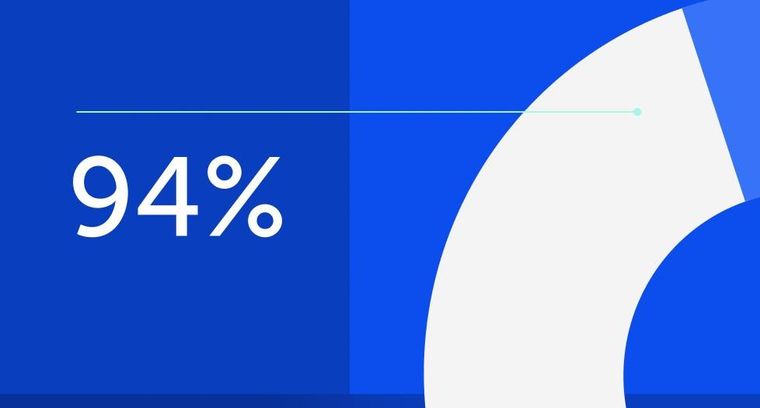
94% of researchers rate our articles as excellent or good
Learn more about the work of our research integrity team to safeguard the quality of each article we publish.
Find out more
REVIEW article
Front. Soil Sci., 21 February 2022
Sec. Soil Biology, Ecosystems and Biodiversity
Volume 2 - 2022 | https://doi.org/10.3389/fsoil.2022.833181
This article is part of the Research TopicMicrobiome-based Technologies: use of inoculants for improving agricultural productivity and sustainabilityView all 5 articles
The use of fertilizers and chemical pesticides promotes significant improvements in crop development, but some problems and risks associated with them limit their application. An alternative is using biological inputs based on microorganisms, increasing production while combining efficiency and sustainability. Actinomycetes are a group of bacteria belonging to the phylum Actinobacteria, recently re-named Actinobacteriota. They represent important microbial communities in the soils with increasing agricultural applications, especially in the biological control of insect-pest and plant disease and in plant growth promotion. Studies report their promising use as microbiological inoculants by exploring mechanisms to improve plant development, such as biological nitrogen fixation, phosphate solubilization, production of phytohormones, and other biocompounds. In addition, many species produce metabolic pathways that generate high-value antibiotics, extracellular enzymes and secondary metabolites other than antimicrobials, with potential in the control of phytopathogenic fungi, insects, and nematodes. These actinomycetes could be used to formulate novel bioinoculants composed of spores and/or mycelium. Considering that the research in this field is up-and-coming, with significant economic and environmental impacts in the future, this review aims to group the most relevant works that explore the biodiversity of actinomycetes, helping to develop inoculants and biodefensives for more productive and conscious agriculture.
The technological development of agriculture comes with several problems and challenges. The main ones are related to the guarantee of high production rates in a global scenario with the increasing scarcity of resources and environmental degradation (1). Some estimates suggest that the need to produce agricultural sources will grow up to 70% by 2050 (2). This high demand for productivity has resulted in the search for strategies that ensure good plant performance and help optimal develop economically important crops (3). To increase plant nutrition and protection, chemical fertilizers and pesticides are commonly applied. However, when misused, these products tend to accumulate in nature and promote eutrophication of water bodies, besides the presence of compounds that may be toxic to human health at overexposure (4, 5).
Currently, producers and consumers have been gaining more ecological and food awareness. This phenomenon is consistent with the market trend of prioritizing the use of more sustainable products, aiming to reduce waste and decrease impacts on nature through the search for biodegradable, safe, and effective replacements (6). In this sense, microorganism-based products work as an efficient alternative for reducing the use of agrochemicals, combining high productivity with a responsible view of the planet and human health (7). The demand for microbial inoculants, or biofertilizers, has increased considerably in recent decades, being used both as a substitute for conventional fertilizers and as an additional and complementary resource to existing ones (8, 9). Biopesticides based on microbial biological control agents (MBCAs) show excellent results against phytopathogens of agricultural importance (10), especially due to the high specificity of targets provided by their natural molecular mechanisms, able to decrease pest population and reestablish the ecological balance of the environment (11, 12).
Among the many varieties of microorganisms that can be part of a product for agricultural application, actinomycetes stand out for their bioactive particularities (13). These are bacteria primarily found in soil with biologically important properties, capable of producing numerous metabolites of commercial interest, such as enzymes, hormones, and antibiotics (14). These compounds are generally products of secondary metabolism, which are not used in the vital stages of their development and reproduction (14).
The area surrounding plant roots, called rhizosphere, is a space richly colonized by actinomycetes. Their high synthesis of antimicrobial compounds and other metabolites gives them a competitive advantage, besides helping with the improvement of soil fertility by promoting plant growth, providing protection against phytopathogens and resisting environmental stresses (15, 16). Bacterial endophytes are other important class of microorganisms that help the host develop while using nutrients and shelter provided by the plant cells. They constitute a group of non-pathogenic bacteria capable of colonizing the interior of plant tissues, either inside the vegetable cells or between them (17). Endophytic actinomycetes produce secondary metabolites that establish beneficial relations with the plant host by protecting them from phytopathogens and stimulating plant growth, which further demonstrates their promising role in agricultural management (18). However, despite the biotechnological potential of actinomycetes, only a few products on the market take advantage of the microorganisms' abilities and a small number of bibliographic studies on the subject.
Therefore, this review aims to elucidate some of the main aspects involving the study of actinomycetes and their applications as inoculants and biodefensives in agriculture. The mechanisms behind the bioactivity of actinomycetes capable of promoting plant growth and protection, especially rhizospheric and endophytic species already characterized in the literature, are described. Furthermore, the main challenges behind the large-scale production of actinomycetes, the achievement of stable formulations and the different forms of application in the field are listed. The strategies adopted to improve this field of research are also listed, in order to contribute to the development of a more sustainable and productive agriculture.
Actinomycetes represent one of the largest and most diverse bacterial groups described (19). They are Gram-positive bacteria belonging to the order Actinomycetales and the phylum Actinobacteria, being cosmopolitan microorganisms with habitats in both terrestrial and marine aquatic and freshwater environments (20). Its genome is enriched in G+C content. Its morphology varies from cocci and rods to complex multicellular structures similar to the mycelium of filamentous fungi, as shown in Figure 1. The mycelial structures can be aerial or adhered to the substrate (22, 23). This characteristic, together with the fact that some individuals produce spores as a form of asexual reproduction, has already led actinomycetes to be considered as transitional microorganisms between bacteria and fungi. However, actinomycetes' genetic and cellular profiles are characteristic of bacterial attributes, such as a chromosome wrapped in a prokaryotic nucleoid and a cell wall composed of peptidoglycans (20, 24).
Figure 1. Streptomycetes isolated from Brazilian medicinal plants (21) cultivated in IPS2 medium (agar 20.0 g/L, malt extract 10.0 g/L, dextrose 4.0 g/L, yeast extract 4.0 g/L, and distilled water) (A,B) and its microscopic characteristics in optical (C) and atomic force microscopy (D). Source: Photos by Dr. F. P. N. Cruz—Department of Morphology and Pathology, Federal University of São Carlos, São Carlos, Brazil.
The potential use of actinomycetes as a source of bioproducts began in the 1920's when the first report of antibiosis by a species belonging to this group was reported. Later, in the 1940's, the first molecules with antibiotic properties produced by actinomycetes were isolated, including streptomycin, which would become the first active treatment against tuberculosis (25). Currently, it is estimated that 64% of all naturally produced antibiotics come from actinomycete species (26). In fact, the number of published articles related to actinomycetes has increased considerably since the beginning of the last decade, especially the ones focused on species capable of promoting plant growth and biocontrol (Figure 2). This behavior is in line with global trends of studying microbial biodiversity for the development of biotechnological solutions.
Figure 2. Number of papers published in the period from 2011 to 2021 regarding (A) actinomycetes and (B) plant-growth promoting actinomycetes and biocontrol actinomycetes, found on Scopus database.
Studies have already demonstrated actinomycetes metabolites active against bacteria (27), fungi (28), viruses (29), nematodes (30), and insects (31), as well as compounds with antitumor (32) and herbicide (33) activities. Some species can also produce enzymes of industrial interest, such as chitinases, cellulases, and amylases (34), and enzymes involved in plant growth promotion, like phosphatases and nitrogenases (35). In addition, actinomycetes also produce volatile organic compounds (VOCs), playing a significant role in their bioactivity, acting as antimicrobial agents, and even inducing changes in the gene expression of nearby microorganisms (36).
Actinomycetes are primarily found in soil, where they represent more than 30% of the entire microbial population and can vary from 106 to 109 bacteria per gram (24, 37, 38). In order to survive in this environment, actinomycetes developed intricate mechanisms mainly for the decomposition of organic matter by producing enzymes through which they could break soil components and obtain the nutrition required for survival (39). Cellulose and lignin, found in wood residues, and the chitin found in the exoskeletons of insects, are some of the substrates that actinomycetes enzymes are capable of hydrolyzing (34). In this process, the nutrients released become bioavailable for plants and other organisms present in the soil (39).
Actinomycetes have a strong capacity of colonizing the region near plant roots, called the rhizosphere. This area has higher nutritional values due to the roots' exudation, secretion, and deposition of important compounds (e.g., amino acids, fatty acids, organic acids, phenols, sterols, sugars, and vitamins) (37). For this reason, actinomycetes that colonize the rhizosphere are more likely to obtain nutrients, which explains the higher bacterial concentration in this region when compared to bulk soil (40). Among the various plant species that actinomycetes can colonize there are some economically important crops, such as soybean (41), tomato (42), wheat (43), and pea and chickpea (44).
Streptomyces is the most common soil actinomycetes, and some studies suggest that this genus represents more than 95% of the total actinobacteria found in the soil (37). However, other genera have already been reported as rhizoactinomycetes, like Sanguibacter, Rhodococcus, Pseudonocardia, Propionibacterium, Nocardia, Mycobacterium, Micrococcus, Microbacterium, Frankia, Corynebacterium, Clavibacter, Cellulomonas, Arthrobacter, and Actinomyces (45, 46).
Regarding important interactions among bacteria and plants, endophytic actinomycetes are another promising biotechnological source found in nature (13). They constitute a group of non-pathogenic microorganisms that colonize the interior of plant tissues during its entire or partial life cycle, from roots to aerial structures (17).
Actinobacteria is one of the most common classes of endophytic bacteria (47). The actinomycetes endophytes genera already reported are Actinomadura, Actinoplanes, Dietzia, Glycomyces, Kineosporia, Leifsonia. Microbispora, Micrococcus, Micromonospora, Nocardia, Nocardioides, Pseudonocardia, Saccharopolyspora, Streptomyces, and Streptosporangium, among others (48, 49).
Plant growth-promoting bacteria (PGPB) are the most used microorganisms in the composition of inoculants, especially those that colonize the rhizospheric region of the plant, called plant growth-promoting rhizobacteria [PGPR; (50)]. Associations between plants and plant-growth-promoting actinomycetes (PGPA) have been extensively studied over the years. In general terms, the PGPA with the potential to constitute microbial inoculants can benefit the host plant through two main strategies: phytohormones modulation (synthesis of auxins, gibberellins, cytokines, and production of 1-aminocyclopropane-1-carboxylate (ACC) deaminase) and increase in nutrients bioavailability (biological nitrogen fixation, phosphate solubilization and production of siderophores) (Figure 3).
Figure 3. Main mechanisms of action of plant-growth promoting bacteria (PGPB; Figure by authors). Created with BioRender.com.
Producing phytohormones that stimulate plant growth is recurrent in many PGPA species. They are, in most cases, indole compounds belonging to the class of auxins, with indole-3-acetic acid (IAA) being the most common (51). Harikrishnan et al. (52) conducted a study in which phytohormone production by 90 isolated actinomycetes was evaluated, of which 65 tested positive. In similar work, Gopalakrishnan et al. (53) quantified the production of several biomolecules involved in plant growth promotion and biocontrol by six strains of the genus Streptomyces. The ability to synthesize IAA is common among actinomycetes, particularly endophytic and rhizospheric species of the genus Streptomyces (54–56). The PGPA that produce IAA tend to promote more significant root growth of the plants associated with it, increasing their access to soil nutrients and improving their development behavior (57).
All bacteria tested positive for IAA production, and two strains (CAI-95 and CAI-93) stood out for promoting greater root development and productivity when inoculated in rice plants. The real-time quantitative PCR test (qRT-PCR) showed an up-regulation of genes involved in producing IAA of these two strains, which explains their greater bioactivity. Additional studies have reported IAA synthesis by other species, such as S. rosealbus (58), S. scabiei (59), and S. roseoflavus (60).
Besides Streptomyces, other genera of actinomycetes also can produce IAA, as reported in a study by Shutsrirung et al. (61). IAA synthesizing species belonging to the genera Spirillospora, Streptomyces, Nocardia, Nocardiopsis, Microbispora, and Micromonospora were identified in this work. All six genera had microorganisms with positive results for phytohormone production. The actinomycetes of the genus Nocardiopsis showed expressively high levels of IAA production (from 62.23 to 222.75 μg/mL). Nimnoi et al. (62) identified species of the genera Nonomuraea, Actinomadura, and Pseudonocardia who also had significant IAA production rates (from 9.85 to 12.07 μg/mL).
Many inoculants have more than one microorganism in their composition, aiming to achieve a synergistic effect that enhances different bioactive mechanisms and increases the product's spectrum of action (63). The endophytic actinomycete S. griseoflavus is an IAA-producing species with great potential to promote plant growth. Its association with other PGPB in the development of biofertilizers has been drawing attention in recent years (64). Htwe et al. (65) reported that the combination of S. griseoflavus P4 with two distinct species of Bradyrhizobium promotes a series of beneficial increases to soybeans and mung beans, such as root elongation, more significant plant growth, improved capture of N, P, and K, in addition to a higher yield in the production of soybean seeds. Similarly, a study conducted by Soe et al. (64) demonstrated that co-inoculation of B. yuanmingense with S. griseoflavus P4 results in a symbiotic and synergistic relationship between the two bacteria, which induces greater nodule formation in the roots of soybean plants and increases biological nitrogen fixation rates. This behavior may be related to the improvement in plant performance caused by the phytohormone produced by the actinomycete, directly affecting the production of nodules and NBF by the nodulating bacteria.
Gibberellin is another class of phytohormones that can promote plant growth (66). They are tetracyclic diterpenoid carboxylic acids with C20 or C19 structures, affecting important vegetable growth processes, such as stem elongation, seed germination, flowering, and fruit setting, improving photosynthesis rate and chlorophyll content (57). Synthesis of gibberellins is less recurrent in actinomycetes, but some species have already been reported as producers of these phytohormones. The production of gibberellic acid (GA) by four actinomycetes of four different genera (Frankia, Streptomyces, Actinoplanes, and Micromonospora) was investigated by Solans et al. (67), and the results were positive for all bacteria, showing concentrations values higher than the ones for IAA production for three strains, except Micromonospora sp. GA synthesis was also reported for A. campanulatus, S. spiralis, S. olivaceoviridis, and S. rochei (68, 69).
Cytokinins are phytohormones that play an important role in plant development, modulating seed germination, plant senescence, photosynthesis, and respiration (70). Few studies relate actinomycetes with the ability to produce cytokinins. Still, it has already been demonstrated that the genus Actinoplanes, Frankia, Streptomyces, and Micromonospora can synthesize zeatine, one of the most well-known cytokinins (67).
