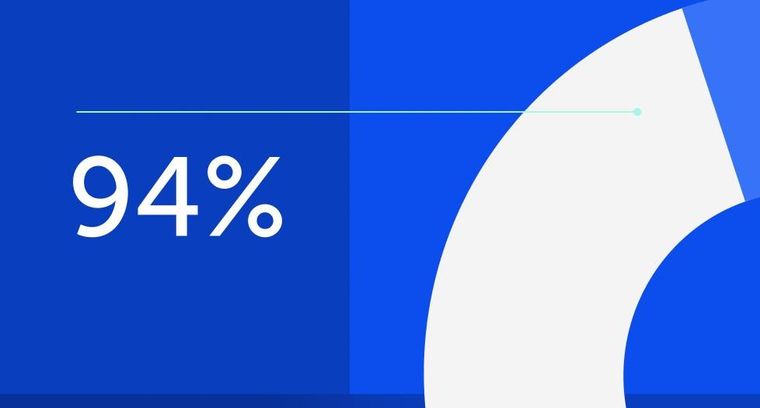
94% of researchers rate our articles as excellent or good
Learn more about the work of our research integrity team to safeguard the quality of each article we publish.
Find out more
ORIGINAL RESEARCH article
Front. Soil Sci., 07 July 2022
Sec. Soil Pollution & Remediation
Volume 2 - 2022 | https://doi.org/10.3389/fsoil.2022.934491
This article is part of the Research TopicGlobal Excellence in Soil Pollution and Remediation: Europe 2022View all 4 articles
Phytostabilization is a more appropriate way for rehabilitation of metal-polluted environments in the Copperbelt region. A 1.5-hectare tree planting trial had been installed on the tailings dam (TD) with the help of organic matter (OM) mixed to topsoil. Then, 15 years later, this study aimed to evaluate the performance of the tree plantation, in regard to soils and spontaneous vegetation. The Cu and Co concentration was higher in the reclaimed soil compared with the unreclaimed. Acacia auriculiformis, Acacia polyacantha, Albizia lebbeck, Pinus sylvestris, Psidium guajava, Senna siamea, Senna spectabilis, and Syzygium guineense responded well to the criteria of phytostabilization, whereas Leucaena leucocephala was very invasive. Metal concentration was manifold higher in charcoals than in woods (Zn: 3.8–39.8 mg kg−1 in wood versus 272–523 mg kg−1 in charcoal) with no differences between charcoals regardless of the origin. The tree implantation aided with amendments can meet the criteria of phytostabilization through good survival and regeneration in the heavily contaminated TD.
Mining is one of the larger spreaders of trace metals in the environment (1). Once trace metals are introduced into the environment, they cannot be biodegraded; they persist indefinitely and cause pollution of air, water, and soils (2, 3). The contamination of soil with trace metals leads to the loss of species diversity, resulting in the replacement of the original flora with a metal-tolerant flora, sparse vegetation, or large areas of bare soil (4–7). When polluted lands remain bare and unreclaimed for a long time, they can spread trace metals to larger areas via eolian dispersion and water erosion contaminating agricultural lands, surface, and groundwater over many years (8). People may be exposed to trace metal hazards through food consumption, dust inhalation, dermal contact, and ingestion (for children) from polluted lands (9–13). In the Copperbelt region, for instance, a last epidemiological study in Katanga reported much higher trace metal concentrations (particularly As, Cd, Co, Pb, and U) in the urine of the neighboring human populations living close to mining areas and smelting plants representing a serious health hazard compared with those from a non-mining area (14). Three types of areas affected by mining and ore processing were identified in the Copperbelt region: mine deposits from quarries, and soils contaminated by metalliferous fallout from the copper smelter and tailings from hydrometallurgical process (15, 16). The tailings dam (TD) in Katanga Copperbelt can create more concerns given the larger and deeper soil volumes that they have (7, 17, 18), and then, their management to prevent health risks and environmental damage is as challenging in this region as it is in other mining regions throughout the world (18, 19), mostly due to the high cost, the unfeasibility in large areas, and creation of secondary pollution problems by the physicochemical soil remediation engineering techniques (19–22). For this reason, the use of phytoremediation becomes very crucial, because it is an efficient, low cost, and eco-friendly technology, based on plants that has been carried for land remediation in these recent years (22–25). Among the various phytoremediation techniques, phytostabilization is indicated as very suitable for large and elevated trace metal concentration areas (26), because it is a technique that uses plants to contain metals in the soil and prevent their leaching or lateral migration (20, 24). The successful phytostabilization will be observed when there is a long-term succession of the plant community in TD to promote soil development processes and microbial diversity and, finally, to restore soil ecosystem functions to a state of self-sustainability (8). Burges et al. (27) showed that phytoremediation should be based not only on the reduction of contaminants but also on the restoration/or regeneration of economic gain and other wider services, becoming then the phytomanagement. Tree species are suitable to respond to this purpose because wood of the mature tree is used in the manufacture of commercial products; if the metal uptake of the species in wood is low, then it is feasible to harvest the wood grown on heavy-affected soils for making paper and furniture (26, 28) or energy production (e.g., charcoal or firewood) (29). Since, the miombo woodland in the Copperbelt region is permanently regressing, mainly because of slash-and-burn agriculture, charcoal production, and timber harvesting (30, 31). Therefore, reforestation with native and exotic fast-growing species is a means of combating forest degradation and reducing pressure on forests. In this way, the installation of woody species in polluted areas is also a good option to contribute to the reduction of wood harvesting in natural forests by recovering polluted sites (32). However, knowing the trace metal concentration status of tree species prior to firewood or charcoal production would be very crucial in this kind of environment to prevent transfer of trace metals from polluted lands to residential plots (28, 33). The objectives of this study were to (i) compare the macro and metal-element content between the reclaimed soil and bare soil on TD, (ii) assess the performance of the tree plantation and the diversity of spontaneous species facilitated by tree species, and (iii) investigate metal content in tissues of tree species and their implications on charcoal production.
