: 10.1016/j.envpol.2016.09.096
58. Accinelli C, Abbas HK, Shier WT, Vicari A, Little NS, Aloise MR, et al. Degradation of microplastic seed film-coating fragments in soil. Chemosphere (2019) 226:pp.645–650. doi: 10.1016/j.chemosphere.2019.03.161
59. Patzold S, Mertens FM, Bornemann L, Koleczek B, Franke J, Feilhauer H, et al. Soil heterogeneity at the field scale: A challenge for precision crop protection. Precis Agric (2008) 9:367–90. doi: 10.1007/s11119-008-9077-x
60. Way C, Hudson MD, Williams ID, Langley GJ. Evidence of underestimation in microplastic research: A meta-analysis of recovery rate studies,”. Sci Total Environ (2022) 805:150227. doi: 10.1016/j.scitotenv.2021.150227
61. Song YK, Hong SH, Jang M, Han GM, Rani M, Lee J, et al. A comparison of microscopic and spectroscopic identification methods for analysis of microplastics in environmental samples. Mar pollut Bull (2015) 93(1–2):202–9. doi: 10.1016/j.marpolbul.2015.01.015
Keywords: biosolids, microplastics, agricultural, soils, contamination
Citation: Radford F, Horton A, Hudson M, Shaw P and Williams I (2023) Agricultural soils and microplastics: Are biosolids the problem? Front. Soil Sci. 2:941837. doi: 10.3389/fsoil.2022.941837
Received: 11 May 2022; Accepted: 12 December 2022;
Published: 19 January 2023.
Edited by:
Baljinder Singh, Panjab University, IndiaReviewed by:
Changyin Zhu, Nanjing University, ChinaDominic Brose, Metropolitan Water Reclamation District of Greater Chicago (MWRDGC), United States
Copyright © 2023 Radford, Horton, Hudson, Shaw and Williams. This is an open-access article distributed under the terms of the Creative Commons Attribution License (CC BY). The use, distribution or reproduction in other forums is permitted, provided the original author(s) and the copyright owner(s) are credited and that the original publication in this journal is cited, in accordance with accepted academic practice. No use, distribution or reproduction is permitted which does not comply with these terms.
*Correspondence: Freya Radford, ZnJleWEucmFkZm9yZEB1d2UuYWMudWs=
†ORCID: Alice Horton, orcid.org/0000-0001-6058-6048
Peter Shaw, orcid.org/0000-0003-0925-5010
Ian Williams, orcid.org/0000-0002-0121-1219