Ethylene, a phytohormone involved in the regulation of plant development, plays a crucial role in maintaining healthy growth at low concentration levels (~0.05 μL/L) (71). Many PGPA can produce ACC deaminase, contributing to greater control of ethylene levels and plant growth (72). In addition, this enzyme can promote aerial and root elongation of plants (73).
The investigation of ACC deaminase-producing rhizosphere actinomycetes was done by Anwar et al. (74). Six strains of Streptomyces were found capable of producing the enzyme at concentrations ranging from 1.9 to 0.71 mmol/L. Species of the genera Microbacterium (75), Actinobacterium (76), Micrococcus (77), and Rhodococcus (78) have already shown the potential of ACC deaminase production.
The importance of the interaction of ACC deaminase and IAA in the inoculation of plant-growth-promoting actinomycetes was evidenced by El-Tarabily (42). In this work, strains of Streptomyces isolated from tomato rhizosphere were tested for both productions of ACC deaminase and IAA, along with evaluating their competence as root colonizers. A strain identified as S. filipinensis no. 15 produced the enzyme and the phytohormone, while the isolate S. atrovirens no. 26 produced only ACC deaminase. Both bacteria were rhizosphere-competent. When inoculated in tomato plants in greenhouse conditions, both actinomycetes induced a decrease of the endogenous levels of ACC, a precursor in ethylene biosynthesis pathway, and promoted plant growth. However, the increase in plant growth was higher in the treatment with S. filipinensis no. 15, which may be related to its ability to produce IAA together with ACC deaminase. In addition, when a strain, not rhizosphere-competent producing ACC deaminase, was inoculated under the same conditions, the treatment did not result in plant-growth promotion. These findings show that, besides the connection between ACC deaminase and IAA, it is also important to look at the ability of the strains to colonize the rhizosphere to achieve a successful inoculation.
Nitrogen (N) is found in the atmosphere as a gas (N2), but plants are not capable of assimilating it in this form. The biological nitrogen fixation (BNF) process turns it into NH4+, making the nutrient available for use in the biosynthesis of vital molecules in vegetable cells (79, 80). PGPB species capable of BNF are called diazotrophic bacteria, capable of forming N-fixing nodules in the roots of the plants when imposed to nitrogen-deficient environments (79–81).
Several studies have focused on bioprospecting diazotrophic actinomycetes. However, a small number of species have been reported to own the ability of BNF. The species belonging to the genus Frankia and their capacity to induce the formation of N2-fixing nodules on more than 200 species of dicotyledonous angiosperms, called actinorhizal plants (82). These diazotrophic actinomycetes can be found in a symbiotic association with actinorhizal plants or as free-living N2-fixing bacteria (83). Their mechanism of N2 fixation involves the expression of nif genes and the development of vesicles named diazovesicles when exposed to a nitrogen-deprived environment, where the nitrogenase complex is protected from oxygen by laminated lipid layers (81, 84). Frankia was considered the only N-fixing representative group among actinomycetes for a long time. However, some studies in the past decades reported non-Frankia diazotrophic species belonging to the genus Streptomyces, Mycobacterium, Corynebacterium, Microbacterium, Agromyces, and Micromonospora (85).
Streptomyces thermoautotrophicus was the first diazotrophic streptomycete described (86). On the other hand, more recent studies suggest a reclassification of the bacteria since the reported genes related to nitrogenase enzymes have similarities in genomes of non-diazotrophic species (87). Dahal et al. (88) also showed species of Streptomyces capable of biological nitrogen fixation. This study demonstrated that not all strains that can grow in a nitrogen-free culture medium necessarily have the nifH genes. Of 46 Streptomyces isolated from soil and capable of growing under nitrogen-free conditions, only four strains presented gene clusters responsible for the nitrogenase complex. This shows that other gene clusters are also related to BNF (89). Wahyudi et al. (41) evaluated the biological nitrogen fixation capacity of 18 rhizospheric Streptomyces sp, isolated from the soybean (Glycine max L.) rhizosphere. 15 strains showed positive results for growth in N-free culture medium in vitro. The best results were S. panaciradicis, S. recifenses, S. polychromogenes, and S. manipurensis. They were tested in vivo on soybean, inducing a visibly, more significant growth in all treatments after 5 days of inoculation compared to controls.
Actinomycetes can also act as Rhizobia Helper Bacteria (RHB), agents that interact with natural N-fixing microbes and improve bioactivity. A strain of Micromonospora isolated from nitrogen-fixing nodules of alfalfa (Medicago sativa L.) improved plant-growth of alfalfa when associated with the rhizobium Ensifer meliloti 1021, as reported by Martínez-Hidalgo et al. (90). The results indicated that the interactions between bacteria increased roots nodulation and nitrogen nutrition by the plant, besides promoting aerial growth, a higher shoot-to-root ratio, and a raise in the level of essential nutrients, indicating its potential as an indirect plant-growth promoter.
Phosphate solubilization is a trait found among some genus of actinomycetes, such as Streptomyces, Microbacterium, Thermobifida, Angustibacter, Kocuria, Isoptericola, and Agromyces (91, 92). The exploration of this ability usually comprises bioprospecting in mines or rocky regions, where phosphate-solubilizing bacteria are most likely to occur in the soil. Phosphorus (P) is an essential element for good plant performance, being involved in functions ranging from cellular energy metabolism to the transfer of genetic characteristics through generations (50). In nature, inorganic and organic forms of phosphorus available in the soil are not assimilated by plants. To make them accessible, some plant-associated bacteria can solubilize inorganic phosphates by producing low molecular weight acids (93).
Hamdali et al. (94) screened 300 actinomycetes isolated from Moroccan phosphate mines, an area rich in insoluble rock phosphate (RP), and 18.3% of the strains were able to solubilize rock phosphate as the only phosphate source in a minimal medium. Eight selected strains from the genus Streptomyces and Micromonospora, showed mechanisms of phosphate solubilization involving siderophores production.
Aallam et al. (95) tested the ability of 10 isolates of the genus Streptomyces to use two sources of phosphate in the soil: natural rock phosphate (RP) and tricalcium phosphate (TCP). Species identified as S. bellus and S. enissocaesilis used both forms of phosphorus for their development and released the excess as soluble P in the medium at concentrations ranging from 60 to 170 μg/mL. In the same work, preliminary studies indicated that the phosphate solubilization by S. enissocaesilis is probably related to the acidification of the medium and the production of siderophores. A similar result was found by Farhat et al. (96). They identified an actinomycete (Streptomyces sp. CTM396) capable of mineral phosphate solubilization (MPS) through a mechanism that involves the expression of the genes gdh and pqq, responsible for encoding precursors in the pathway of gluconic acid, which is stimulated by the presence of humic acids. The decrease in the pH is followed by MPS, making phosphate available for plant uptake.
The effect of phosphate-solubilizing actinomycetes on plant growth and fitness was explored by Hamdali et al. (97). In this study, the phosphate-solubilizing strains Micromonospora aurantiaca and S. griseus were inoculated in seeds of the wheat plant (Triticum durum L. cv. Vitron) grown under greenhouse conditions, with sterile soil deficient in forms of soluble phosphate supplemented or not with soluble phosphate or with insoluble RP. The treatments of both actinomycetes in the soil with insoluble RP increased plant growth, with 80–78% and 50–47% weight increase of roots and shoots, respectively. This result was associated with a higher N and P content of the plant's tissue. El-Tarabily et al. (98) isolated a strain of M. endolithica from a calcareous soil deficient in available phosphorus capable of solubilizing powdered rock phosphate (PRP) along with the production of organic acids. When inoculated in plants of beans (Phaseolus vulgaris L.) grown in soil with single super-phosphate (SP) or PRP, the growth of shoots and roots significantly increased when compared to the controls, which was in accordance with the high levels of nutrients available in the soil and the tissues of the plants inoculated with the actinomycete. The inability of the strain to produce phytohormones instigated the correlation of phosphate solubilization with the stimulation of plant growth.
Siderophores are low molecular weight molecules produced by microorganisms that can chelate Fe3+ ions present in the environment (50, 99). Siderophore-producing microorganisms have competitive advantages in environments where there is a low occurrence of iron. Under these conditions, the ability to capture Fe3+ ions prioritizes the survival of these species over other components of the local microbiota, including phytopathogenic microorganisms, which can suffer losses and cause less damage (100).
Several species of actinomycetes are capable of synthesizing siderophores compounds. Lee et al. (101) evaluated the pattern of siderophore production by actinomycetes isolated from two different sites in Western Australia. The results indicated that all the isolates had a similar percentage of synthesis of general siderophores classes, being catechol the only one to show a distinguished proportion (3% of the isolates from site 1 and 17% from site 2). A strain identified as belonging to the genus Streptomyces produced an extracellularly excreted enterobactin, an important siderophore known to be common in the Enterobacteriaceae family. Further PCR analysis demonstrated that the strain had in its genome the entF gene, responsible for the final assembly of the tri-cyclic structure of enterobactin. A second Streptomyces isolated showed the ability to produce heterobactin, a siderophore molecule also found in species of the genus Rhodococcus and Nocardia.
Desferrioxamine siderophores are one of the most common classes of siderophores produced by actinomycetes, being reported in the metabolome of more than 10 species of Streptomyces (102). Desferrioxamine B, in particular, is an important siderophore produced by S. pilosus with pharmacological properties, used as a drug in the treatment of iron intoxication (103). Nocardichelins A and B are siderophores produced by species of the genus Nocardia discovered by Schneider et al. (104), capable of inhibiting human cell lines from gastric adenocarcinoma, breast, and hepatocellular carcinoma. These bioactive compounds combine the structure of mycobactin-type siderophores from mycobacteria and desferrioxamine B produced by Streptomycetes.
In order to investigate the potential influence of siderophore production on plant-growth promotion, Rungin et al. (105) inactivated the desD-like gene of the endophytic Streptomyces sp. GMKU 3100, responsible for encoding a controlling enzyme involved in the final step of a siderophore biosynthesis, inoculated both the mutant and wild strain on plants of rice and mungbean. The treatments with the wild types had the best plant development, showing a significant increase in root and shoot biomass and length. The results were even better when the wild type was exposed to ferric citrate during inoculation on both plants, indicating a key function of siderophores in improving plant growth. Similar results were found by Verma et al. (54), who isolated endophytic actinomycetes from Azadirachta indica A. Juss. and tested them for plant-growth promotion and biocontrol agents. The strain Streptomyces AzR-051 was identified as a siderophore-producing actinomycete, capable of significantly promoting plant growth and antagonizing the phytopathogen Alternaria alternata when inoculated in seeds of the tomato.
The siderophore expression may also be related to the virulence of actinomycetes' pathogenic species, as reported by Miranda-CasoLuengo et al. (106). This work demonstrated that Rhodococcus equi produces a hydroxamate siderophore, rhequichelin, expressed by the gene cluster rhbABCDE, whose transcription increases during infection in the host tissue. In addition, when the genes were deleted, the levels of the strain virulence were low. This finding highlights that, although less common, there are important roles played by siderophores other than iron uptake and host defense.
Although they possess countless abilities related to plant growth promotion, only a few actinomycete-based biofertilizers are currently commercialized. Mizorin® is a biofertilizer based on actinomycetes used in corn, beetroot, and mustard (107). Bamil® and Omug® are also microbial inoculants based on actinomycetes (Micrococcus spp.), acting by increasing the nitrification process in wheat, beetroot, onion, carrot, potato, and marrow (108). The small amount of products available on the market is indirectly proportional to the potential of bioactive biodiversity of actinomycetes, which further demonstrates the importance of studies aimed at developing microbial inoculants that take advantage of this wasted potential.
Agriculture is constantly affected by the presence of pests, which are undesirable organisms that colonize crops and cause a disbalance in plant health and productivity (109). They constitute a varied group of organisms, being insects the most common (110). Plant-parasitic nematodes and phytopathogenic microorganisms, such as fungi, bacteria, and viruses, are also considered agricultural pests of significant impacts in production, capable of causing diseases that compromise plant performance (111, 112).
Usually, pest management in agriculture is made by using pesticides, substances, or mixtures of substances, whose chemical structures interact with unwanted organisms and decrease their levels and damages (113). Therefore, it is increasingly important to look for options that allow the reduction of chemical pesticides utilization, aiming to achieve crop productivity more safely and sustainably. In this sense, microorganisms with biological control abilities are a promising and efficient way of managing agricultural pests, from insects and nematodes to phytopathogenic fungi and bacteria (114). The microbial biological control agents (MBCAs) are the main constituents of biopesticides, and the main examples of actinomycetes with potential for being MBCAs, their mechanisms of action and targets are discussed in this topic.
MBCAs can act by indirect and direct mechanisms (Figure 4). The first type is characterized by controlling unwanted species without having direct contact with them (115), while the second happens when the unwanted species are directly affected by MBCAs (116). The indirect mechanism comprises the increase in plant resistance against pathogenic infections through activation of the systemic acquired resistance (SAR) and the induced systemic resistance (ISR) (11, 117). Competition for nutrients and space is also an indirect mode of action of biopesticide's MBCAs (115), in which a site's anticipated occupation by harmless microorganisms can prevent the plant from pathogens that have the same requirement for survival (118).
Figure 4. Main mechanisms of action of microbial biocontrol agents (MBCAs; Figure by authors). Created with BioRender.com.
Antibiosis is the most frequent type of direct biocontrol among actinomycetes, defined as a situation where the growth of a pathogen is compromised by toxic metabolites produced by an antagonistic presence (119, 120). Usually, these metabolites are antibiotics produced by bacteria as a defense mechanism, with chemical structures capable of inhibiting microbial cells' vital processes (121). Streptomyces is the actinomycete genus most known for antibiotics production, being responsible for synthesizing about 60% of all the antibiotics applied in agriculture and horticulture (122). Lytic enzymes are an important part of antibiosis biocontrol mechanisms (123), through which MBCAs can lyse vital structures of pathogen cells and inhibit their development (124). Other biocontrol direct mechanisms, like hyperparasitism (when an organism gains nutrients by colonizing a pathogen), are also potential interactions to be explored in formulations of biopesticides. However, they are more common among fungal species, with rare occurrences in bacteria (115).
Many MBCAs can either exhibit biocontrol abilities (direct or indirect) by protecting the plant against pathogen attacks and enabling better growth, or have PGPB traits that establish better plant development and make it fit to defend itself against harmful organisms (125, 126). In addition, the same microbial species can have both biocontrol mechanisms and PGPB traits, maximizing their interactions to prioritize plant protection and nutrition (126).
Biopesticides based on actinomycetes and their metabolites have already been developed and are commercialized to help control several pests in agriculture, such as fungus, insects, and nematodes (Table 1).
Fungal diseases are a major cause of agricultural crop losses, with species capable of infecting plants and soil and high ability to adapt under selection pressures (137, 138). In agriculture, this adaptable disposition turns them into effective plant pathogens with notable susceptibility for acquiring resistance to chemical pesticides (139). Furthermore, some phytopathogenic fungi are known as producers of mycotoxins highly noxious for humans, compromising food security (138).
Species belonging to the genus Streptomyces have been identified as strong biocontrol agents against both foliar and soil-borne fungal diseases caused by important agricultural pathogens, such as Phytophthora cinnamomi (140), Colletotrichum musae, Fusarium oxysporum (141), Botryts cinerea (142), Colletotrichum gloeosporioides (143), Fusarium moniliforme (144), Rhizoctonia solani, Fusarium solani, Fusarium verticillioides, Alternaria alternata (145), Sclerotinia sclerotiorum (146), Macrophomina phaseolina (147), Corynespora cassiicola (148), Pythium aphanidermatum (149), Penicillium digitatum (150), and others.