The study was conducted at Kipushi, 30 km west of the city of Lubumbashi in the Upper Katanga province, DR Congo (11° 46′ 27.1″ N and 27° 16′ 21.6″ E with an altitude ranging 1,300 m). The climate is the same as in Lubumbashi characterized by 6-month respective dry (May to October) and rainy (November to April) seasons, with 1,300 mm of annual rainfall. Temperatures range from 16° to 33°C, with the lowest values in the first half of the dry season (June to July). Three big TD are found in this mining town of Kipushi, created by the liquid discharges from the processing of copper–zinc suldide ores since 1960 (34). The TD was created in the lowland where miombo woodland vegetation was replaced by bare soil due to the change of physicochemical conditions of soils. Because of its proximity to the residential areas, the size, and the hazards to the public health, one of the three Kipushi TDs was selected for the trial of tree planting in 2005. This TD covers around 146 hectares, with the tree plantation covering 1.5 hectare. The circular planting holes had on average 2-m diameter and 1-m depth in which all the TD soil was removed and replaced by the amendments (organic amendments combined with topsoil from uncontaminated areas), and the seedlings composed of different tree species were planted in 2005.
The sampling of soil was stratified, with sampling points distant of 35 m × 30 m. The number of samples was 16, i.e., 12 samples were collected in the reclaimed area and four samples (controls) in the unreclaimed area (bare soil). At each point, a soil sample was taken at three different depth measurements (0 to 20, 20 to 40, and 40 to 60 cm) using the auger.
The vegetation inventory was conducted during the years 2019–2020 (whereas trees were planted in 2005). A total of 258 amended holes filled with amendments were counted, but only 191 were having living trees composed of 10 different species, namely, Acacia auriculiformis, Acacia polyacantha, Albizia lebbeck, Cupressus lusitanica, Leucaena leucocephala, Pinus sylvestris, Psidium guajava, Senna siamea, Senna spectabilis, and Syzygium guineense with an unequal number of individuals. The inventory of spontaneous species was carried out in rainy season (January 2020). Then, squares of 9 m² were established for the inventory of spontaneous species that established themselves around planted trees and squares of 12m² for the inventory of spontaneous species that established themselves in between tree lines. The species recovery was done according to the Braun-Blanquet’s phytosociological method, where the defined species are given an abundance–dominance coefficient.
Leaves, barks, and wood were collected from two trees of species that can provide good wood usable for energy or timber, namely, Acacia auriculiformis, Acacia polyacantha, Albizia lebbeck, Cupressus lusitanica, Leucaena leucocephala, and Syzygium guineense.
Micromillstones were made to produce charcoals from the wood of A. auriculiformis and L. leucocephala, chosen for their high height and number of individuals on the TD plantation. The same was done with their wood in the non-polluted areas. Four micromillstones were made for each species in the polluted and the non-polluted area, and, lastly, four samples of charcoals were randomly taken from millstones made of miombo woodland trees (dominated by Brachystegia boehmii and Julbernadia paniculata).
Soil analyses were performed on air-dried samples (2 mm). The total organic carbon, pH KCl, available macronutrients (phosphorous, potassium, calcium, and magnesium), and trace metals (copper, cobalt, and zinc) were analyzed using the ammonium acetate-EDTA extraction at the Provincial Centre for Agriculture and Rurality (CPAR) of La Hulpe, Belgium. The average fractions of Cu, Co, and Zn total concentrations in this TD are 1,700, 45, and 14,000 mg kg−1, respectively [see also Pourret et al. (16)]. The plant samples consisted of wood (a tree branch of 15 cm long), leaves, and barks (barks were separated from the same wood after drying). Leaves samples were washed with tap water and then with distilled water, after all air-dried samples were put into paper envelopes and dried together in the proofer at the temperature of 95°C during 72 h for leaves and 120 h for woods and barks to obtain dry weight. Then, these were crushed into powder for mineralization attack and analyses in the laboratory of Gembloux Agro-Bio Tech, Axe Echanges Eau-Sol Plant, University of Liège. Two grams of homogenized samples were weighed into a 150-ml beaker and attacked with 30 ml of mixed 65% HNO3 and 70% HClO4 on a plate for a cold reaction during 16 h; then, the solution is heated until the solid residues remain; after cooling, 5 ml of 10% HCl was added unto the residues, poured into a 25-ml volumetric flask, and diluted to the mark. The concentrations of Cu, Co, and Zn in the digests were determined using a flame atomic absorption spectrometer. The results were obtained in milligrams per liter and then converted into milligrams per kilogram. Charcoals were crushed and sieved into a 2-mm mesh sieve and followed the same process of mineralization and analysis as plant organs.
Data from chemical elements in soil, tree organs, and charcoals were analyzed using R software (version 4.0.3). The fitting of the data to a normal distribution for all parameters measured was checked with the Shapiro–Wilk test. When necessary, analytical data were transformed using logarithms to assure normal distribution. Two-way ANOVA was applied on soil, 2 soils (reclaimed and unreclaimed) and 3 depths (0-20, 20-40 and 40-60 cm) and as well as for charcoals, main samples (wood and charcoal) and subsamples (tree species and their origin); and one-way ANOVA was applied for comparing metal concentration according to organs and according to species. Means were compared using the Tukey’s (honestly significant difference) HSD test with an error of 5%. Species richness and Shannon’s diversity index were computed, concerning the influence of tree species on spontaneous vegetation diversity, and the relative abundance was calculated to assess the diversity in spontaneous vegetation around trees and in corridors. The computations were done after transformation of Braun-Blanquet coefficients into percentage with Shannon index (H′) and the evenness (EH); the square around tree species is considered in this case as specific habitats. The species richness and the recovery rate of the spontaneous vegetation were compared using a one-way ANOVA with tree species as factors.
There was no reclaimed soil, depth, and interaction effect on the concentration of available macronutrients EDTA (phosphorous, potassium, calcium, and magnesium), pH KCl, and total organic carbon after the ANOVA test (p > 0.05). However, ranges between minimum and maximum of phosphorous and potassium were larger in the reclaimed soil compared with the unreclaimed soil; and the pH level was alkaline in both soil conditions (7.0 to 7.7). The available concentration of trace metals indicates that this TD is extremely contaminated in Zn and Cu both on reclaimed and bare soil compared with the pedo-geochemical background of this region, which is of Zn, Cu, and Co of 69, 187, and 20 mg kg−1, respectively (35). However, copper and cobalt concentration on the mobile fraction of metals was significantly high in the reclaimed soil (composed of woody and herbaceous plants) than in the bare soil (p < 0.05) with no significant difference for zinc; however, the two first depths tended to have a higher average, median, and maximum Zn concentration in the reclaimed soil than in the bare soil (Figures 1, 2).
Figure 1 Extractible macronutrient concentration and pH in the reclaimed soil (RS) and on the bare soil (BS) at different depth (0–20cm, 20–40cm, and 40–60 cm). n = 12 for RS, and n = 4 for BS. TOC, total organic carbon. (A): P, (B): K, (C): Mg, (D): Ca, (E): pH, (F): TOC.
Figure 2 Available trace metal concentration (mg kg−1) in the reclaimed soil (RS) and on bare soil (BS) at different depth measurements (0–20cm, 20–40cm, and 40–60 cm). n = 12 for RS, and n = 4 for BS. (A): Cu, (B): Co, (C): Zn.
A total of 126 trees with dbh ≥ 10 cm were numbered in the Kipushi TD (1.5 ha) in 2020 (planted in 2005). Ten trees species with an uneven number of tree individuals are listed in Table 1; L. lecocephala had the most abundant tree individuals. It is followed by S. siamea, A. polyacantha, and A. lebbeck. Then, C. lusitanica, A. auriculiformis, P. sylvestris, S. spectabilis, and P. guajava contributed with four to six individuals, whereas S. guineense was only represented by one individual. A. auriculiformis had the highest mean height (6.7 m) and dbh (21.9 cm), and the other species had the height ranging from 3.4 to 5.5 m and the dbh from 7.9 to 16.6 cm. An individual was counted as juveniles when it had a height from 15 to 150 cm; L. leucocephala had highest and increasing number of juveniles (716 to 1,032), followed by A. lebbeck (38 to 81), A. polyacantha (2 to 25), P. guajava (8 to 15), and S. spectabilis (4 to 15) between the 2 years. A. auriculiformis, C. lusitanica, P. sylvestris, S. siamea, and S. guineense had a decrease to nil juveniles between the 2 years.
Table 1 Dendrometric characteristics and performance of tree species in the TD 15 years after planting (1.5 ha).
Table 2 shows the diversity of vegetation that has been established naturally around the woody species planted on the TD with P. guajava having a high number of spontaneous species (11 species) in comparison with other tree species (six to seven species) and C. lusitanica having a lower number of spontaneous species (four species).
Table 2 Diversity of spontaneous species around planted woody species (CL, C. lusitanica; SS, S. siamea; SSp, S. spectabilis; PS, P. sylvestris; LL, L. leucocephala; AP, A. polyacantha; PG, P. guajava; AA, A. auriculiformis; AL, A. lebbeck).
Eight tree species shared nearly the same recovery rate of spontaneous species, ranging from 70.8% to 166.3% (ANOVA, p = 0.000); C. lusitanica (32.0%) had the lowest recovery rate of species. Considering Shannon diversity (H′), C. lusitanica had the lowest diversity (1.97) and evenness of spontaneous species (0.60), and L. leucocephala showed lower evenness in its habitats (0.63), whereas the diversity was nearly the same around other tree species (Table 2).
Thirty-four species in total were recorded on the 64 square around tree species, each species shown with its biological and ecological types—the ecological type being the relation of species to trace metals on TD. Tithonia diversifolia, Leucaena leucocephala, Imperata cylindrica, Celosia trigyna, Lantana camara, Cyperus sp., Michrochloa altera, and Hyparrhenia rufa are the species that were the most abundant in the square around trees with 22.3%, 21.7%, 18.5%, 9.6%, 4.2%, 2.5%, 2.3%, and 2.1% respectively; and the rest of the species had a lower abundance that ranged from 1.9% to 0.02% (Table 3).
Table 3 Spontaneous vegetation around main tree species (64 quadrats of 9 m2 around trees in the plantation of 15,000 m2; RA, relative abundance).
A total of 28 species were recorded on 34 quadrats in the corridors of trees, each species with its biological and ecological characteristics. C. trigyna (33.2%), I. cylindrica (26.1%), M. altera (9.5%), B. pseudoperennis (6.9%), L. leucocephala (6.1%), T. diversifolia (5.9%), and A. hispidus (2.9%) were the most abundant and the rest of the species had a relative abundance lower than 2% (Table 4).
Table 4 Spontaneous vegetation in tree corridors (34 quadrats of 12-m2 17 corridors in the plantation of 15,000 m2; RA, relative abundance).
Both soil around trees and on corridors are largely dominated by therophytes (44.1% and 42.9%, respectively), followed by phanerophytes (20.6%), hemicryptophytes (14.7%), and less than 9% for other types on soil conditions around trees; whereas, hemicryptophytes (21.4%), then phanerophytes (17.9%), and other types with less than 8% rate on soil conditions of corridors (Table 5). Weedy taxa were the most dominant spontaneous species in this TD; juveniles of trees, true metallophytes, and facultative metallophytes had the same proportion around trees, whereas facultative metallophytes, true metallophytes, and tree juveniles had different rates (25.0%, 21.4%, and 10.7% respectively) in corridors.
The ANOVA test showed no significant difference on concentration means of Cu and Co in leaves and of Cu and Zn in wood among tree species altogether (Table 6). However, the concentration of Zn trended to be high in leaves and barks of C. lusitanica 426.1 mg kg−1 and 528.9 mg kg−1, respectively, and in barks of L. leucocephala (710.2 mg kg−1), whereas S. guineense trended to have high Co concentration in wood and barks (3.3 and 26.4 mg kg−1, respectively). Compared with the toxicity limit range, barks were the organs that had Cu and Zn concentrations above the limits for C. lusitanica and S. guineense, and Zn alone for L. leucocephala and A. lebbeck; whereas only C. lusitanica had higher Zn concentration in leaves.
Table 6 Trace metal concentration in organs according to the species and range of allowable concentration limits (mg kg−1 dry weight).
Table 7 Comparison of trace metal concentration in leaves, wood, and barks of tree species (mg kg−1 dry weight; n = 2).
Two way-ANOVA with samples (wood and charcoal) as the first factor and subsamples (wood and charcoals from different species and soils) as the second factor showed high significant differences between charcoal and wood samples and between subsamples and the interaction effect (p < 0.001 and p <0.01) (Table 8). The concentration of Cu, Co, and Zn was manifold higher in charcoals compared with wood for all species and soils. There was no any significant difference of metal concentration in charcoals of A. auriculiformis and L. leucocephala from TD soil and normal soil, but charcoals from these two species (A. auriculiformis and L. leucocephala) had a high Cu and Co concentration than in charcoal from miombo woodland species except the zinc concentration that was similar in charcoals altogether. Whereas, no clear tendency could be drawn concerning metal concentration in wood from the three groups, i.e., A. auriculiformis and L. leucocephala wood from TD, A. auriculiformis and L. leucocephala wood from normal soil, and miombo wood trees, with lower and closer metal concentrations.