The ways through which Streptomyces control fungal diseases are diverse. Some species, like the rhizospheric S. lilacinus NRRL B-1968T, have in their genome the types I and II polyketide synthase genes (PKS-I and PKS-II), responsible for the pathway of fatty acids biosynthesis in some bacteria and encoding secondary metabolites with bioactive properties. In addition, this type of Streptomyces produces a varied amount of phenol, pyrrolizidine, hydrocarbons, esters, and acids compounds associated with fungus inhibition and cell membrane destruction (151). The non-ribosomal peptide synthetase (NRPS) gene clusters are also important players in the antibiosis of some actinomycetes against fungal phytopathogens, like S. triticiradiciss, isolated from rhizospheric soil of wheat (Triticum aestivum L.). The NRPS proteins can use non-proteinogenic amino acids as building blocks for producing peptides with multi structures and functions, including fungicide activity. Natamycin, an antibiotic used to treat fungal diseases, is also found as a bioactive compound in the metabolome of S. triticiradiciss (43).
Some other metabolites produced by Streptomyces have also been reported as active against phytopathogenic fungi, such as salvianolic acid B, able to cause damage to mycelial cells and spores of Alternaria spp., Fusarium spp., Colletotrichum spp., Cladosporium herbarum, and Botrytis cinerea (152) and 6-amino-5-nitrosopyrimidine-2,4-diol, a compound present in the crude extract of S. amritsarensis V31 with the ability to inhibit mycelium growth of R. solani (7.5–65%), A. alternata (5.5–52.7%), Aspergillu–s flavus (8–30.7%), F. oxysporum f. sp. lycopersici (25–44%), Sarocladium oryzae (11–55.5%), and S. sclerotiorum (29.7–40.5%) (153).
Streptomyces biocontrol of fungal diseases can also be accomplished by the secretion of extracellular lytic enzymes, such as chitinases, able to hydrolyze the chitin located in the fungal cell walls (154). Several chitinases with antifungal activity isolated from Streptomyces have already been described, like chitinase F (ChiF) from S. coelicolor A3(2), chitinase 30 (Chi30) from S. olivaceoviridis ATCC 11238, chitinase 35 (Chi35) from S. thermoviolaceus OPC-520, chitinase IS (ChiIS) from Streptomyces sp. MG3 and chitinase A (ChiA) from S. cyaneus SP-27 (155). Glucanases (156), cellulases (157), and proteases (158) are other enzymes produced by some Streptomyces involved in their antifungal abilities, capable of degrading vital fungal structures and limiting their growth.
Inderiati and Franco (159) identified non-streptomycete endophytic actinomycetes from the genus Actinomycetales, Microbispora, and Nonomuraea with considerable inhibition activity against A. solani, P. parasitica, R. solani, and P. irregulare, either in antagonistic assays performed in vitro and in vivo inoculation in tomato seeds. The genus Nocardia has already been associated with the biocontrol of A. brassicicola, P. dresclea, B. cinerea (160), and F. oxysporum (161). Endophytic actinomycetes belonging to the genus Saccharopolyspora and Actinopolyspora demonstrated strong antibiosis in vitro against A. brassicicola, Chaetomium globosum, F. oxysporum, P. dresclea, R. solani, and B. cinerea (160). A rare actinomycete, Saccharothrix yanglingensis, isolated from the cucumber roots, exhibited a high pattern of chitinase production, which was related to its antifungal activity against Valsa mali (162). Nocardiopsis prasina OPC-131 is another actinomycete that also produces a chitinase (ChiB) to control the growth of phytopathogenic fungi (163).
Arbuscular mycorrhizal fungi (AMF) are symbiotic fungi found in roots and soils of some plants. Their relationship with biocontrol promoting actinomycetes has been gaining attention in the last years. Reyes-Tena et al. (164) tested the synergistic effect of a mycorrhizal consortium with two strains of actinomycetes (ABV39 and ABV02) against Phytophthora capsici in pepper plants (Capsicum annuum L.). The results showed that the co-inoculation of the AMFs with the actinomycetes decreased the disease 3 points on a severity scale. Similar findings were observed by Sastrahidayat et al. (165) when inoculating soybean rhizosphere with mychorrhizal fungi and actinomycetes for the treatment of Sclerotium rolfsii. In this worl, the disease in the plants treated with both microrganisms was decreased up to 40% when compared to the controls.
In vivo performance of actinomycetes against fungal phytopathogens are often associated with positive results, demonstrating inhibiting effects that consolidate its possible application as constituents of biopesticides for plant defense (166–168). However, the in vitro behavior may not always be reflected when evaluating the antagonistic effect in vivo. Similarly, bacterial strains with low activity in vitro can show good inhibition results when applied in planta (169). This discrepancy in both types of screening may be related to the additional biotic and abiotic pressures that overcome or maximize microbial bioactivities, such as competition and other interactions between the actinomycete and the soil/foliar microbiota, climate changes (e.g., unstable temperature and humidity), the inability of proper colonization of the rhizosphere or the plant tissues and levels of nutrient bioavailability (169, 170). For this reason, the results in sterile environments and controlled parameters need to be corroborated in field conditions to assess the real performance of the studied microorganism (171).
Insects are the most biodiverse group of animals in the world, and their ability to feed on all kinds of organic matter, including plant tissues of important crops, has turn them into one of the main causes of agricultural losses and reduced yield (172, 173). Despite using traditional sustainable control strategies, such as crop rotation, healthy crop variety, and integrated pest management, insects are usually controlled by chemical insecticides (172). Although helpful, new alternatives are being seeked to prevent ecological damages and overcome insect resistance (174).
Actinomycetes are potential candidates due to their impressive synthesis of metabolites with insecticidal properties. The polyketide compounds are the main family of natural insecticides produced by actinomycetes, including avermectins, spinosyns, polynactins, tetramycins, and analogs (175).
Avermectins are found in the fermentation products of Streptomyces avermitilis and are widely commercialized for crop protection. Their molecular structure has a high ability of binding to muscular neurons of some insects, acting as agonists for γ-aminobutyric acid (GABA)-gated chloride channels, causing insect paralysis and death (176). They are active against several species from the orders Blattodea, Coleoptera, Diptera, Hymenoptera, Isoptera, Lepidoptera, and more (175). Abamectins and emamectins are insecticides derived from avermectins (176). Milbemycins are metabolites with different structures but act similarly to avermectins, produced by S. bingchenggensis (177). Recently, novel avermectins analogs have been tested to overcome insect resistance. Zhang et al. (178) evaluated the activity of more than 40 avermectin derivatives against Aphis craccivora, of which 4 had superior effect compared to avermectin (7–8 times better), with LC50 (lethal dose) ranging from 5.634 to 52.234 mM. The quantitative structure-activity relationships (QSARs) analysis suggest that the avermectin analogs activity is related to their branching degree, different molecular shape and size, and the number of double bonds.
Spinosyns comprise another important class of insecticidal metabolites produced by actinomycetes, specifically Saccharopolyspora spinosa. The most common are spinosyn A and spinosyn D, molecules with more specific action mechanisms and more focused targets, like Heliothis virescens and Spodoptera eridania (179). Spinosyns act by direct contact or ingestion, modifying GABA and the nicotinic receptor (176). Salgado (180) was the fisrt to report the mechanism through which spinosyn A acts, testing it against Drosophila melanogaster, Periplaneta amerina, Heliothis virescens, and Musca domestica. The LC50 was 8.0 ppm, capable of inducing involuntary muscle contractions and tremors by the excitation of insect's neurons, followed by paralysis due to neuromuscular fatigue.
Other classes of secondary metabolites with insecticidal activity can be found in actinomycetes. El-khawaga and Megahed (181) discovered the strain S. bikiniensis A11, isolated from sandy soil in Cairo, Egypt, capable of producing aminoglycosidic antibiotics active against larvae of cotton leaf worm (Spodoptera littoralis). In another study, Kim et al. (182) screened the ability of 363 actinomycetes to promote the death of Aedes albopictus and Plutella xylostella. Among them, the selected strain Streptomyces sp. AN120537 produced five different antimycins associated with its insecticidal activity. The authors suggested that the antimycins act as insect juvenile hormone antagonists (JHANs), compounds capable of promoting premature molting and larval stages by disrupting the endocrine system of insects. Liu et al. (183) isolated the strain Streptomyces sp. KN-0647 from forest soil in China identified the secondary metabolite quinomycin A, an antibiotic active against Spodoptera exigua, Dendrolimus punctatus, Plutella xylostella, Aphis glycines, and Culex pipiens. Some non-streptomycete actinomycetes have also demonstrated good results against insects, such as Micromonospora and Amycolatopsis (184).
As discussed before, actinomycetes demonstrate a high ability to produce lytic enzymes. Among them, chitinolytic species are important tools for controlling insect pests (185). Chitin is a major constituent of insects' cuticles, providing them with rigidity and shape while helping prevent moisture loss from the insect integument. Chitinase is an enzyme that can hydrolyse these chitin structures in insects, disrupting the skin that induces their death (176). Although lytic enzyme production is more often associated with fungal control, it has been demonstrated by Gadelhak et al. (185) that the chitinases produced by the chitinolytic actinomycetes Actinoplanes philippinensis, A. missouriensis, and S. clavuligerus have a role in the controlling mechanisms of Drosophila melanogaster. The application of A. missouriensi showed the lowest pulp formation percentage (31.75%), and the combinations of S. clavuligerus with A. philippinensis decreased the values to 27.35%, demonstrating a synergistic effect.
Plant-parasitic nematodes (PPNs) are soil-borne pathogens that disturb plant nutrition, compromising its physiological functions and resulting in productivity drops and economic losses (186). The endoparasitic PPNs are the most pathogenic ones for directly interfering in the absorption of water and nutrients by the plants. They comprise the most common nematodes found in crops, such as root-knot nematodes (Meloidogyne spp.), cyst nematodes (Heterodera spp., Globodera spp.), and pine wood nematode (Bursaphlenchus xylophilus) (187, 188). Management of PPNs is not easy, especially because of their hidden nature and difficulty in identifying and associating symptoms, which are not very specific. Usually, they are controlled by soil fumigation with chemical nematicides (189).
Liu et al. (190) screened 5,000 actinobacteria for nematicidal activity against the pine wood nematode (B. xylophilus), identifying the strain Streptomyces sp. AN091965 is the most active one. Further investigations found that the compound spectinabilin was responsible for the highest nematicidal activity against the nematode, with an LC50 value of 0.84 μg/mL, besides suppressing the disease in 5-year-old Pinus densiflora trees under greenhouse and field conditions. Sharma et al. (135) reported the nematicidal activity of the strain Streptomyces antibioticus M7 against root-knot nematode (M. incognita), which was associated with the production of actinomycins V, X2, and D, with LD50 values ranging from 28 to 120 μg/ml in vitro. Tomato plants treated with culture supernatant/cells/solvent extract displayed a reduction in root galls and egg masses and significantly enhanced plant growth measurements. In addition, when the strain was applied to non-infected plants, it showed a significant increase in many parameters of plant development, indicating Streptomyces antibioticus M7 as a potential nematicidal biopesticide and PGPB. Fungichromin B, a metabolite produced by S. albogriseolus HA10002, was discovered as a nematicidal agent by Zeng et al. (191). In this study, the compound was isolated and investigated against second-stage juveniles (J2) of M. incognita and M. javanica, achieving LC50 values of 7.64 and 7.83 μg/ml, respectively. Fervenulin, a low molecular weight compound, is a nematicidal metabolite produced by a strain of S. roseoverticillatus isolated and characterized by Ruanpanun et al. (192). In in vitro assays, the lowest minimum inhibitory concentrations (MICs) of the fervenulin against egg hatch of M. incognita was 30 μg/ml and J2 mortality was observed at 120 μg/ml.
Other metabolites produced by actinomycetes, such as volatile compounds, fatty acids, hydrogen sulfide, ammonia, alcohol, and phenolic compounds, have also been linked to nematicidal activity (193). Polyketide compounds produced by Streptomyces, such as avermectins and its derivate abamectin, are important nematicidal metabolites that constitute some of the main commercial biological nematicides (194).
The surface coat of nematodes (cuticle) and nematode egg shells targets this pest biocontrol. Their compositions are similar, with different layers of proteins, chitin, and lipids (135). Actinomycetes lytic enzymes, such as proteases, chitinases, and lipases, can degrade these structures and interfere with the motility and reproduction of nematodes, leading to their death (195, 196). Other lytic enzymes, like glucanases, cellulases, and pectinases, have also been reported to be involved in nematode biocontrol. However, their mechanisms of action are not well-elucidated yet (197).
Rashad et al. (193) identified 28 strains of Streptomyces with nematicidal activity against M. incognita, possibly associated with their ability to produce proteases, chitinases, and lipases. In another work, Yoon et al. (198) characterized the nematicidal metabolites from Streptomyces cacaoi GY525 and observed that the enzymes chitinase and glucanase presented in the bacterial culture filtrate are possibly related to the increased mortality of J2 of M. incognita and decreased eggs hatch. Furthermore, the secondary metabolite compound 3-benzyl-1,4-diaza-2,5-dioxobicyclo[4.3.0]nonane (BDDB) was also identified with nematicidal activity and the strain inoculation in tomato plants for 7 weeks decreased the number of egg masses and population of J2 in the soil.
The losses caused by phytopathogenic bacteria are usually related to crops of less economic impact (199). This factor can explain the scarcity of products based on actinomycetes to bacterial control diseases and why research in this field is occasionally related to the application in the control of post-harvest diseases of fruits and vegetables (200). However, the bactericidal activity found among actinomycetes is expressive and may be a good source for developing biocontrol strategies of phytopathogenic bacteria.
The strain Streptomyces cinereoruber can suppress infections caused by Erwinia chrysanthemi, the causal agent of the soft rot disease (201). S. misionensis, S. cacaoi subsp. Cacaoi, and S. puniceus are active against Xanthomonas campestris pv. campestris, the causal agent of the black rot disease (202). S. albidoflavus H12 and Nocardiopsis aegyptica H14 inhibit the growth of several bacterial phytopathogens, such as Pseudomonas syringae, P. corrugata, and Pectobacterium carotovorum subsp. carotovorum (203). S. ramulosus, S. axinellae, and S. drozdowiczii produce a crude extract active against Ralstonia solanacearum, the causal agent of bacterial wilt (204).
The antibacterial mechanisms of action are mainly related to the expression of antibiotic metabolites, like actinomycin (205), maklamicin (206), nomimicin (207), ansamycin (208), and much more. Encheva-Malinova et al. (209) tested 23 actinomycetes isolated from the Antarctic soils against phytopathogenic bacteria, finding that the most active strains inhibited Clavibacter michiganensis and five species of Xanthomonas. Molecular biology investigations showed that their genomes had combinations of the PKS-II, NRPS, and glycopeptides genes, possibly involved in the expression of antimicrobial metabolites.