Table 8 Trace metal concentration in wood and charcoals from contaminated and normal sites (mg kg−1 dry weight; n = 4) . AA, A. auriculiformis; LL, L. leucocephala.
Fertility parameters (phosphorous, potassium, magnesium, calcium, total organic carbon, and pH) in TD did not differ horizontally (at the reclaimed soil and bare soil) and vertically (from 0 to 60 cm). The similarity of P, K, and TOC between these two parts of soil may be due to the fact that the vegetation installed has not yet provided enough organic matters to improve fertility in the corridors of trees where samples were collected. It is also worth noting that dense and closed vegetation was observed around trees than on the corridors because of the amendments made to the trees corroborating the result of Shutcha et al. (5), where the vegetation plots amended with compost and limestone had significantly high P, TOC, pH, and Ca than on the bare soil 5 years later. Moreover, the concentration ranges of P and K (P, 1–9 mg kg−1; K, 10–45 mg kg−1) of this TD were relatively low compared with non-disturbed metalliferous and non-metalliferous soils of this region (P, 5–772 mg kg−1; K, 10–163 mg kg−1) (15, 38). The high Ca, Mg, and alkaline pH (7.5) are due to the addition of lime by the mining companies (34, 39). The TOC on the bare soil of Kipushi TD (1.6%) was also found by Pourret et al. (16) (2%) and was largely higher than the TOC concentration on bare soil metalliferous fallout from copper smelter (0.3%) (5). The trace metal concentration was higher (mostly Co and Cu) in the reclaimed soil than in the bare soil; this result goes in the same line with that of Shutcha et al. (5) who justified it by the erosion effect, in which the bare soil is permanently undergoing through wind and rainwater carrying trace metals to other new areas, increasing the contamination risk to different compartments of the environment (40, 41). The concentration of trace metals did not differ from soil surface to 60-cm depth, showing that the contaminated layer of this TD was deeper in contrast to the metalliferous fallout soils from smelter that have a high concentration of metals on the surface that decreases with depth (4).
The soil in this TD was bare, with no single plant species present at the time of tree implantation, except on the top of termite mounds (42). Fifteen years after tree planting, 10 tree species overall have been identified as thriving well, and the high growth rate and tolerance to high heavy metal concentrations have been indicated by Ali et al. (43) to be among the beneficial traits for qualified species for phytostabilization. L. leucocephala (73 stems) had the largest number of stems, seven times higher than S. siamea (11 stems) that was the second in number of stems. The high number of stems of L. leucocephala is also due to its high and fast capacity to regenerate, as L. leucocephala could regenerate on the amended pits of another species or of the same species in a very short period after planting (personal observation). Spontaneous regeneration on the polluted soil should be considered to select those who present good traits for phytostabilization (44, 45). However, the high and fast capacity to regenerate and the large number of juveniles recorded in this TD confirm the invasive character of L. leucocephala already reported in several regions of the world (46, 47). All species, except C. lusitanica, could have juveniles reaching up to 15 cm high, but L. leucocephala, A. lebbeck, A. polyacantha, P. guajava, and S. spectabilis showed an increasing number of juveniles within 2 years (2020 and 2021).
The seedlings from A. auriculiformis, P. sylvestris, S. siamea, S. guineense, and C. lusitanica reaching 15 cm of height were scarce, and the few that grew could not survive in the period of 1 year.
The phytoremediation potential of some species used in this trial was also reported by other researchers: A. lebbeck and A. auriculiformis on Cr-contaminated soil in India (48) and P. guajava on Cu- and Ni-contaminated soil as a high tolerance species to metal stress in Zambia, Brazil, and Italy (49–51); A. polyacantha and S. guineense on copper-rich TD with high tolerance in Zambia (39, 49) were the two native species meeting the phytostabilization criterion that the species should be native to the region that needs to be depolluted (52, 53).
The assessment of spontaneous vegetation on the 9 m2 around tree species indicated that the species richness ranged from 4 to 11, with C. lusitanica (4) and A. lebbeck (6) having a lower number of species and P. guajava (11) having a higher number of species. The species’ recovery rates ranged from 27.3% to 164.2% with C. lusitanica, S. siamea, P. sylvestris, and S. spectabilis having a lower recovery rate and L. leucocephala, P. guajava, and A. polyacantha having a higher recovery rate of spontaneous vegetation. However, the species richness, recovery rate, and diversity of spontaneous species depend not only on the main tree species to facilitate spontaneous colonization but also on the amendments used when planting the trees and the tree-shade density accompanied by thick litter. Cordova et al. (54) showed that the application of compost and lime on trace metal-polluted soil could lead to the same plant cover and biomass production between spontaneous and assisted revegetation, implying that the sole use of amendments on trace metal contaminated soil can induce spontaneous revegetation when the disturbance is reduced and a seed bank is sufficient (39, 55). However, active human intervention is necessary for efforts to accelerate and/or influence the successional trajectory and provide to the contaminated area with a reclamation pattern (8, 27, 56). In terms of relative abundance of spontaneous vegetation, facultative metallophytes (T. diversifolia, 22.3%; I. cylindrica, 18.5%; L. camara, 4.2%; H. rufa, 2.1%) were the most dominant around trees, whereas true metallophytes (C. trigyna, 33.2%; M. altera, 9.5%; B. pseudoperennis, 6.9%; A. micans, 2.9%) dominated on the corridors. Soil conditions around trees were more favorable due to amendments applied in the plantation hole to aid phytostabilization (44, 54), whereas in corridors, these were the tailings without any amendment influence. Weedy taxa, true metallophytes, and facultative metallophytes are also important groups of species that should be looked upon to select useful plants for phytostabilization (45); nevertheless, species already reported as invasive, such as T. diversifolia (57) and L. camara (58), should be avoided in the reclamation of degraded lands. M. altera has been proven as a good hemicryptophyte species to be used for phytostabilization of polluted lands in the Copperbelt region (59). It is also worth noting that facultative metallophytes are species that can have some populations in metal-rich soil (37) and non-metal-rich soil; while weedy taxa and tree juveniles are just non-metallophyte species.
One of the criteria for plants suitable for phytostabilization is to keep root-to-shoot translocation as small as possible (excluder behavior; 60) to prevent the entry of contaminants into the food chain (8, 61).