Biofertilizers are usually inoculated in plants by three different methods: seed inoculation, consisting of the seeds immersion in a microbial solution of known concentration; soil inoculation, where the active solution is mixed directly in the soil subtracted or applied to the region near rhizosphere; and roots inoculation, in which the plant roots are immersed in the biofertilizer solution and then planted in the soil for its development (210). Seed inoculation provides the microorganism with nutrients from germination, which it uses to colonize the roots as soon as they are formed. On the other hand, inoculation directly into the roots or soil allows the bacteria to colonize the already developed roots. Inoculation in the roots allows an immediate contact of the microorganism with the plant, accelerating the colonization process (210, 211). The choice of inoculation method will depend on the plant species, the ease with which the actinomycete colonizes the plant roots and what stage of plant development is desired for the plant to receive the nutritional compounds of interest. In the case of endophytic species, the foliar application may also be considered (212).
The methods for biopesticides application can vary depending on the type of the product formulation. In general, formulations are designed to minimize additional costs over conventional chemical pesticide applications (213). In this sense, biopesticides are commonly applied in crops through basic spraying techniques, covering the aerial structures of plants and the soil (214).
Biofertilizers and most biopesticides are living products. Therefore, their success is entirely interconnected with the microbial response to biotic and abiotic factors present in the environment. Soil holds a wide range of biodiversity. The interaction between the actinomycete with surrounding microbiota and other organisms and its relation with the host plant may be explored to ensure product efficiency (210). Furthermore, actinomycetes can face severe changes in pH, temperature, salinity, moisture, light incidence, inorganic, and organic constituents of soil (215, 216). The stress caused by these factors can interfere in the rhizosphere and its constituents, causing modifications in actinomycete bioactivity regarding plant growth promotion and plant defense (217). Some species can tolerate these abiotic stresses by producing osmoregulating metabolites that prevent cell membrane plasmolysis and regulate enzymatic action and homeostasis (210). Some strains of Streptomyces and Nocardiopsis produce the osmoprotectants glycine betaine, proline, and trehalose at high osmolarity situations, agents that induce osmotic adaptation and preserve bioactivity even under stress (218).
The ability of root colonization is essential for a successful inoculation of a microbial-based biofertilizer (219). Usually, actinomycetes are strong rhizospheric and root colonizers (220). It is thought to relate to their isolation source background, associated with soil and plant tissue environments. However, many bacteria demonstrate good colonization competence in vitro studies and controlled conditions but fail to replicate this behavior in field applications (221). The causes may be explained by the reduced expression of bioactive metabolites when competing with other microorganisms in the soil, which results in lower rhizosphere competence and poor colonization of root and plant tissues (222).
Rhizosphere colonization competence is also important for biopesticides that act against soil-borne pathogens, such as fungi and insects (223). For controlling leaf diseases, it may be necessary that the active ingredient of the biopesticide can colonize plant tissues (224). This can be accomplished by using endophytic actinomycetes, which produce lytic enzymes and other metabolites that improve their ability to colonize the plant (225). The chosen inoculation method is another microbial colonization impact factor (226). Usually, bacteria have little motility in the soil. For this reason, depending on the species and its activity, its application as close as possible to the rhizosphere is necessary to obtain a good result (210).
The microbial concentration in the product is directly related to proper colonization and efficacy since the number of viable cells disposed of in the crop needs to be enough to promote the nutrition and protection of the plant (227). One of the main challenges faced in developing actinomycete-based products is to achieve high culture concentrations at large-scale production, once they show a slower reproduction rate than other bacteria (228, 229). In addition, liquid cultures of actinomycetes tend to form aggregates and mycelial pellets, making homogenization difficult (230). The optimization of bioprocesses associated with the industrial production of actinobacteria spores can be achieved by submerged fermentation (SF) and solid-state fermentation (SSF). The first usually involves a more expensive operation, however allowing greater control of parameters, shorter culture time and easier industrialization. Several studies have been performed to understand and optimize cultivation variables that maximize spore production combined with the mitigation of pellet and aggregate production by this fermentation strategy (231–234). On the other hand, SSF usually involves a cheaper operation, however performing a longer growing time and labored downstream operations. Likewise, in this fermentation route, some studies have investigated the optimization of spore production by actinobacteria (235–237).
These problems are also found when it comes to on-farm production of actinobacteria, mainly regarding its cultivation through solid state fermentation (SSF) or submerged fermentation (SF), usually forming aggregates and pellets (238). These structures formed in submerged cultivation reduce the homogeneity of cellular structures dispersion, can cause clogging of spray nozzles and overcoming these obstacles can implicate in bioreactors and downstream steps that can make the operation economically unfeasible under on-farm production conditions.
Formulation may be another obstacle in developing bioproducts based on actinomycetes (239). Fresh microbial cells are not suitable for agricultural application, becoming necessary research for effective solid, liquid, or powder formulations with bacterial spores/mycelium (240). Combining the optimized production of spores, the development of formulations can be a focus of study to enable a greater number of stable commercial formulations based on actinobacteria. This development is usually linked to drying or encapsulation technologies that protect the spores from conditions of high water activity (Aw) and other abiotic factors that may compromise their viability over shelf life. In this sense, some studies have studied the stabilization strategies for actinobacteria in formulations using drying approaches combined with protectants (240–245). However, advances are still needed to achieve greater stability of formulations.
Besides the active microorganism, formulations contain other inert ingredients that help with field application and shelf-life of the product. These compounds need to interact so that the strain bioactivity is not compromised (246). Nutritional Streptomyces requirements and susceptibility to abiotic factors can become problems regarding formulation development (241, 247). For this reason, the products based on actinomycetes cells are less frequent than the ones based only on their active metabolites (36).
Actinomycetes, both rhizospheric and endophytic, possess a strong ability to produce metabolites of interest directly related to their interactions with the microbiome in the environment and the plant host. Production of phytohormones modulators, nutrient-uptake increasing compounds, lytic enzymes, antibiotics, and other active metabolites makes actinomycetes an undeniable promising tool for developing microbial biofertilizers and biopesticides. Streptomyces is the most recurrent actinomycetes found in the environment and the main group with agricultural applications, showing a considerable pattern of producing extracts rich in secondary metabolites active against phytopathogenic fungi, bacteria, insects, and plant-parasitic nematodes. Although many of their bioactive compounds have already been described, further studies are required to fully comprehend its diversity, chemical structures, and molecular modes of action.
Despite all this potential, only a few products based on actinomycetes are available on the market. This may be due to many factors, such as challenges in microbial large-scale production and formulations development, which highlights the importance of optimizing bioprocesses in the cultivation of actinomycetes to achieve maximum bacterial performance and improvement of formulation technologies. Furthermore, it is also imperative to better understand the interactions between actinomycetes, environmental microbiota, and host plants to target the best method for bacterial inoculation and increase its effectiveness. Thus, the entire biological treasure of actinomycetes can be used in favor of developing products that make agriculture more sustainable and productive, having better plant nutrition and protection through the application of microbial inoculants and biopesticides.
GS, IK, IR, and PL designed the project, wrote the manuscript, and edited the manuscript. All authors contributed to the article and approved the submitted version.
This work was supported by grants from the São Paulo Research Foundation, FAPESP (Proc. Nos. 2015/10974-8 and 2020/11315-6); National Council for Scientific and Technological Development, CNPq; and Agrivalle Brasil Industria e Comércio de Produtos Agrícolas Ltda.
The authors declare that the research was conducted in the absence of any commercial or financial relationships that could be construed as a potential conflict of interest.
All claims expressed in this article are solely those of the authors and do not necessarily represent those of their affiliated organizations, or those of the publisher, the editors and the reviewers. Any product that may be evaluated in this article, or claim that may be made by its manufacturer, is not guaranteed or endorsed by the publisher.
We wish to acknowledge F. P. N. Cruz, from Department of Morphology and Pathology, Federal University of São Carlos, São Carlos, Brazil, for kindly providing the photos of the Figure 1.
1. Plumecocq G, Debril T, Duru M, Magrini MB, Sarthou JP, Therond O. The plurality of values in sustainable agriculture models. Ecol Soc. (2018) 23:121. doi: 10.5751/ES-09881-230121
2. Bindraban PS, Dimkpa CO, Angle S, Rabbinge R. Unlocking the multiple public good services from balanced fertilizers. Food Secur. (2018) 10:273–85. doi: 10.1007/s12571-018-0769-4
3. Zhang H, Li Y, Zhu JK. Developing naturally stress-resistant crops for a sustainable agriculture. Nat Plants. (2018) 4:989–96. doi: 10.1038/s41477-018-0309-4
4. Bonner MR, Alavanja MC. Pesticides, human health, and food security. Food Energy Security. (2017) 6:89–93. doi: 10.1002/fes3.112
5. Khan MN, Mohammad F. Eutrophication: challenges and solutions. In: Ansari AA, Gill SS, editors. Eutrophication: Causes, Consequences and Control. Dordrecht: Springer (2014). p. 1–15. doi: 10.1007/978-94-007-7814-6_1
6. Melović B, Cirović D, Backovic-Vulić T, Dudić B, Gubiniova K. Attracting green consumers as a basis for creating sustainable marketing strategy on the organic market—relevance for sustainable agriculture business development. Foods. (2020) 9:1552. doi: 10.3390/foods9111552
7. Umesha S, Singh PK, Singh RP. Microbial biotechnology and sustainable agriculture. In: Singh RL, Mondal S, editors. Biotechnology for Sustainable Agriculture. Sawston: Woodhead Publishing (2018). p. 185–205. doi: 10.1016/B978-0-12-812160-3.00006-4
8. Thomas L, Singh I. Microbial biofertilizers: types and applications. In: Giri B, Prasad R, Wu Q, Varma A, editors. Biofertilizers for Sustainable Agriculture and Environment. Cham: Springer (2019). p. 1–19. doi: 10.1007/978-3-030-18933-4_1
9. Tikhonovich IA, Provorov NA. Microbiology is the basis of sustainable agriculture: an opinion. Ann Appl Biol. (2011) 159:155–68. doi: 10.1111/j.1744-7348.2011.00489.x
10. Thakur N, Kaur S, Tomar P, Thakur S, Yadav AN. Microbial biopesticides: current status and advancement for sustainable agriculture and environment. In: Rastegari AA, Yadav AN, Yadav N, editors. New and Future Developments in Microbial Biotechnology and Bioengineering. Amsterdam: Elsevier (2020). p. 243–82. doi: 10.1016/B978-0-12-820526-6.00016-6
11. O'Brien PA. Biological control of plant diseases. Aust Plant Pathol. (2017) 46:293–304. doi: 10.1007/s13313-017-0481-4
12. Abbey L, Abbey J, Leke-Aladekoba A, Iheshiulo EMA, Ijenyo M. Biopesticides and biofertilizers: types, production, benefits, and utilization. In: BK Simpson, editor. Byproducts from Agriculture and Fisheries: Adding Value for Food, Feed, Pharma, and Fuels. Hoboken, NJ: John Wiley & Sons (2019). p. 479–500. doi: 10.1002/9781119383956.ch20
13. Matsumoto A, Takahashi Y. Endophytic actinomycetes: promising source of novel bioactive compounds. J Antib. (2017) 70:514–9. doi: 10.1038/ja.2017.20
14. Jakubiec-Krzesniak K, Rajnisz-Mateusiak A, Guspiel A, Ziemska J, Solecka J. Secondary metabolites of actinomycetes and their antibacterial, antifungal and antiviral properties. Pol J Microbiol. (2018) 67:259. doi: 10.21307/pjm-2018-048
15. Javed Z, Tripathi GD, Mishra M, Dashora K. Actinomycetes–the microbial machinery for the organic-cycling, plant growth, and sustainable soil health. Biocatal Agric Biotechnol. (2021) 31:101893. doi: 10.1016/j.bcab.2020.101893
16. Nagendran S, Agrawal SS, Patwardhan AG. Eco-friendly association of plants and actinomycetes. In: Shrivastava N, Mahajan S, Varma A, editors. Symbiotic Soil Microorganisms. Cham: Springer (2021). p. 99–116. doi: 10.1007/978-3-030-51916-2_6
17. Santos MLD, Berlitz DL, Wiest SLF, Schünemann R, Knaak N, Fiuza LM. Benefits associated with the interaction of endophytic bacteria and plants. Braz Arch Biol Technol. (2018) 61. doi: 10.1590/1678-4324-2018160431
18. Singh R, Dubey AK. Diversity and applications of endophytic actinobacteria of plants in special and other ecological niches. Front Microbiol. (2018) 9:1767. doi: 10.3389/fmicb.2018.01767
19. Ludwig W, Euzéby J, Schumann P, Busse HJ, Trujillo ME, Kämpfer P, et al. Road map of the phylum Actinobacteria. In: Goodfellow M, Kámpfer P, Busse H, Trujillo ME, Suzuki K, Ludwig W, Whitman WB, editors. Bergey's Manual® of Systematic Bacteriology. Springer (2012). p. 1–28. doi: 10.1007/978-0-387-68233-4_1
20. van Bergeijk DA, Terlouw BR, Medema MH, van Wezel GP. Ecology and genomics of Actinobacteria: new concepts for natural product discovery. Nat Rev Microbiol. (2020) 18:546–58. doi: 10.1038/s41579-020-0379-y
21. Cruz FPN, de Paula AF, Nogueira CT, Andrade PHM, Borges LM, Lacava PT, et al. Discovery of a novel lineage Burkholderia cepacia ST 1870 endophytically isolated from medicinal Polygala paniculata which shows potent in vitro antileishmanial and antimicrobial effects. Int J Microbiol. (2021) 6618559:17. doi: 10.1155/2021/6618559
22. Cimmino T, Metidji S, Labas N, Le Page S, Musso D, Raoult D, et al. Genome sequence and description of Actinomyces polynesiensis str. MS2 sp nov isolated from the human gut. New Microbes New Infections. (2016) 12:1–5. doi: 10.1016/j.nmni.2016.02.014
23. Wu Y, Kang Q, Zhang LL, Bai L. Subtilisin-Involved morphology engineering for improved antibiotic production in actinomycetes. Biomol. (2020) 10:851. doi: 10.3390/biom10060851
24. Barka EA, Vatsa P, Sanchez L, Gaveau-Vaillant N, Jacquard C, Klenk HP, et al. Taxonomy, physiology, and natural products of Actinobacteria. Microbiol Mol Biol Rev. (2016) 80:1–43. doi: 10.1128/MMBR.00019-15
25. Waksman SA, Schatz A, Reynolds DM. Production of antibiotic substances by actinomycetes. Ann N Y Acad Sci. (2010) 1213:112–24. doi: 10.1111/j.1749-6632.2010.05861.x
26. Hutchings MI, Truman AW, Wilkinson B. Antibiotics: past, present and future. Curr Opinion Microbiol. (2019) 51:72–80. doi: 10.1016/j.mib.2019.10.008
27. Putri DKT, Amirda F, Muzadi H, Carabelly AN, Erlita I. The antibacterial activity of actinomycetes against the growth of Streptococcus mutans and Lactobacillus acidophilus. In: BIO Web of Conferences, 20, 03006. EDP Sciences. Banjarbaru (2020). doi: 10.1051/bioconf/20202003006
28. Yeng CM, Pahirulzaman KAK. Potential application of actinomycetes as natural fungicide. In: IOP Conference Series: Earth and Environmental Science. Bristol: IOP Publishing. (2021). p. 756. doi: 10.1088/1755-1315/756/1/012066
29. Berezin V, Abdukhakimova D, Trenozhnikova L, Bogoyavlenskiy A, Turmagambetova A, Issanov A, et al. Antiviral activities of extremophilic actinomycetes extracts from Kazakhstan's unique ecosystems against influenza viruses and paramyxoviruses. Virol J. (2019) 16:1–16. doi: 10.1186/s12985-019-1254-1
30. Sholkamy EN, Muthukrishnan P, Abdel-Raouf N, Nandhini X, Ibraheem IB, Mostafa AA. Antimicrobial and antinematicidal metabolites from Streptomyces cuspidosporus strain SA4 against selected pathogenic bacteria, fungi and nematode. Saudi J Biol Sci. (2020) 27:3208–20. doi: 10.1016/j.sjbs.2020.08.043
31. Chen Y, Shafi J, Li M, Fu D, Ji M. Insecticidal activity of endophytic actinomycetes isolated from Azadirachta indica against Myzus persicae. Archives of Biol Sci. (2018) 70:349–57. doi: 10.2298/ABS170729052C
32. Tangerina MM, Furtado LC, Leite VM, Bauermeister A, Velasco-Alzate K, Jimenez PC, et al. Metabolomic study of marine Streptomyces sp.: secondary metabolites and the production of potential anticancer compounds. PLoS ONE. (2020) 15:e0244385. doi: 10.1371/journal.pone.0244385
33. Shi L, Wu Z, Zhang Y, Zhang Z, Fang W, Wang Y, et al. Herbicidal secondary metabolites from actinomycetes: structure diversity, modes of action, and their roles in the development of herbicides. J Agricul Food Chem. (2019) 68:17–32. doi: 10.1021/acs.jafc.9b06126
34. Salwan R, Sharma V. The role of actinobacteria in the production of industrial enzymes. In: Singh BP, Gupta VK, Pasari AK, editors. New and Future Developments in Microbial Biotechnology and Bioengineering. Amsterdam: Elsevier (2018). p. 165–77. doi: 10.1016/B978-0-444-63994-3.00011-4
35. Singh DP, Patil HJ, Prabha R, Yandigeri MS, Prasad SR. Actinomycetes as potential plant growth-promoting microbial communities. In: Prasad R, Gill SS, Tuteja N, editors. Crop Improvement Through Microbial Biotechnology. Amsterdam: Elsevier (2018) p. 27–38. doi: 10.1016/B978-0-444-63987-5.00002-5
36. Pacios-Michelena S, González CNA, Alvarez-Perez OB, Rodriguez-Herrera R, Chávez-González M, Valdés RA, et al. (2021). Application of Streptomyces antimicrobial compounds for the control of phytopathogens. Front Sustain Food Syst. 5:696518. doi: 10.3389/fsufs.2021.696518
37. Yadav AN, Verma P, Kumar S, Kumar V, Kumar M, Sugitha TCK, et al. Actinobacteria from rhizosphere: molecular diversity, distributions, and potential biotechnological applications. In: Singh BP, Gupta VK, Pasari AK, editors. New and Future Developments in Microbial Biotechnology and Bioengineering. Amsterdam: Elsevier (2018). p. 13–41. doi: 10.1016/B978-0-444-63994-3.00002-3
38. Vieira FCS, Nahas E. Comparison of microbial numbers in soils by using various culture media and temperatures. Microbiol Res. (2005) 160:197–202. doi: 10.1016/j.micres.2005.01.004
39. Bhatti AA, Haq S, Bhat RA. Actinomycetes benefaction role in soil and plant health. Microb Pathogenesis. (2017) 111:458–67. doi: 10.1016/j.micpath.2017.09.036
40. Hassan MK, McInroy JA, Kloepper JW. The interactions of rhizodeposits with plant growth-promoting rhizobacteria in the rhizosphere: a review. Agriculture. (2019) 9:142. doi: 10.3390/agriculture9070142
41. Wahyudi AT, Priyanto JA, Afrista R, Kurniati D, Astuti RI, Akhdiya A. Plant growth promoting activity of actinomycetes isolated from soybean rhizosphere. Online J Biol Sci. (2019) 19:1–8. doi: 10.3844/ojbsci.2019.1.8
42. El-Tarabily KA. Promotion of tomato (Lycopersicon esculentum Mill.) plant growth by rhizosphere competent 1-aminocyclopropane-1-carboxylic acid deaminase-producing streptomycete actinomycetes. Plant Soil. (2008) 308:161–74. doi: 10.1007/s11104-008-9616-2
43. Yu Z, Han C, Yu B, Zhao J, Yan Y, Huang S, et al. Taxonomic characterization, and secondary metabolite analysis of Streptomyces triticiradicis sp. nov: a novel actinomycete with antifungal activity. Microorganisms. (2020) 8:77. doi: 10.3390/microorganisms8010077
44. AbdElgawad H, Abuelsoud W, Madany MM, Selim S, Zinta G, Mousa AS, et al. Actinomycetes enrich soil rhizosphere and improve seed quality as well as productivity of legumes by boosting nitrogen availability and metabolism. Biomolecules. (2020) 10:1675. doi: 10.3390/biom10121675
45. Yadav N, Yadav AN. Actinobacteria for sustainable agriculture. J Appl Biotech Bioeng. (2019) 6:38–41. doi: 10.15406/jabb.2019.06.00172
46. Franco-Correa M, Chavarro-Anzola V. Actinobacteria as plant growth promoting rhizobacteria. In: Dhanasekaran D, Jiang Y, editors. Actinobacteria-Basis and Biotechnological Application. London: IntechOpen (2016). p. 249–70. doi: 10.5772/61291
47. Reinhold-Hurek B, Hurek T. Living inside plants: bacterial endophytes. Curr Opinion Plant Biol. (2011) 14:435–43. doi: 10.1016/j.pbi.2011.04.004
48. Shimizu M. Endophytic actinomycetes: biocontrol agents and growth promoters. In: Maheshwari DK, editor. Bacteria in Agrobiology: Plant Growth Responses. Amsterdam: Springer (2011). p. 201−20. doi: 10.1007/978-3-642-20332-9_10
49. Nalini MS, Prakash HS. Diversity and bioprospecting of actinomycete endophytes from the medicinal plants. Lett Appl Microbiol. (2017) 64:261–70. doi: 10.1111/lam.12718
50. Lobo CB, Tomás MSJ, Viruel E, Ferrero MA, Lucca ME. Development of low-cost formulations of plant growth-promoting bacteria to be used as inoculants in beneficial agricultural technologies. Microbiol Res. (2019) 219:12–25. doi: 10.1016/j.micres.2018.10.012
51. Souza RD, Ambrosini A, Passaglia LM. Plant growth-promoting bacteria as inoculants in agricultural soils. Gen Mol Biol. (2015) 38:401–19. doi: 10.1590/S1415-475738420150053
52. Harikrishnan H, Shanmugaiah V, Balasubramanian N. Optimization for production of Indole acetic acid (IAA) by plant growth promoting Streptomyces sp VSMGT1014 isolated from rice rhizosphere. Int J Curr Microbiol Appl Sci. (2014) 3:158–71.
53. Gopalakrishnan S, Vadlamudi S, Bandikinda P, Sathya A, Vijayabharathi R, Rupela O, et al. Evaluation of Streptomyces strains isolated from herbal vermicompost for their plant growth-promotion traits in rice. Microbiol Res. (2014) 169:40–8. doi: 10.1016/j.micres.2013.09.008
54. Verma VC, Singh SK, Prakash S. Bio-control and plant growth promotion potential of siderophore producing endophytic Streptomyces from Azadirachta indica A. Juss J Basic Microbiol. (2011) 51:550–6. doi: 10.1002/jobm.201000155
55. Khamna S, Yokota A, Lumyong S. Actinomycetes isolated from medicinal plant rhizosphere soils: diversity and screening of antifungal compounds, indole-3-acetic acid and siderophore production. World J Microbiol Biotechnol. (2009) 25:649–55. doi: 10.1007/s11274-008-9933-x
56. Lin L, Xu X. Indole-3-acetic acid production by endophytic Streptomyces sp. En-1 isolated from medicinal plants. Curr Microbiol. (2013) 67:209–17. doi: 10.1007/s00284-013-0348-z
57. Alori ET, Babalola OO. Microbial inoculants for improving crop quality and human health in Africa. Front Microbiol. (2018) 9:2213. doi: 10.3389/fmicb.2018.02213
58. Lakshmikandan M, Wang S, Murugesan AG, Saravanakumar M, Selvakumar G. Co-cultivation of Streptomyces and microalgal cells as an efficient system for biodiesel production and bioflocculation formation. Bioresour Technol. (2021) 332:125118. doi: 10.1016/j.biortech.2021.125118
59. Legault GS, Lerat S, Nicolas P, Beaulieu C. Tryptophan regulates thaxtomin A and indole-3-acetic acid production in Streptomyces scabiei and modifies its interactions with radish seedlings. Phytopathol. (2011) 101:1045–51. doi: 10.1094/PHYTO-03-11-0064
60. Shi L, Nwet TT, Ge B, Zhao W, Liu B, Cui H, et al. Antifungal and plant growth-promoting activities of Streptomyces roseoflavus strain NKZ-259. Biol Control. (2018) 125:57–64. doi: 10.1016/j.biocontrol.2018.06.012
61. Shutsrirung A, Chromkaew Y, Pathom-Aree W, Choonluchanon S, Boonkerd N. Diversity of endophytic actinomycetes in mandarin grown in northern Thailand, their phytohormone production potential and plant growth promoting activity. Soil Sci Plant Nutr. (2013) 59:322–30. doi: 10.1080/00380768.2013.776935
62. Nimnoi P, Pongsilp N, Lumyong S. Endophytic actinomycetes isolated from Aquilaria crassna Pierre ex Lec and screening of plant growth promoters production. World J Microbiol Biotechnol. (2010) 26:193–203. doi: 10.1007/s11274-009-0159-3
63. Nain L, Rana A, Joshi M, Jadhav SD, Kumar D, Shivay YS, et al. Evaluation of synergistic effects of bacterial and cyanobacterial strains as biofertilizers for wheat. Plant Soil. (2010) 331:217–30. doi: 10.1007/s11104-009-0247-z
64. Soe KM, Yamakawa T. Evaluation of effective Myanmar Bradyrhizobium strains isolated from Myanmar soybean and effects of coinoculation with Streptomyces griseoflavus P4 for nitrogen fixation. Soil Sci Plant Nutr. (2013) 59:361–70. doi: 10.1080/00380768.2013.794437
65. Htwe AZ, Moh SM, Soe KM, Moe K, Yamakawa T. Effects of biofertilizer produced from Bradyrhizobium and Streptomyces griseoflavus on plant growth, nodulation, nitrogen fixation, nutrient uptake, and seed yield of mung bean, cowpea, and soybean. Agronomy. (2019) 9:77. doi: 10.3390/agronomy9020077
66. Swarnalakshmi K, Yadav V, Tyagi D, Dhar DW, Kannepalli A, Kumar S. Significance of plant growth promoting rhizobacteria in grain legumes: growth promotion and crop production. Plants. (2020) 9:1596. doi: 10.3390/plants9111596
67. Solans M, Vobis G, Cassán F, Luna V, Wall LG. Production of phytohormones by root-associated saprophytic actinomycetes isolated from the actinorhizal plant Ochetophila trinervis. World J Microbiol Biotechnol. (2011) 27:2195–202. doi: 10.1007/s11274-011-0685-7
68. Aldesuquy HS, Mansour FA, Abo-Hamed SA. Effect of the culture filtrates of Streptomyces on growth and productivity of wheat plants. FM. (1998) 43:465–70. doi: 10.1007/BF02820792
69. El-Tarabily KA, Nassar AH, Hardy GSJ, Sivasithamparam K. Plant growth promotion and biological control of Pythium aphanidermatum, a pathogen of cucumber, by endophytic actinomycetes. J Appl Microbiol. (2009) 106:13–26. doi: 10.1111/j.1365-2672.2008.03926.x
70. Siddiqui ZA. PGPR: Biocontrol and Biofertilization (No. 631.8 PGP). Dordrecht: Springer (2006). doi: 10.1007/1-4020-4152-7
71. Glick BR. Modulation of plant ethylene levels by the bacterial enzyme ACC deaminase. FEMS Microbiol Lett. (2005) 251:1–7. doi: 10.1016/j.femsle.2005.07.030
72. Naing AH, Maung TT, Kim CK. The ACC deaminase-producing plant growth-promoting bacteria: influences of bacterial strains and ACC deaminase activities in plant tolerance to abiotic stress. Physiol Plant. (2021) 173:1992–2012. doi: 10.1111/ppl.13545
73. Orozco-Mosqueda M, Glick BR, Santoyo G. ACC deaminase in plant growth-promoting bacteria (PGPB): an efficient mechanism to counter salt stress in crops. Microbiol Res. (2020) 235:126439. doi: 10.1016/j.micres.2020.126439
74. Anwar S, Ali B, Sajid I. Screening of rhizospheric actinomycetes for various in-vitro and in-vivo plant growth promoting (PGP) traits and for agroactive compounds. Front Microbiol. (2016) 7:1334. doi: 10.3389/fmicb.2016.01334
75. Qin S, Zhang YJ, Yuan B, Xu PY, Xing K, Wang J, et al. Isolation of ACC deaminase-producing habitat-adapted symbiotic bacteria associated with halophyte Limonium sinense (Girard) Kuntze and evaluating their plant growth-promoting activity under salt stress. Plant Soil. (2014) 374:753–66. doi: 10.1007/s11104-013-1918-3
76. Selvakumar G, Bhatt RM, Upreti KK, Bindu GH, Shweta K. Citricoccus zhacaiensis B-4 (MTCC 12119) a novel osmotolerant plant growth promoting actinobacterium enhances onion (Allium cepa L.) seed germination under osmotic stress conditions. World J Microbiol Biotechnol. (2015) 31:833–9. doi: 10.1007/s11274-015-1837-y
77. Siddikee MA, Chauhan PS, Anandham R, Han GH, Sa TM. Isolation, characterization, and use for plant growth promotion under salt stress, of ACC deaminase-producing halotolerant bacteria derived from coastal soil. J Microbiol Biotechnol. (2010) 20:1577–84. doi: 10.4014/jmb.1007.07011
78. Hontzeas N, Richardson AO, Belimov A, Safronova V, Abu-Omar MM, Glick BR. Evidence for horizontal transfer of 1-aminocyclopropane-1-carboxylate deaminase genes. Appl Environ Microbiol. (2005) 71:7556–8. doi: 10.1128/AEM.71.11.7556-7558.2005