The metal accumulation in plants may be tissue-specific (62, 63), metal-specific, or species-specific characteristics (64–66). This result suggests that more significant differences between these tree species were observed on barks concerning Cu, Zn, and Co concentration in organs than on leaves and wood, with C. lusitanica, S. guineense, and L. leucocephala tending to have higher concentrations than A. auriculiformis, A. polyacantha, and A. lebbeck. The concentration of these three trace metals in tree tissues (Cu, Co, and Zn) followed this pattern: bark > leaves > wood for C. lusitanica, S. guineense, L. leucocephala, and A. lebbeck and A. polyacantha likewise on copper, but the pattern for A. auriculiformis was bark = leaves > wood. These results are confirmed by other investigations (on Pinus pinea and Nerium oleander, and in poplar coppice culture) showing trace metal concentrations being lower in wood compared with bark and leaves; metals in bark could be lower, equal, or higher depending on the type of metals or species (62, 67). Metal contents in bark tissues were above the toxicity limit range concerning Cu (20–100 mg kg−1) or Zn (100–400 mg kg−1) for C. lusitanica, S. guineense, L. leucocephala, and A. lebbeck, but wood was far below the toxicity limit range. Oliva and Mingorance (62) suggested that wood can accumulate elements mainly from the soil and its metal concentration does not reflect the atmospheric pollution, whereas bark can accumulate atmospheric pollutants through wet and dry deposition and has been found to be a useful bioindicator and biomonitor for airborne pollution monitoring.
It is feasible to harvest the wood of the mature trees grown on heavy metal-affected soils for energy production, i.e., charcoal or firewood (26, 29). This study points out the trace metal concentration status of wood charcoal from A. auriculiformis and L. leucocephala from polluted and unpolluted land and with miombo woodland species; the findings show that metal concentrations in charcoal from all species were far higher in charcoal than in wood regardless of the origin (Cu: charcoal, 8.5–159.9 mg kg−1; wood, 0.4–3.4 mg kg−1; Co: charcoal, 18.5–38.4 mg kg−1; wood, 0.4 mg kg−1; Zn: charcoal, 272–523 mg kg−1; wood, 3.8–39.8 mg kg−1) with the exception of Co content in miombo woodland charcoal, which was the same in charcoals and in wood. The high metal content in charcoals than in wood of the same species may be due to the loss of all the fresh matters from the wood product that might lead to the increase of metal concentration as metals do not volatilize during the burning process or the contamination of charcoals from soil (62) during the manufacturing time, because the soil geochemical background of this region is characterized by metal-rich soil (35). The European Commission decision suggested the limits of trace metal concentration in soil improvers with Cu of 100 mg kg−1 and Zn of 300 mg kg−1 (68), which may lead to analyze metal concentration in the ash from these charcoals and know whether there is a risk of metal contamination to gardens or residential plots; however, Mollon et al. (69) suggested that ash amendment loaded with metals may increase metal concentration in soil and plants mostly when it is applied alone; however, when combined with manure or compost, the humic acid matrix of OM and the liming effect increasing pH reduce significantly the effect of metals on soil and their absorption by plants.
This study reports the results from the plantation trial of mixed exotic and native tree species for the phytostabilization of the Kipushi TD aided with organic matter and topsoil amendments. This TD is extremely rich in Zn and Cu, but Cu accompanied by Co concentration was clearly higher in the reclaimed soil than in the bare soil, which confirms the metal stabilization effect of plants in this phytoremediation strategy. However, there was no significant difference of K and P content between these two soils. Two native species (A. polyacantha and S. guineense) and eight exotic species (A. auriculiformis, A. lebbeck, C. lusitanica, L. leucocephala, P. sylvestris, P. guajava, S. siamea, and S. spectabilis) were recorded 15 years after planting. Beyond growth and survival, L. leucocephala, P. guajava, A. polyacantha, and A. lebbeck showed good performance in regeneration and the facilitation of spontaneous species. However, it would be better to avoid the use of L. leucocephala in the phytostabilization program as much as possible, because of its invasive nature. Spontaneous vegetation had 28 species in corridors and 34 species around trees with different biological and ecological types. Barks followed by leaves were likely showing high Zn, Cu, and Co concentrations in most of the six tree species, whereas wood had far lower content. This testifies that the metal concentrations in these organs might be airborne rather than soilborne; however, the Zn, Cu, and Co concentrations were manifold higher in charcoal than in wood with no significant differences between charcoals regardless of the origin. Knowing metal concentration in wood ashes would be necessary because the ash has always been used by people as a soil amendment for gardening.
The raw data supporting the conclusions of this article will be made available by the authors, without undue reservation.
JK, MS, and GC designed the research; JK and SL performed the sampling and analyzed the data. JK wrote themanuscript. MS and GC corrected the manuscript. All authors contributed to the article and approved the submitted version.
The authors declare that the research was conducted in the absence of any commercial or financial relationships that could be construed as a potential conflict of interest.
All claims expressed in this article are solely those of the authors and do not necessarily represent those of their affiliated organizations, or those of the publisher, the editors and the reviewers. Any product that may be evaluated in this article, or claim that may be made by its manufacturer, is not guaranteed or endorsed by the publisher.
1. Candeias C, Melo R, Ávila PF, da Silva EF, Salgueiro AR, Teixeira JP. Heavy Metal Pollution in Mine-Soil-Plant System in S. Francisco De Assis-Panasqueira Mine (Portugal). Appl Geochem. (2014) 44:12–26. doi: 10.1016/j.apgeochem.2013.07.009
2. Khan MS, Zaidi A, Goel R, Musarrat J eds. Biomanagement of Metal-Contaminated Soils Vol. 20). Springer Science & Business Media (Dordrech Heidelberg London New York) (2011).
3. Małachowska-Jutsz A, Gnida A. Mechanisms of Stress Avoidance and Tolerance by Plants Used in Phytoremediation of Heavy Metals. Arch Environ Prot (2015) 41(4):104–14. doi: 101515/aep-2015-0045
4. Mpundu MM. Contaminations Des Sols En Eléments Traces Métalliques À Lubumbashi Katanga/RDCongo). Evaluation Des Risques De Contamination De La Chaîne Alimentaire Et Choix De Solutions De Remédiation. DR Congo: University of Lubumbashi (2010). p. 460.