79. Bellenger JP, Darnajoux R, Zhang X, Kraepiel AML. Biological nitrogen fixation by alternative nitrogenases in terrestrial ecosystems: a review. Biogeochem. (2020) 149:53–73. doi: 10.1007/s10533-020-00666-7
80. Soumare A, Diedhiou AG, Thuita M, Hafidi M, Ouhdouch Y, Gopalakrishnan S, et al. Exploiting biological nitrogen fixation: a route towards a sustainable agriculture. Plants. (2020) 9:1011. doi: 10.3390/plants9081011
81. Nouioui I, Cortés-Albayay C, Carro L, Castro JF, Gtari M, Ghodhbane-Gtari F, et al. Genomic insights into plant-growth-promoting potentialities of the genus Frankia. Front Microbiol. (2019) 10:1457. doi: 10.3389/fmicb.2019.01457
83. Benson DR, Silvester WB. Biology of Frankia strains, actinomycete symbionts of actinorhizal plants. Microbiol Rev. (1993) 57:293–319. doi: 10.1128/mr.57.2.293-319.1993
84. Rascio N, Rocca L. Biological nitrogen fixation. In: Jørgensen SE, Fath BD, editors. Reference Module in Earth Systems and Enronmental Sciences: Encyclopedia of Ecology. Cham: Springer (2013). p. 412−9. doi: 10.1016/B978-008045405-4.00273-1
85. Gtari M, Ghodhbane-Gtari F, Nouioui I, Beauchemin N, Tisa LS. Phylogenetic perspectives of nitrogen-fixing actinobacteria. Arch Microbiol. (2012) 194:3–11. doi: 10.1007/s00203-011-0733-6
86. Ribbe M, Gadkari D, Meyer O. N2 fixation by Streptomyces thermoautotrophicus involves a molybdenum-Dinitrogenase and a manganese-Superoxide oxidoreductase that couple N2Reduction to the oxidation of superoxide produced from O2 by a molybdenum-CO dehydrogenase. J Biolol Chem. (1997) 272:26627–33. doi: 10.1074/jbc.272.42.26627
87. MacKellar D, Lieber L, Norman JS, Bolger A, Tobin C, Murray JW, et al. Streptomyces thermoautotrophicus does not fix nitrogen. Sci Rep. (2016) 6:1–12. doi: 10.1038/srep20086
88. Dahal B, NandaKafle G, Perkins L, Brözel VS. Diversity of free-Living nitrogen fixing Streptomyces in soils of the badlands of South Dakota. Microbiol Res. (2017) 195:31–9. doi: 10.1016/j.micres.2016.11.004
89. Turk-Kubo KA, Achilles KM, Serros TR, Ochiai M, Montoya JP, Zehr JP. Nitrogenase (nifH) gene expression in diazotrophic cyanobacteria in the Tropical North Atlantic in response to nutrient amendments. Front Microbiol. (2012) 3:386. doi: 10.3389/fmicb.2012.00386
90. Martínez-Hidalgo P, Galindo-Villardón P, Trujillo ME, Igual JM, Martínez-Molina E. Micromonospora from nitrogen fixing nodules of alfalfa (Medicago sativa L.). A new promising Plant Probiotic Bacteria. Sci Rep. (2014) 4:1–11. doi: 10.1038/srep06389
91. Saif S, Khan MS, Zaidi A, Ahmad E. Role of phosphate-solubilizing actinomycetes in plant growth promotion: current perspective. In: Khan MS, Zaidi A, Musarrat J, editors. Phosphate Solubilizing Microorganisms. Cham: Springer (2014). p. 137–56. doi: 10.1007/978-3-319-08216-5_6
92. Dastager SG, Damare S. Marine actinobacteria showing phosphate-solubilizing efficiency in Chorao Island, Goa, India. Curr Microbiol. (2013) 66:421–7. doi: 10.1007/s00284-012-0288-z
93. Novo LA, Castro PM, Alvarenga P, da Silva EF. Plant growth–promoting rhizobacteria-assisted phytoremediation of mine soils. In: Prasad MNV, Favas PJC, Maiti SK, editors. Bio-Geotechnologies for Mine Site Rehabilitation. Amsterdam: Elsevier. (2018). p. 281–95. doi: 10.1016/B978-0-12-812986-9.00016-6
94. Hamdali H, Hafidi M, Virolle MJ, Ouhdouch Y. Growth promotion and protection against damping-off of wheat by two rock phosphate solubilizing actinomycetes in a P-deficient soil under greenhouse conditions. Appl Soil Ecol. (2008) 40:510–7. doi: 10.1016/j.apsoil.2008.08.001
95. Aallam Y, Dhiba D, Lemriss S, Souiri A, Karray F, Rasafi TE, et al. Isolation and characterization of phosphate solubilizing Streptomyces sp. endemic from sugar beet fields of the beni-mellal region in Morocco. Microorganisms. (2021) 9:914. doi: 10.3390/microorganisms9050914
96. Farhat MB, Boukhris I, Chouayekh H. Mineral phosphate solubilization by Streptomyces sp. CTM396 involves the excretion of gluconic acid and is stimulated by humic acids. FEMS Microbiol Lett. (2015) 362:fnv008. doi: 10.1093/femsle/fnv008
97. Hamdali H, Bouizgarne B, Hafidi M, Lebrihi A, Virolle MJ, Ouhdouch Y. Screening for rock phosphate solubilizing Actinomycetes from Moroccan phosphate mines. Appl Soil Ecol. (2008) 38:12–9. doi: 10.1016/j.apsoil.2007.08.007
98. El-Tarabily KA, Nassar AH, Sivasithamparam K. Promotion of growth of bean (Phaseolus vulgaris L.) in a calcareous soil by a phosphate-solubilizing rhizosphere-competent isolate of Micromonospora endolithica. Appl Soil Ecol. (2008) 39:161–71. doi: 10.1016/j.apsoil.2007.12.005
99. Ferreira MJ, Silva H, Cunha A. Siderophore-producing rhizobacteria as a promising tool for empowering plants to cope with iron limitation in saline soils: a review. Pedosphere. (2019) 29:409–20. doi: 10.1016/S1002-0160(19)60810-6
100. Gu S, Yang T, Shao Z, Wang T, Cao K, Jousset A, et al. Siderophore-mediated interactions determine the disease suppressiveness of microbial consortia. Msystems. (2020) 5:e00811–9. doi: 10.1128/mSystems.00811-19
101. Lee J, Postmaster A, Soon HP, Keast D, Carson KC. Siderophore production by actinomycetes isolates from two soil sites in Western Australia. Biometals. (2012) 25:285–96. doi: 10.1007/s10534-011-9503-9
102. Wang W, Qiu Z, Tan H, Cao L. Siderophore production by actinobacteria. Biometals. (2014) 27:623–31. doi: 10.1007/s10534-014-9739-2
103. Nakouti I, Sihanonth P, Hobbs G. A new approach to isolating siderophore-producing actinobacteria. Lett Appl Microbiol. (2012) 55:68–72. doi: 10.1111/j.1472-765X.2012.03259.x
104. Schneider K, Rose I, Vikineswary S, Jones AL, Goodfellow M, Nicholson G, et al. Nocardichelins A and B, siderophores from Nocardia strain acta 3026. J Nat Prod. (2007) 70:932–5. doi: 10.1021/np060612i
105. Rungin S, Indananda C, Suttiviriya P, Kruasuwan W, Jaemsaeng R, Thamchaipenet A. Plant growth enhancing effects by a siderophore-producing endophytic streptomycete isolated from a Thai jasmine rice plant (Oryza sativa L. cv KDML105). Antonie Van Leeuwenhoek. (2012) 102:463–72. doi: 10.1007/s10482-012-9778-z
106. Miranda-CasoLuengo R, Coulson GB, Miranda-CasoLuengo A, Vázquez-Boland JA, Hondalus MK, Meijer WG. The hydroxamate siderophore rhequichelin is required for virulence of the pathogenic actinomycete Rhodococcus equi. Infect Immun. (2012) 80:4106–14. doi: 10.1128/IAI.00678-12
107. Afonin AM, Gribchenko ES, Akhtemova GA, Laktionov YV, Kozhemyakov AP, Zhukov VA. Complete genome sequence of the bacterial component of mysorin biopreparation. Microbiol Resourc Announc. (2021) 10:e01287–e01220. doi: 10.1128/MRA.01287-20
108. Mishra J, Arora NK. Bioformulations for plant growth promotion and combating phytopathogens: a sustainable approach. In: Bioformulations: For sustainable agriculture, ed. Arora NK, Mehnaz S amd Balestrini R. New Delhi: Springer. (2016). p. 3–33. doi: 10.1007/978-81-322-2779-3_1
109. Sawicka B, Egbuna C. Pests of agricultural crops and control measures. In: Natural Remedies for Pest, Disease and Weed Control. Cambridge, MA: Academic Press. (2020). p. 1–16. doi: 10.1016/B978-0-12-819304-4.00001-4
110. Alphey N, Bonsall MB. Genetics-based methods for agricultural insect pest management. Agric Forest Entomol. (2018) 20:131–40. doi: 10.1111/afe.12241
111. Mashela PW, De Waele D, Dube Z, Khosa MC, Pofu KM, Tefu G, et al. Alternative nematode management strategies. In: Fourie H, Spaull VW, Jones R, Daneel MS, Waele DD, editors. Nematology in South Africa: A View From the 21st Century. Cham: Springer (2017). p. 151–81. doi: 10.1007/978-3-319-44210-5_7
112. Penha RO, Vandenberghe LP, Faulds C, Soccol VT, Soccol CR. Bacillus lipopeptides as powerful pest control agents for a more sustainable and healthy agriculture: recent studies and innovations. Planta. (2020) 251:1–15. doi: 10.1007/s00425-020-03357-7
113. Nicolopoulou-Stamati P, Maipas S, Kotampasi C, Stamatis P, Hens L. Chemical pesticides and human health: the urgent need for a new concept in agriculture. Front Publ Health. (2016) 4:148. doi: 10.3389/fpubh.2016.00148
114. Stirling GR. Biological Control of Plant-Parasitic Nematodes. Boca Raton, FL: CRC Press (2018). p. 103–50. doi: 10.1201/9781351071468-9
115. Köhl J, Kolnaar R, Ravensberg WJ. Mode of action of microbial biological control agents against plant diseases: relevance beyond efficacy. Front Plant Sci. (2019) 10:845. doi: 10.3389/fpls.2019.00845
116. Legein M, Smets W, Vandenheuvel D, Eilers T, Muyshondt B, Prinsen E, et al. Modes of action of microbial biocontrol in the phyllosphere. Front Microbiol. (2020) 11:1619. doi: 10.3389/fmicb.2020.01619
117. Beneduzi A, Ambrosini A, Passaglia LM. Plant growth-promoting rhizobacteria (PGPR): their potential as antagonists and biocontrol agents. Genet Mol Biol. (2012) 35:1044–51. doi: 10.1590/S1415-47572012000600020
118. Jamalizadeh M, Etebarian HR, Aminian H, Alizadeh A. A review of mechanisms of action of biological control organisms against post-harvest fruit spoilage. Eppo Bulletin. (2011) 41:65–71. doi: 10.1111/j.1365-2338.2011.02438.x
119. Maramorosch K, Loebenstein G. Plant Disease Resistance: Natural, Non-Host Innate or Inducible. Amsterdam: Elsevier. (2009). doi: 10.1016/B978-012373944-5.00341-2
120. Arseneault T, Filion M. Biocontrol through antibiosis: exploring the role played by subinhibitory concentrations of antibiotics in soil and their impact on plant pathogens. Can J Plant Pathol. (2017) 39:267–74. doi: 10.1080/07060661.2017.1354335
121. Ulloa-Ogaz AL, Muñoz-Castellanos LN, Nevárez-Moorillón GV. Biocontrol of phytopathogens: antibiotic production as mechanism of control. Technol Adv Educat Progr. (2015) 2015:305–9.
122. Cuesta G, García-de-la-Fuente R, Abad M, Fornes F. Isolation and identification of actinomycetes from a compost-amended soil with potential as biocontrol agents. J Environ Manag. (2012) 95:S280–4. doi: 10.1016/j.jenvman.2010.11.023
123. Sharma A, Diwevidi VD, Singh S, Pawar KK, Jerman M, Singh LB, et al. Biological control and its important in agriculture. Int J Biotech Bioeng Res. (2013) 4:175–80.
124. de Oliveira TB, de Lucas RC, Scarcella ASDA, Pasin TM, Contato AG, Polizeli MD. Cold-active lytic enzymes and their applicability in the biocontrol of postharvest fungal pathogens. J Agric Food Chem. (2020) 68:6461–3. doi: 10.1021/acs.jafc.0c03085
125. David BV, Chandrasehar G, Selvam PN. Pseudomonas fluorescens: a plant-growth-promoting rhizobacterium (PGPR) with potential role in biocontrol of pests of crops. In: R Prasad, editor, Crop Improvement Through Microbial Biotechnology. Amsterdam: Elsevier (2018). p. 221–43. doi: 10.1016/B978-0-444-63987-5.00010-4
126. Olanrewaju OS, Babalola OO. Streptomyces: implications and interactions in plant growth promotion. Appl Microbiol Biotechnol. (2019) 103:1179–88. doi: 10.1007/s00253-018-09577-y
127. Suleman P, Al-Musallam A, Menezes CA. The effect of biofungicide Mycostop on Ceratocystis radicicola, the causal agent of black scorch on date palm. BioControl. (2002) 47:207–16. doi: 10.1023/A:1014519726573
128. Lichatowich T. The plant growth enhancing and biocontrol mechanisms of Streptomyces lydicus WYEC 108 and its use in nursery and greenhouse production. In: Proceedings RMRS-P-50. Fort Collins, CO: US Department of Agriculture, Forest Service, Rocky Mountain Research Station (2007). p. 61–2.
129. European Food Safety Authority. Conclusion on the peer review of the pesticide risk assessment of the active substance Streptomyces lydicus. WYEC. (2013) 11:3425. doi: 10.2903/j.efsa.2013.3425
130. Berg G, Marten P, Minkwitz A, Bruckner S, Luth P. Efficient biological control of fungal plant diseases by Streptomyces rimosus DSMZ 12424. IOBC WPRS Bullet. (2001) 24:9–14.
131. Amiri-Besheli B. Toxicity evaluation of Tracer, Palizin, Sirinol, Runner and Tondexir with and without mineral oils on Phylocnistis citrella Stainton. Afr J Biotechnol. (2009) 8:3382–6. doi: 10.5897/AJB09.616
132. Kuhar TP, Speese J. A Powerful New Insecticide for the Organic Grower. Blacksburg: VirginaTech (2009).
133. Reddy PP. Recent Advances in Crop Protection. New Delhi: Springer (2012). doi: 10.1007/978-81-322-0723-8
134. Burns JA. Actinovate STP Fungicide. Washington, DC: United States Environmental Protection Agency (2017). p. 20460.
135. Sharma M, Jasrotia S, Ohri P, Manhas RK. Nematicidal potential of Streptomyces antibioticus strain M7 against Meloidogyne incognita. AMB Express. (2019) 9:1–8. doi: 10.1186/s13568-019-0894-2
136. d'Errico G, Vinale RMF, Landi S, Roversi PF, Woo SL. Nematicidal efficacy of new abamectin-based products used alone and in combination with indolebutyric acid against the root-knot nematode Meloidogyne incognita. Redia. (2017) 100:95–101. doi: 10.19263/REDIA-100.17.12
137. Almeida F, Rodrigues ML, Coelho C. The still underestimated problem of fungal diseases worldwide. Front Microbiol. (2019) 10:214. doi: 10.3389/fmicb.2019.00214
138. Fisher MC, Gurr SJ, Cuomo CA, Blehert DS, Jin H, Stukenbrock EH, et al. Threats posed by the fungal kingdom to humans, wildlife, and agriculture. MBio. (2020) 11:e00449–20. doi: 10.1128/mBio.00449-20
139. Price CL, Parker JE, Warrilow AG, Kelly DE, Kelly SL. Azole fungicides–understanding resistance mechanisms in agricultural fungal pathogens. Pest Manag Sci. (2015) 71:1054–8. doi: 10.1002/ps.4029
140. Shimizu M, Nakagawa Y, Yukio SATO, Furumai T, Igarashi Y, Onaka H, et al. Studies on endophytic actinomycetes (I) Streptomyces sp. isolated from rhododendron and its antifungal activity. J Gen Plant Pathol. (2000) 66:360–6. doi: 10.1007/PL00012978
141. Taechowisan T, Peberdy JF, Lumyong S. Isolation of endophytic actinomycetes from selected plants and their antifungal activity. World J Microbiol Biotechnol. (2003) 19:381–5. doi: 10.1023/A:1023901107182
142. Lian Q, Zhang J, Gan L, Ma Q, Zong Z, Wang Y. The biocontrol efficacy of Streptomyces pratensis LMM15 on Botrytis cinerea in tomato. BioMed Res Int. (2017) 2017:11. doi: 10.1155/2017/9486794
143. Palaniyandi SA, Yang SH, Cheng JH, Meng L, Suh JW. Biological control of anthracnose (Colletotrichum gloeosporioides) in yam by Streptomyces sp. MJM5763. J Appl Microbiol. (2011) 111:443–55. doi: 10.1111/j.1365-2672.2011.05048.x
144. Kaur T, Rani R, Manhas RK. Biocontrol and plant growth promoting potential of phylogenetically new Streptomyces sp. MR14 of rhizospheric origin. AMB Express. (2019) 9:1–14. doi: 10.1186/s13568-019-0849-7
145. Khair A. In vitro antifungal activity of Streptomyces spororaveus RDS28 against some phytopathogenic fungi Afr. J Agricul Res. (2011) 6:2835–42.