5. Shutcha MN, Faucon M-P, Kamengwa CK, Colinet G, Mahy G, Ngongo ML, et al. Three Years of Phytostabilisation Experiment of Bare Acidic Soil Extremely Contaminated by Copper Smelting Using Plant Biodiversity of Metal-Rich Soils in Tropical Africa (Katanga. DR Congo). Ecol Eng (2015) 82:81–90. doi: 10.1016/j.ecoleng.2015.04.062
6. Chowdhury A, Maiti SK. Identification of Metal Tolerant Plant Species in Mangrove Ecosystem by Using Community Study and Multivariate Analysis: A Case Study From Indian Sunderban. Environ Earth Sci (2016) 75(9):1–21. doi: 10.1007/s12665-016-5391-1
7. Navarro-Cano JA, Verdú M, Goberna M. Trait-Based Selection of Nurse Plants to Restore Ecosystem Functions in Mine Tailings. J Appl Ecol (2018) 55(3):1195–206. doi: 10.1111/1365-2664.13094
8. Mendez MO, Maier RM. Phytostabilization of Mine Tailings in Arid and Semiarid Environments—an Emerging Remediation Technology. Environ Health Perspect (2008) 116(3):278–83. doi: 10.1289/ehp.10608
9. Kim H, Song B, Kim H, Park J. Distribution of Trace Metals at Two Abandoned Mine Sites in Korea and Arsenic-Associated Health Risk for the Residents. Toxicol Environ Heal Sci (2009) 1(2):83–90. doi: 10.1007/BF03216468
10. Liao X, Fu Y, He Y, Yang Y. Occurrence of Arsenic in Fruit of Mango Plant (Mangifera Indica L.) and its Relationship to Soil Properties. Catena (2014) 113:213–8. doi: 10.1016/j.catena.2013.07.011
11. Bošković-Rakočević L, Milivojević J, Milošević T, Paunović G. Heavy Metal Content of Soils and Plum Orchards in an Uncontaminated Area. Water. Air. Soil Pollution. (2014) 225(11):2199.
12. Naderizadeh Z, Khademi H, Ayoubi S. Biomonitoring of Atmospheric Heavy Metals Pollution Using Dust Deposited on Date Palm Leaves in Southwestern Iran. Atmósfera (2016) 29(2):141–55. doi: 10.20937/ATM.2016.29.02.04
13. Taghipour M, Ayoubi S, Khademi H. Contribution of Lithologic and Anthropogenic Factors to Surface Soil Heavy Metals in Western Iran Using Multivariate Geostatistical Analyses. Soil Sediment Contamination.: Int J (2011) 20(8):921–37. doi: 10.1080/15320383.2011.620045
14. Banza CLN, Nawrot TS, Haufroid V, Decrée S, De Putter T, Smolders E, et al. High Human Exposure to Cobalt and Other Metals in Katanga. A Mining Area of the Democratic Republic of Congo. Environ Res (2009) 109(6):745–52. doi: 10.1016/j.envres.2009.04.012
15. Faucon MP, Parmentier I, Colinet G, Mahy G, Ngongo Luhembwe M, Meerts P. May Rare Metallophytes Benefit From Disturbed Soils Following Mining Activity? The Case of the Crepidorhopalon Tenuis in Katanga (DR Congo). Restor Ecol (2011) 19(3):333–43. doi: 10.1111/j.1526-100X.2009.00585.x
16. Pourret O, Lange B, Bonhoure J, Colinet G, Decrée S, Mahy G, et al. Assessment of Soil Metal Distribution and Environmental Impact of Mining in Katanga (Democratic Republic of Congo). Appl Geochem. (2016) 64:43–55. doi: 10.1016/j.apgeochem.2015.07.012
17. Wong JWC, Ip CM, Wong MH. Acid-Forming Capacity of Pb-Zn Mine Tailings and its Implications for Mine Rehabilitation. Environ Geochem. Health (1998) 20:149–55. doi 10.1023/A:10065891242204
18. Kaniki AT, Tumba K. Management of Mineral Processing Tailings and Metallurgical Slags of the Congolese Copperbelt: Environmental Stakes and Perspectives. J Cleaner Production. (2019) 210:1406–13. doi: 10.1016/j.jclepro.2018.11.131
19. Wang L, Ji B, Hu Y, Liu R, Sun W. A Review on in Situ Phytoremediation of Mine Tailings. Chemosphere. (2017) 184:594–600. doi: 10.1016/j.chemosphere.2017.06.025
20. Berti WR, Cunningham SD. Phytostabilisation of Metals. In: Raskin I, Ensley BD, editors. Phytoremediation of Toxic Metals: Using Plants to Clean-Up the Environment. New York: Wiley (2000). p. pp 71–88.
21. Martin I, Bardos P. A Review of Full-Scale Treatment Technologies for the Remediation of Contaminated Land. In: Pulford ID, Watson C, editors. 2003. Phytoremediation of Heavy Metal-Contaminated Land by Trees-a Review. Environment International, vol. 29 . Richmond. Surrey: EPP Publications (1996). p. 529– 540.
22. Pulford ID, Watson C. Phytoremediation of Heavy Metal-Contaminated Land by Trees—a Review. Environ Int (2003) 29(4):529–40. doi: 10.1016/S0160-4120(02)00152-6
23. McCutcheon SC, Schnoor JL. Phytoremediation: Transformation and Control of Contaminants. (Washington, DC 20460, USA: John Wiley & Sons) (2004).
24. Pilon-Smits E. Phytoremediation. Annu Rev Plant Biol (2005) 56:15–39. doi: 10.1146/annurev.arplant.56.032604.144214
25. Lone MI, He ZL, Stoffella PJ, Yang XE. Phytoremediation of Heavy Metal Polluted Soils and Water: Progresses and Perspectives. J Zhejiang. Univ Sci B (2008) 9(3):210–20. doi: 10.1631/jzus.B0710633
26. Dubourguier H-C, Deram D, Logeay C. Le Phytomanagement: Eléments De Synthèse. Lille: Pôle de compétence sites et sédiments pollués (2001).
27. Burges A, Alkorta I, Epelde L, Garbisu C, Garbisu C. From Phytoremediation of Soil Contaminants to Phytomanagement of Ecosystem Services in Metal Contaminated Sites Int J Phytoremediation. (2018) 20(4):384–97. doi: 10.1016/j.scitotenv.2016.04.008
28. Meeinkuirt W, Kruatrachue M, Pitchel J, Phusantisampan T. & Saengwilai P. Influence of Organic Amendments on Phytostabilisation of Cd-Contaminated Soil by Eucalyptus Camaldulensis. ScienceAsia (2016) 42:83–91. doi: 10.2306/scienceasia1513-1874.2016.42.083
29. Prasad MNV, Sajwan KS, Naidu R. Phytoremediation Technologies Using Trees. In: Trace Elements in the Environment. (Boca Raton: CRC Press) (2005). p. 401–22.
30. Chidumayo EN. Woodland Structure, Destruction and Conservation in the Copperbelt Area of Zambia. Biol Conserv (1987) 40(2):89–100. doi: 10.1016/0006-3207(87)90060-7
31. Malambo FM, Syampungani S. Opportunities and Challenges for Sustainable Management of Miombo Woodlands: The Zambian Perspective. Work. Pap. Finn. For Res Inst (RDC) (2008) 98:125–30.
32. Kwesiga F, Akinnifesi FK, Mafongoya PL, McDermott MH, Agumya A. Agroforestry Research and Development in Southern Africa During the 1990s: Review and Challenges Ahead. Agroforestry Syst (2003) 59(3):173–86. doi: 10.1023/B:AGFO.0000005222.68054.38
33. Dickinson NM, Baker AJM, Doronila A, Laidlaw S, Reeves RD. Phytoremediation of Inorganics: Realism and Synergies. Int J Phytorem. (2009) 11:97–114. doi: 10.1080/15226510802378368
34. Kitobo WS. Dépollution Et Valorisation Des Rejets Miniers Sulfurés Du Katanga. Cas Des Tailings De L’ancien Concentrateur De Kipushi. Université de Liège: Thèse de doctorat. Faculté des Sciences Appliquées (2009). 254p.
35. Shutcha MN, Mukobo RP, Muyumba DK, Mubemba MM, Faucon MP, Kimbamba TL, et al. Fond Pédogéochimique Et Cartographie Des Pollutions Des Sols À Lubumbashi. In: Boaert J, Collinet G, Mahy G, editors. Anthropisation Des Paysages Katangais. Agronomie Gembloux: Presse Universitaire de Liège (2018). p. pp 215–228.
36. Duvigneaud P, Denaeyer-De Smet S. Cuivre Et Végétation Au Katanga. Bull Soc R Bot Belg. (1963) 96:92–231.
37. Leteinturier B. Evaluation Du Potential Phytocénotique Des Gisements Cuprifères D’Afrique Centro-Australe En Vue De La Phytoremédiation De Sites Pollués Par L’activité Minière. Gembloux: Faculté des Sciences Agronomiques de Gembloux (2002).
38. Ilunga wa Ilunga E, Séleck M, Colinet G, Faucon MP, Meerts P, Mahy G. Small-Scale Diversity of Plant Communities and Distribution of Species Niches on a Copper Rock Outcrop in Upper Katanga, DR Congo. Plant Ecol Evol (2013) 146(2):173–82. doi: 10.5091/plecevo.2013.816
39. Festin ES, Salk C, Tigabu M, Syampungani S, Odén PC. Biological Traits of Tropical Trees Suitable for Restoration of Copper-Polluted Lands. Ecological Engineering. (2019) 138:118–25. doi: 10.1016/j.ecoleng.2019.07.010
40. Yukselen MA. Characterization of Heavy Metal Contaminated Soils in Northern Cyprus. Environ Geology (2002) 42:597–603. doi: 10.1007/s00254-002-0556-5
41. Kien CN, Noi NV, Son LT, Ngoc HM, Tanaka S, Nishina T, et al. Heavy Metal Contamination of Agricultural Soils Around a Chromite Mine in Vietnam. Soil Sci Plant Nutr (2010) 56(2):344–56. doi: 10.1111/j.1747-0765.2010.00451.x
42. Shutcha MN. Phytostabilisation Des Sols Contaminés En Métaux Lourds Par L’activité Minière Au Katanga «Cas Du Quartier Gécamines/Penga Penga Contaminés En Métaux Par Les Émissions De La Fonderie De Cuivre De L’usine Gécamines/Lubumbashi». Université de Lubumbashi (2010).
43. Ali H, Khan E, Sajad MA. Phytoremediation of Heavy Metals—Concepts and Applications. Chemosphere (2013) 91(7):869–81. doi: 10.1016/j.chemosphere.2013.01.075
44. Madejón E, De Mora AP, Felipe E, Burgos P, Cabrera F. Soil Amendments Reduce Trace Element Solubility in a Contaminated Soil and Allow Regrowth of Natural Vegetation. Environ Pollut (2006) 139(1):40–52. doi: 10.1016/j.envpol.2005.04.034
45. Perrino EV, Brunetti G, Farrag K. Plant Communities in Multi-Metal Contaminated Soils: A Case Study in the National Park of Alta Murgia (Apulia Region-Southern Italy). Int J Phytoremem. (2014) 16(9):871–88. doi: 10.1080/15226514.2013.798626
46. Richardson DM, Rejmánek M. Trees and Shrubs as Invasive Alien Species–a Global Review. Diversity Distributions (2011) 17(5):788–809. doi: 10.1111/j.1472-4642.2011.00782.x
47. de Sousa Machado MT, Drummond JA, Barreto CG. Leucaena Leucocephala (Lam.) De Wit in Brazil: History of an Invasive Plant. Estudos Ibero-Americanos (2020) 46(1):e33976–6. doi: 10.1544/1980-864x.2020.1.33976
48. Manikandan M, Kannan V, Mahalingam K, Vimala A, Chun S. Phytoremediation Potential of Chromium-Containing Tannery Effluent-Contaminated Soil by Native Indian Timber-Yielding Tree Species. Preparative. Biochem Biotechnol (2016) 46(1):100–8. doi: 10.1080/10826068.2015.1045607
49. Kambing'a MK, Syampungani S. Performance of Tree Species Growing on Tailings Dam Soils in Zambia: A Basis for Selection of Species for Re-Vegetating Tailings Dams. J Environ Sci Eng (2012) 1(7B):827.