146. Baniasadi F, Bonjar GS, Baghizadeh A, Nik AK, Jorjandi M, Aghighi S, et al. Biological control of Sclerotinia sclerotiorum, causal agent of sunflower head and stem rot disease, by use of soil borne actinomycetes isolates. AJABS. (2009) 4:146–51. doi: 10.3844/ajabssp.2009.146.151
147. Shrivastava P, Kumar R, Yandigeri MS. In vitro biocontrol activity of halotolerant Streptomyces aureofaciens K20: A potent antagonist against Macrophomina phaseolina (Tassi) Goid. Saudi Saudi J Biol Sci. (2017) 24:192–9. doi: 10.1016/j.sjbs.2015.12.004
148. Devi SS, Rao KB. Exploration of antimicrobial compounds from Streptomyces S9 against a phytopathogen, Corynespora cassiicola (Berk & Curtis). J Biopestic. (2017) 10:1–9.
149. Loliam B, Morinaga T, Chaiyanan S. Biocontrol of Pythium aphanidermatum by the cellulolytic actinomycetes Streptomyces rubrolavendulae S4. Sci Asia. (2013) 39:584–90. doi: 10.2306/scienceasia1513-1874.2013.39.584
150. Maldonado MC, Orosco CE, Gordillo MA, Navarro AR. In vivo and in vitro antagonism of Streptomyces sp. RO3 against Penicillium digitatum and Geotrichum candidum. Afr J Microbiol Res 4. (2010) 2451–6. doi: 10.5897/AJMR.9000406
151. Qi D, Zou L, Zhou D, Chen Y, Gao Z, Feng R, et al. Taxonomy and broad-spectrum antifungal activity of Streptomyces sp. SCA3-4 isolated from rhizosphere soil of opuntia stricta. Front Microbiol 10. (2019) 2019:1390. doi: 10.3389/fmicb.2019.01390
152. Sharma M, Manhas RK. Purification and characterization of salvianolic acid B from Streptomyces sp. M4 possessing antifungal activity against fungal phytopathogens. Microbiol Res 237. (2020) 126478. doi: 10.1016/j.micres.2020.126478
153. Shahid M, Singh BN, Verma S, Choudhary P, Das S, Chakdar H, et al. Bioactive antifungal metabolites produced by Streptomyces amritsarensis V31 help to control diverse phytopathogenic fungi. Braz J Microbiol. (2021) 1–13. doi: 10.1007/s42770-021-00625-w
154. Nayak SK, Dash B, Nayak S, Mohanty S, Mishra BB. Chitinase producing soil bacteria: prospects and applications. In: Frontiers in Soil and Environmental Microbiology. Boca Raton, FL: CRC Press. (2020). p. 289–98. doi: 10.1201/9780429485794-30
155. Yano S, Kanno H, Tsuhako H, Ogasawara S, Suyotha W, Konno H, et al. Cloning, expression, and characterization of a GH 19-type chitinase with antifungal activity from Lysobacter sp. MK9-1. J Biosci Bioeng. (2021) 131:348–55. doi: 10.1016/j.jbiosc.2020.11.005
156. Malviya MK, Trivedi P, Pandey A. Chitinase and glucanase activities of antagonistic Streptomyces spp isolated from fired plots under shifting cultivation in northeast India. J Adv Res Biotech. (2018) 3:1–7. doi: 10.15226/2475-4714/3/1/00130
157. Saini A, Aggarwal NK, Sharma A, Yadav A. Actinomycetes: a source of lignocellulolytic enzymes. Enzyme Res. (2015) 2015:15. doi: 10.1155/2015/279381
158. Solanki P, Putatunda C, Kumar A, Bhatia R, Walia A. Microbial proteases: ubiquitous enzymes with innumerable uses. Biotech. (2021) 11:1–25. doi: 10.1007/s13205-021-02928-z
159. Inderiati S, Franco CM. Isolation and identification of endophytic actinomycetes and their antifungal activity. J Biotechnol Res Trop Reg. (2008) 1:1–6.
160. Gangwar M, Rani S, Sharma N. Investigating endophytic actinomycetes diversity from rice for plant growth promoting and antifungal activity. IJALS. (2012) 1:10–21.
161. Kavitha A, Vijayalakshmi M, Sudhakar P, Narasimha G. Screening of actinomycetes strains for the production of antifungal metabolites. Afr J Microbiol Res. (2010) 4:027–32. doi: 10.5897/AJMR.9000642
162. Lu Y, Wang N, He J, Li Y, Gao X, Huang L, et al. Expression and characterization of a novel chitinase with antifungal activity from a rare actinomycete, Saccharothrix yanglingensis Hhs.015. Protein Expression Purification. (2018) 143:45–51. doi: 10.1016/j.pep.2017.10.013
163. Tsujibo H, Kubota T, Yamamoto M, Miyamoto K, Inamori Y. Characterization of chitinase genes from an alkaliphilic actinomycete, Nocardiopsis prasina OPC-131. Appl Environ Microbiol. (2003) 69:894–900. doi: 10.1128/AEM.69.2.894-900.2003
164. Reyes-Tena A, Rincón-Enríquez G, López-Pérez L, Quiñones-Aguilar EE. Effect of mycorrhizae and actinomycetes on growth and bioprotection of Capsicum annuum L. against Phytophthora capsici. Pakist J Agricul Sci. (2017) 54:4245. doi: 10.21162/PAKJAS/17.4245
165. Sastrahidayat IR, Djauhari S, Prasetya B, Saleh N. Biocontrol of damping-off disease (Sclerotium rolfsii Sacc.) using Actinomycetes and VAM fungi on soybean and impact to crop production and microorganism diversity in rhizosfer zone. Int J Academ Res. (2011) 3:114–9. doi: 10.17503/agrivita.v33i1.37
166. Marimuthu S, Karthic C, Mostafa AA, Al-Enazi NM, Abdel-Raouf N, Sholkamy EN. Antifungal activity of Streptomyces sp. SLR03 against tea fungal plant pathogen Pestalotiopsis theae. J King Saud Univ Sci. (2020) 32:3258–64. doi: 10.1016/j.jksus.2020.08.027
167. Boukaew S, Yossan S, Cheirsilp B, Petlamul W, Prasertsan P. Influences of culture media, temperature and light/dark conditions on growth and antifungal activity of Streptomyces spp. Against Botrytis cinerea, in vitro and on Tomato Leaf. Res Square. (2021) 1:1–20. doi: 10.21203/rs.3.rs-385032/v1
168. Sangkanu S, Rukachaisirikul V, Suriyachadkun C, Phongpaichit S. Antifungal activity of marine-derived actinomycetes against Talaromyces marneffei. J Appl Microbiol. (2021) 130:1508–22. doi: 10.1111/jam.14877
169. Besset-Manzoni Y, Joly P, Brutel A, Gerin F, Soudière O, Langin T, et al. Does in vitro selection of biocontrol agents guarantee success in planta? A study case of wheat protection against Fusarium seedling blight by soil bacteria. PLoS ONE. (2019) 14:e0225655. doi: 10.1371/journal.pone.0225655
170. Comby M, Gacoin M, Robineau M, Rabenoelina F, Ptas S, Dupont J, et al. Screening of wheat endophytes as biological control agents against Fusarium head blight using two different in vitro tests. Microbiol Res. (2017) 202:11–20. doi: 10.1016/j.micres.2017.04.014
171. Thambugala KM, Daranagama DA, Phillips AJ, Kannangara SD, Promputtha I. Fungi vs. fungi in biocontrol: an overview of fungal antagonists applied against fungal plant pathogens. Front Cell Infec Microbiol. (2020) 10:604923. doi: 10.3389/fcimb.2020.604923
172. Rai M, Ingle A. Role of nanotechnology in agriculture with special reference to management of insect pests. Appl Microbiol Biotechnol. (2012) 94:287–93. doi: 10.1007/s00253-012-3969-4
173. Sharma S, Kooner R, Arora R. Insect pests and crop losses. In: Arora R, Sandhu S, editors. Breeding Insect Resistant Crops for Sustainable Agriculture. Singapore: Springer (2017). p. 45–66. doi: 10.1007/978-981-10-6056-4_2
174. Campos EV, Proença PL, Oliveira JL, Bakshi M, Abhilash PC, Fraceto LF. Use of botanical insecticides for sustainable agriculture: future perspectives. Ecol Indic. (2019) 105:483–95. doi: 10.1016/j.ecolind.2018.04.038
175. Li S, Yang B, Tan GY, Ouyang LM, Qiu S, Wang W, et al. Polyketide pesticides from actinomycetes. Curr Opinion Biotechnol. (2021) 69:299–307. doi: 10.1016/j.copbio.2021.05.006
176. Hariprasad KV. Recent advancement in the development of biopesticides by Actinomycetes for the control of insect pests. In: Subramaniam G, Arumugam S, Rajendran V, editors. Plant Growth Promoting Actinobacteria. Singapore: Springer (2016). p. 47–62. doi: 10.1007/978-981-10-0707-1_4
177. He H, Ye L, Li C, Wang H, Guo X, Wang X, et al. SbbR/SbbA, an important ArpA/AfsA-like system, regulates milbemycin production in Streptomyces bingchenggensis. Front Microbiol. (2018) 9:1064. doi: 10.3389/fmicb.2018.01064
178. Zhang J, Nan X, Yu HT, Cheng PL, Zhang Y, Liu YQ, et al. Synthesis, biological activities and structure– activity relationships for new avermectin analogues. Eur J Med Chem. (2016) 121:422–32. doi: 10.1016/j.ejmech.2016.05.056
179. Kim HJ, White-Phillip JA, Ogasawara Y, Shin N, Isiorho EA, Liu HW. Biosynthesis of spinosyn in Saccharopolyspora spinosa: synthesis of permethylated rhamnose and characterization of the functions of SpnH, SpnI, and SpnK. J Am Chem Soc. (2010) 132:2901–3. doi: 10.1021/ja910223x
180. Salgado VL. Studies on the mode of action of spinosad: insect symptoms and physiological correlates. Pest Biochem Physiol. (1998) 60:91–102. doi: 10.1006/pest.1998.2332
181. El-Khawaga MA, Megahed MMM. Antibacterial and insecticidal activity of actinomycetes isolated from sandy soil of (Cairo-Egypt). Egypt Acad J Biol Sci. (2012) 4:53–67. doi: 10.21608/eajbsg.2012.16661
182. Kim JH, Choi JY, Park DH, Park DJ, Park MG, Kim SY, et al. Isolation and characterization of the insect growth regulatory substances from actinomycetes. Comparat. Biochem. Physiol. C Toxicol. Pharmacol. (2020) 228:108651. doi: 10.1016/j.cbpc.2019.108651
183. Liu H, Qin S, Wang Y, Li W, Zhang J. Insecticidal action of Quinomycin A from Streptomyces sp. KN-0647 isolated from a forest soil. World J Microbiol Biotechnol. (2008) 24:2243–8. doi: 10.1007/s11274-008-9736-0
184. Hozzein W. Insecticidal activities of newly isolated actinomycetes against the rift valley fever mosquito Aedes aegyptii. In: Proceedings of International Academic Conferences (No. 5107207). London: International Institute of Social and Economic Sciences (2017). doi: 10.20472/IAC.2017.029.013
185. Gadelhak GG, El-Tarabily KA, Al-Kaabi FK. Insect control using chitinolytic soil actinomycetes as biocontrol agents. Int J Agri Biol. (2005) 7:627–33.
186. Singh S, Singh B, Singh AP. Nematodes: a threat to sustainability of agriculture. Procedia Environ Sci. (2015) 29:215–6. doi: 10.1016/j.proenv.2015.07.270
187. Palomares-Rius JE, Hasegawa K, Siddique S, Vicente CS. Protecting our crops-approaches for plant parasitic nematode control. Front Plant Sci. (2021) 2021:1475. doi: 10.3389/fpls.2021.726057
188. Mesa-Valle CM, Garrido-Cardenas JA, Cebrian-Carmona J, Talavera M, Manzano-Agugliaro F. Global research on plant nematodes. Agronomy. (2020) 10:1148. doi: 10.3390/agronomy10081148
189. Bernard GC, Egnin M, Bonsi C. The impact of plant-parasitic nematodes on agriculture and methods of control. In: Shah MM, Mahamood M, editors. Nematology-Concepts, Diagnosis and Control. London: IntechOpen (2017). p. 10. doi: 10.5772/intechopen.68958
190. Liu MJ, Hwang BS, Jin CZ, Li WJ, Park DJ, Seo ST, et al. Screening, isolation and evaluation of a nematicidal compound from actinomycetes against the pine wood nematode, Bursaphelenchus xylophilus. Pest Manag Sci. (2019) 75:1585–93. doi: 10.1002/ps.5272
191. Zeng Q, Huang H, Zhu J, Fang Z, Sun Q, Bao S. A new nematicidal compound produced by Streptomyces albogriseolus HA10002. Antonie Van Leeuwenhoek. (2013) 103:1107–11. doi: 10.1007/s10482-013-9890-8
192. Ruanpanun P, Laatsch H, Tangchitsomkid N, Lumyong S. Nematicidal activity of fervenulin isolated from a nematicidal actinomycete, Streptomyces sp. CMU-MH021, on Meloidogyne incognita. World J Microbiol Biotechnol. (2011) 27:1373–80. doi: 10.1007/s11274-010-0588-z
193. Rashad FM, Fathy HM, El-Zayat AS, Elghonaimy AM. Isolation and characterization of multifunctional Streptomyces species with antimicrobial, nematicidal and phytohormone activities from marine environments in Egypt. Microbiol Res. (2015) 175:34–47. doi: 10.1016/j.micres.2015.03.002
194. Cabrera JA, Menjivar RD, Dababat AEFA, Sikora RA. Properties and nematicide performance of avermectins. J Phytopathol. (2013) 161:65–9. doi: 10.1111/jph.12014
195. Huang XW, Tian BY, Niu QH, Yang JK, Zhang LM, Zhang KQ. An extracellular protease from 35 Brevibacillus laterosporus G4 without parasporal crystal can serve as a pathogenic factor in infection of nematodes. Res Microbiol. (2005) 156:719–27. doi: 10.1016/j.resmic.2005.02.006
196. Wang B, Liu XY, Wu WP, Liu XZ, Li SD. Purification, characterization, and gene cloning of an alkaline serine protease from a highly virulent strain of the nematode endoparasitic fungus Hirsutella rhossiliensis. Microbiol Res. (2009) 164:665–73. doi: 10.1016/j.micres.2009.01.003
197. Marin-Bruzos M, Grayston SJ. Biological control of nematodes by plant growth promoting rhizobacteria: secondary metabolites involved and potential applications. In: Singh HB, Keswani C, Reddy MS, Sansinenea E, García-Estrada C, editors. Secondary Metabolites of Plant Growth Promoting Rhizomicroorganisms. Singapore: Springer (2019). p. 253–64. doi: 10.1007/978-981-13-5862-3_13
198. Yoon GY, Lee YS, Lee SY, Park RD, Hyun HN, Nam Y, et al. Effects on Meloidogyne incognita of chitinase, glucanase and a secondary metabolite from Streptomyces cacaoi GY525. Nematology. (2012) 14:175–84. doi: 10.1163/138855411X584124
199. Tampakati AP, Hatziloukas E, Panopoulos NJ. Plant pathogens, bacterial. Encycloped Microbiol. (2009) 1:655–77. doi: 10.1016/B978-012373944-5.00346-1
200. Abdallah ME, Haroun SA, Gomah AA, El-Naggar NE, Badr HH. Application of actinomycetes as biocontrol agents in the management of onion bacterial rot diseases. Arch Phytopathol. (2013) 46:1797–808. doi: 10.1080/03235408.2013.778451
201. El Karkouri A, El Hassani FZ, El Mzibri M, Benlemlih M, El Hassouni M. Isolation and identification of an actinomycete strain with a biocontrol effect on the phytopathogenic Erwinia chrysanthemi 3937VIII responsible for soft rot disease. Ann Microbiol. (2010) 60:263–8. doi: 10.1007/s13213-010-0036-1
202. Promnuan Y, Promsai S, Pathom-Aree W, Meelai S. Apis andreniformis associated Actinomycetes show antimicrobial activity against black rot pathogen (Xanthomonas campestris pv. campestris). PeerJ. (2021) 9:e12097. doi: 10.7717/peerj.12097
203. Djebaili R, Pellegrini M, Bernardi M, Smati M, Kitouni M, Del Gallo M. Biocontrol activity of actinomycetes strains against fungal and bacterial pathogens of Solanum lycopersicum L. and Daucus carota L: in vitro and in planta antagonistic activity. In: Presented at the 1st International Electronic Conference on Plant Science. Basel (2020). p. 15. doi: 10.3390/IECPS2020-08863
204. Promnuan Y, Promsai S, Meelai S. Antimicrobial activity of Streptomyces spp. isolated from Apis dorsata combs against some phytopathogenic bacteria. Peer J. (2020) 8:e10512. doi: 10.7717/peerj.10512
205. Charousová I, Medo J, Hleba L, Císarová M, Javoreková S. Antimicrobial activity of actinomycetes and characterization of actinomycin-producing strain KRG-1 isolated from Karoo, South Africa. Braz J Pharmac Sci. (2019) 55:97902019000217249. doi: 10.1590/s2175-97902019000217249
206. Igarashi Y, Ogura H, Furihata K, Oku N, Indananda C, Thamchaipenet A. Maklamicin, an antibacterial polyketide from an endophytic Micromonospora sp. J Nat Prod. (2011) 74:670–4. doi: 10.1021/np100727h
207. Igarashi Y, Iida T, Oku N, Watanabe H, Furihata K, Miyanouchi K. Nomimicin, a new spirotetronate-class polyketide from an actinomycete of the genus Actinomadura. J Antibiotics. (2012) 65:355–9. doi: 10.1038/ja.2012.30
208. Rateb ME, Houssen WE, Arnold M, Abdelrahman MH, Deng H, Harrison WT, et al. Chaxamycins A–D, bioactive ansamycins from a hyper-arid desert Streptomyces sp. J Nat Prod. (2011) 74:1491–9. doi: 10.1021/np200320u
209. Encheva-Malinova M, Stoyanova M, Avramova H, Pavlova Y, Gocheva B, Ivanova I, et al. Antibacterial potential of streptomycete strains from Antarctic soils. Biotechnol Biotechnol Equip. (2014) 28:721–7. doi: 10.1080/13102818.2014.947066
210. Lopes MJDS, Dias-Filho MB, Gurgel ESC. Successful plant growth-promoting microbes: inoculation methods and abiotic factors. Front Sustain Food Syst. (2021) 5:48. doi: 10.3389/fsufs.2021.606454
211. Ahemad M, Kibret M. Mechanisms and applications of plant growth promoting rhizobacteria: current perspective. J King Saud Univ Sci. (2014) 26:1–20. doi: 10.1016/j.jksus.2013.05.001
212. Malusà E, Pinzari F, Canfora L. Efficacy of biofertilizers: challenges to improve crop production. In: Singh DP, Singh HB, Prabha R, editors. Microbial Inoculants in Sustainable Agricultural Productivity. New Delhi: Springer (2016). p. 17–40. doi: 10.1007/978-81-322-2644-4_2
213. Glare TR, Moran-Diez ME. Microbial-Based Biopesticides. New York, NY: Spring Sciences (2016). doi: 10.1007/978-1-4939-6367-6
214. Sahayaraj K. Nanotechnology and plant biopesticides: an overview. Ad Plant Biopest. (2014) 279–93. doi: 10.1007/978-81-322-2006-0_14
215. Egamberdieva D, Davranov K, Wirth S, Hashem A, Abd_Allah EF. Impact of soil salinity on the plant-growth–promoting and biological control abilities of root associated bacteria. Saudi JBiol Sci. (2017) 24:1601–8. doi: 10.1016/j.sjbs.2017.07.004
216. Burpee LL. The influence of abiotic factors on biological control of soilborne plant pathogenic fungi. Can J Plant Pathol. (1990) 12:308–17. doi: 10.1080/07060669009501005
217. Etesami H. Plant–microbe interactions in plants and stress tolerance. In: Plant Life Under Changing Environment. Cambridge, MA: Academic Press. (2020). p. 355–96. doi: 10.1016/B978-0-12-818204-8.00018-7
218. Ameur H, Ghoul M, Selvin J. The osmoprotective effect of some organic solutes on Streptomyces sp. MADO2 and Nocardiopsis sp MADO3 growth. Braz J Microbiol 42. (2011) 543–53. doi: 10.1590/S1517-83822011000200019
219. Doumbou CL, Hamby Salove MK, Crawford DL, Beaulieu C. Actinomycetes, promising tools to control plant diseases and to promote plant growth. Phytoprotection. (2001) 82:85–102. doi: 10.7202/706219ar
220. Merzaeva OV, Shirokikh IG. Colonization of plant rhizosphere by actinomycetes of different genera. Microbiol. (2006) 75:226–30. doi: 10.1134/S0026261706020184
221. Bonaldi M, Chen X, Kunova A, Pizzatti C, Saracchi M, Cortesi P. Colonization of lettuce rhizosphere and roots by tagged Streptomyces. Front Microbiol. (2015) 6:25. doi: 10.3389/fmicb.2015.00025
222. Compant S, Clément C, Sessitsch A. Plant growth-promoting bacteria in the rhizo-and endosphere of plants: their role, colonization, mechanisms involved and prospects for utilization. Soil Biol Biochem. (2010) 42:669–78. doi: 10.1016/j.soilbio.2009.11.024
223. Walker TS, Bais HP, Grotewold E, Vivanco JM. Root exudation and rhizosphere biology. Plant Physiol. (2003) 132:44–51. doi: 10.1104/pp.102.019661
224. Akbaba M, Ozaktan H. Biocontrol of angular leaf spot disease and colonization of cucumber (Cucumis sativus L.) by endophytic bacteria. Egypt J Biol Pest Control. (2018) 28:1–10. doi: 10.1186/s41938-017-0020-1
225. Liu H, Carvalhais LC, Crawford M, Singh E, Dennis PG, Pieterse CM, et al. Inner plant values: diversity, colonization and benefits from endophytic bacteria. Front Microbiol. (2017) 8:2552. doi: 10.3389/fmicb.2017.02552
226. Afzal M, Khan S, Iqbal S, Mirza MS, Khan QM. Inoculation method affects colonization and activity of Burkholderia phytofirmans PsJN during phytoremediation of diesel-contaminated soil. Int Biodeterior. (2013) 85:331–6. doi: 10.1016/j.ibiod.2013.08.022
227. Mitter EK, Tosi M, Obregón D, Dunfield KE, Germida JJ. Rethinking crop nutrition in times of modern microbiology: innovative biofertilizer technologies. Front Sustain Food Syst. (2021) 5:29. doi: 10.3389/fsufs.2021.606815
228. Terkina IA, Parfenova VV, Ahn TS. Antagonistic activity of actinomycetes of Lake Baikal. Appl Biochem Microbiol. (2006) 42:173–6. doi: 10.1134/S0003683806020104
229. Králová S., Sandoval-Powers M, Fawwal DV, Degnes KF, Lewin AS, Klinkenberg G, et al. Streptomyces tardus sp. nov.: a slow-growing actinobacterium producing candicidin, isolated from sediments of the trondheim fjord. Front Microbiol. (2021) 12:714233. doi: 10.3389/fmicb.2021.714233
230. Hobbs G, Frazer CM, Gardner DC, Cullum JA, Oliver SG. Dispersed growth of Streptomyces in liquid culture. Appl Microbiol Biotechnol. (1989) 31:272–7. doi: 10.1007/BF00258408
231. Glazebrook MA, Doull JL, Stuttard C, Vining LC. Sporulation of Streptomyces venezuelae in submerged cultures. Microbiol. (1990) 136:581–8. doi: 10.1099/00221287-136-3-581
232. Szponar B, Pawlik KJ, Gamian A, Dey ES. Protein fraction of barley spent grain as a new simple medium for growth and sporulation of soil actinobacteria. Biotechnol Lett. (2003) 25:1717–21. doi: 10.1023/A:1026046403010
233. Chae WB, Kim YB, Choi SW, Lee HB, Kim EK. Enhancing the sporulation of Streptomyces kasugaensis by culture optimization. Kor J Chem Eng. (2009) 26:438–43. doi: 10.1007/s11814-009-0074-1
234. Rho YT, Lee KJ. Kinetic characterization of sporulation in Streptomyces albidoflavus SMF301 during submerged culture. Microbiol. (1994) 140:2061–5. doi: 10.1099/13500872-140-8-2061
235. Zhou S, Hu X, Zhao P, Niu Y, Liao M. Optimization of sporulation conditions for Streptomyces galilaeus AF1. J Northwest A & F Univ Natural Sci Ed. (2019) 47:123–38.
236. Tian X, Zhao H, Tang C, Zong Z. Optimization of the fermentation of solid state medium for biocontrol actinomycetes 153 and its heat tolerance ability. J Northwest A & F Univ Natural Sci Ed. (2010) 38:181–6.
237. Liu H, Zhang D, Zhang X, Zhou C, Zhou P, Zhi Y. Medium optimization for spore production of a straw-cellulose degrading actinomyces strain under solid-state fermentation using response surface method. Sustainability. (2020) 12:8893. doi: 10.3390/su12218893
238. Hamedi J, Poorinmohammad N, Papiran R. Growth and life cycle of actinobacteria. In: Wink J, Mohammadipanah F, Hamedi J, editors. Biology and Biotechnology of Actinobacteria. Cham: Springer (2017). p. 29–50. doi: 10.1007/978-3-319-60339-1_3
239. Vurukonda SSKP, Giovanardi D, Stefani E. Plant growth promoting and biocontrol activity of Streptomyces spp. as endophytes. Int J Mol Sci. (2018) 19:952. doi: 10.3390/ijms19040952
240. Zamoum M, Goudjal Y, Sabaou N, Mathieu F, Zitouni A. Development of formulations based on Streptomyces rochei strain PTL2 spores for biocontrol of Rhizoctonia solani damping-off of tomato seedlings. Biocon Sci Technol. (2017) 27:723–38. doi: 10.1080/09583157.2017.1334257
241. Myo EM, Ge B, Ma J, Cui H, Liu B, Shi L, et al. Indole-3-acetic acid production by Streptomyces fradiae NKZ-259 and its formulation to enhance plant growth. BMC Microbiol. (2019) 19:1–14. doi: 10.1186/s12866-019-1528-1
242. Tamreihao K, Ningthoujam DS, Nimaichand S, Singh ES, Reena P, Singh SH, et al. Biocontrol and plant growth promoting activities of a Streptomyces corchorusii strain UCR3-16 and preparation of powder formulation for application as biofertilizer agents for rice plant. Microbiol Res. (2016) 192:260–70. doi: 10.1016/j.micres.2016.08.005
243. Chung WC, Huang JW, Huang HC. Formulation of a soil biofungicide for control of damping-off of Chinese cabbage (Brassica chinensis) caused by Rhizoctonia solani. Biol Cont. (2005) 32:287–94. doi: 10.1016/j.biocontrol.2004.10.011
244. Sabaratnam S, Traquair JA. Formulation of a Streptomyces biocontrol agent for the suppression of Rhizoctonia damping-off in tomato transplants. Biol Cont. (2002) 23:245–53. doi: 10.1006/bcon.2001.1014
245. Saberi-Riseh R, Moradi-Pour M. A novel encapsulation of Streptomyces fulvissimus Uts22 by spray drying and its biocontrol efficiency against Gaeumannomyces graminis, the causal agent of take-all disease in wheat. Pest Manag Sci. (2021) 77:4357–64. doi: 10.1002/ps.6469
246. Bhardwaj D, Ansari MW, Sahoo RK, Tuteja N. Biofertilizers function as key player in sustainable agriculture by improving soil fertility, plant tolerance and crop productivity. Microb Cell Factories. (2014) 13:1–10. doi: 10.1186/1475-2859-13-66
Keywords: actinobacteria, biocontrol, biofertilizers, endophytic actinobacteria, plant growth promotion, rhizoactinomycetes
Citation: Silva GC, Kitano IT, Ribeiro IAF and Lacava PT (2022) The Potential Use of Actinomycetes as Microbial Inoculants and Biopesticides in Agriculture. Front. Soil Sci. 2:833181. doi: 10.3389/fsoil.2022.833181
Received: 10 December 2021; Accepted: 24 January 2022;
Published: 21 February 2022.
Edited by:
Simone Raposo Cotta, University of São Paulo, BrazilReviewed by:
Adijailton José de Souza, University of São Paulo, BrazilCopyright © 2022 Silva, Kitano, Ribeiro and Lacava. This is an open-access article distributed under the terms of the Creative Commons Attribution License (CC BY). The use, distribution or reproduction in other forums is permitted, provided the original author(s) and the copyright owner(s) are credited and that the original publication in this journal is cited, in accordance with accepted academic practice. No use, distribution or reproduction is permitted which does not comply with these terms.
*Correspondence: Paulo Teixeira Lacava, cHRsYWNhdmFAdWZzY2FyLmJy
Disclaimer: All claims expressed in this article are solely those of the authors and do not necessarily represent those of their affiliated organizations, or those of the publisher, the editors and the reviewers. Any product that may be evaluated in this article or claim that may be made by its manufacturer is not guaranteed or endorsed by the publisher.
Research integrity at Frontiers
Learn more about the work of our research integrity team to safeguard the quality of each article we publish.