50. Bazihizina N, Redwan M, Taiti C, Giordano C, Monetti E, Masi E, et al. Root Based Responses Account for Psidium Guajava Survival at High Nickel Concentration. J Plant Physiol (2015) 174:137–46. doi: 10.1016/j.jplph.2014.10.011
51. Perlatti F, Ferreira TO, da Costa Roberto FA, Romero RE, Sartor LR, Otero XL. Trace Metal/Metalloid Concentrations in Waste Rock, Soils and Spontaneous Plants in the Surroundings of an Abandoned Mine in Semi-Arid NE-Brazil. Environ Earth Sci (2015) 74(6):5427–41. doi: 10.1007/s12665-015-4556-7
52. Remon E, Bouchardon JL, Cornier B, Guy B, Leclerc JC, Faure O. Soil Characteristics, Heavy Metal Availability and Vegetation Recovery at a Former Metallurgical Landfill: Implications in Risk Assessment and Site Restoration. Environ Pollut (2005) 137(2):316–23. doi: 10.1016/j.envpol.2005.01.012
53. Escarré J, Lefèbvre C, Raboyeau S, Dossantos A, Gruber W, Marel JCC, et al. Heavy Metal Concentration Survey in Soils and Plants of the Les Malines Mining District (Southern France): Implications for Soil Restoration. Water Air Soil Pollut (2011) 216(1):485–504. doi: 10.1007/s11270-010-0547-1
54. Córdova S, Neaman A, González I, Ginocchio R, Fine P. The Effect of Lime and Compost Amendments on the Potential for the Revegetation of Metal-Polluted, Acidic Soils. Geoderma (2011) 166(1):135–44. doi: 10.1016/j.geoderma.2011.07.022
55. Prach K, Pyšek P. Using Spontaneous Succession for Restoration of Human-Disturbed Habitats: Experience From Central Europe. Ecol Eng (2001) 17(1):55–62. doi: 10.1016/S0925-8574(00)00132-4
56. Mensah AK. Role of Revegetation in Restoring Fertility of Degraded Mined Soils in Ghana: A Review. Int J Biodiversity Conserv (2015) 7(2):57–80. doi: 10.5897/IJBC 2014.0775
57. Yang J, Tang L, Guan YL, Sun WB. Genetic Diversity of an Alien Invasive Plant Mexican Sunflower (Tithonia Diversifolia) in China. Weed Sci (2012) 60(4):552–7. doi: 10.1614/WS-D-11-00175.1
58. Gooden B, French K, Turner PJ, Downey PO. Impact Threshold for an Alien Plant Invader, Lantana camara L., on Native Plant Communities. Biol Conserv (2009) 142(11):2631–41. doi: 10.1016/j.biocon.2009.06.012
59. Shutcha MN, Mpundu MM, Faucon MP, Ngongo ML, Visser M, Collinet G, et al. Phytostabilisation of Copper-Contaminated Soil in Katanga: An Experiment With Three Native Grasses and Two Amendments. Int J Phytorem (2010) 12:616–32. doi: 10.1080/15226510903390411
60. Baker AJ. Accumulators and Excluders-Strategies in the Response of Plants to Heavy Metals. J Plant Nutr (1981) 3(1-4):643–54. doi: 10.1080/01904168109362867
61. Gómez-Sagasti MT, Alkorta I, Becerril JM, Epelde L, Anza M, Garbisu C. Microbial Monitoring of the Recovery of Soil Quality During Heavy Metal Phytoremediation. Water Air Soil pollut (2012) 223(6):3249–62. doi: 10.1007/s11270-012-1106-8
62. Oliva SR, Mingorance MD. Assessment of Airborne Heavy Metal Pollution by Aboveground Plant Parts. Chemosphere (2006) 65(2):177–82. doi: 10.1016/j.chemosphere.2006.03.003
63. Rana V, Maiti SK. Differential Distribution of Metals in Tree Tissues Growing on Reclaimed Coal Mine Overburden Dumps, Jharia Coal Field (India). Environ Sci pollut Res (2018) 25(10):9745–58. doi: 10.1007/s11356-018-1254-5
64. Baker AJ, Brooks R. Terrestrial Higher Plants Which Hyperaccumulate Metallic Elements. A Review of Their Distribution, Ecology and Phytochemistry. Biorecovery (1989) 1(2):81–126. doi: 10.1080/01904168109362867
65. Tomašević M, Aničić M, Jovanović L, Perić-Grujić A, Ristić M. Deciduous Tree Leaves in Trace Elements Biomonitoring: A Contribution to Methodology. Ecol Indic (2011) 11(6):1689–95. doi: 10.1016/j.ecolind.2011.04.017
66. Madeira AC, De Varennes A, Abreu MM, Esteves C, Magalhães MCF. Tomato and Parsley Growth, Arsenic Uptake and Translocation in a Contaminated Amended Soil. J Geochemical Explor (2012) 123:114–21. doi: 10.1016/j.gexplo.2012.04.004
67. Laureysens I, Blust R, De Temmerman L, Lemmens C, Ceulemans R. Clonal Variation in Heavy Metal Accumulation and Biomass Production in a Poplar Coppice Culture: I. Seasonal Variation in Leaf, Wood and Bark Concentrations. Environ Pollut (2004) 131(3):485–94. doi: 10.1016/j.envpol.2016.07.021
68. Commission of the European Communities. Commission Decision of 3 November 2006 Establishing Revised Ecological Criteria and the Related Assessment and Verification Requirements for the Award of the Community Eco-Label to Soil Improvers. Off J Euro. Union. L. (2006) 325:28–34.
Keywords: tree species, trace metals, reclaimed area, spontaneous vegetation, charcoal
Citation: Mwanasomwe JK, Langunu S, Shutcha MN and Colinet G (2022) Effects of 15-Year-Old Plantation on Soil Conditions, Spontaneous Vegetation, and the Trace Metal Content in Wood Products at Kipushi Tailings Dam. Front. Soil Sci. 2:934491. doi: 10.3389/fsoil.2022.934491
Received: 02 May 2022; Accepted: 02 June 2022;
Published: 07 July 2022.
Edited by:
Luís Miguel Nunes, University of Algarve, PortugalReviewed by:
Shamsollah Ayoubi, Isfahan University of Technology, IranCopyright © 2022 Mwanasomwe, Langunu, Shutcha and Colinet. This is an open-access article distributed under the terms of the Creative Commons Attribution License (CC BY). The use, distribution or reproduction in other forums is permitted, provided the original author(s) and the copyright owner(s) are credited and that the original publication in this journal is cited, in accordance with accepted academic practice. No use, distribution or reproduction is permitted which does not comply with these terms.
*Correspondence: Jacques Kilela Mwanasomwe, amtpbGVsYUB1bGllZ2UuYmU=; Gilles Colinet, R2lsbGVzLkNvbGluZXRAdWxpZWdlLmJl
Disclaimer: All claims expressed in this article are solely those of the authors and do not necessarily represent those of their affiliated organizations, or those of the publisher, the editors and the reviewers. Any product that may be evaluated in this article or claim that may be made by its manufacturer is not guaranteed or endorsed by the publisher.
Research integrity at Frontiers
Learn more about the work of our research integrity team to safeguard the quality of each article we publish.