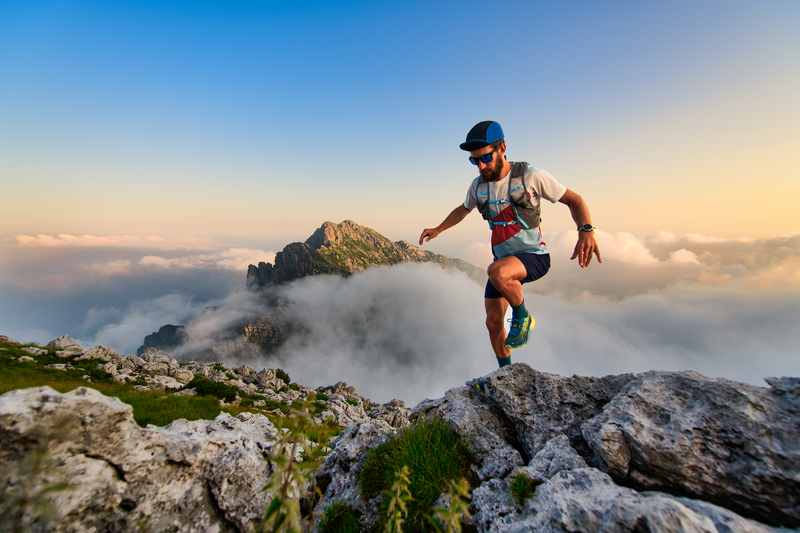
95% of researchers rate our articles as excellent or good
Learn more about the work of our research integrity team to safeguard the quality of each article we publish.
Find out more
ORIGINAL RESEARCH article
Front. Soil Sci. , 30 November 2021
Sec. Plant-Soil Interactions
Volume 1 - 2021 | https://doi.org/10.3389/fsoil.2021.757839
This article is part of the Research Topic Sustainable Phosphorus Use in Agriculture View all 6 articles
Phosphorus (P) is the second most important mineral nutrient for plant growth and plays a vital role in maintaining global food security. The natural phosphorus reserves [phosphate rock (PR)] are declining at an unprecedented rate, which will threaten the sustainable food supply in near future. Rendered animal byproducts such as meat and bone meal (MBM), could serve as a sustainable alternative to meet crop phosphorus demand. Even though nitrogen (N) from MBM is readily mineralized within a few days, >75% of the P in MBM is present as calcium phosphate that is sparingly available to plants. Thus, application of MBM with the aim of meeting crop N demand could result in buildup of P reserves in soil, which necessitates the need to improve the P mobilization from MBM to achieve higher plant P use efficiency. Here, we tested the potential of two microbial inoculum-arbuscular mycorrhizal fungi (AMF) and P solubilizing fungi (Penicillium bilaiae), in improving the mobilization of P from MBM and the subsequent P uptake by maize (Zea mays). Compared to the non-inoculated MBM control, the application of P. bilaiae increased the P mobilization from MBM by more than two-fold and decreased the content of calcium bound P in the soil by 26%. However, despite this mobilization, P. bilaiae did not increase the tissue content of P in maize. On the other hand, AMF inoculation with MBM increased the plant root, shoot biomass, and plant P uptake as compared to non-inoculated control, but did not decrease the calcium bound P fraction of the soil, indicating there was limited P mobilization. The simultaneous application of both AMF and P. bilaiae in association with MBM resulted in the highest tissue P uptake of maize with a concomitant decrease in the calcium bound P in the soil, indicating the complementary functional traits of AMF and P. bilaiae in plant P nutrition from MBM. Arbuscular mycorrhizal fungi inoculation with MBM also increased the plant photosynthesis rate (27%) and root phosphomonoesterase activity (40%), which signifies the AMF associated regulation of plant physiology. Collectively, our results demonstrate that P mobilization and uptake efficiency from MBM could be improved with the combined use of arbuscular mycorrhizal fungi and P. bilaiae.
Phosphorus (P) is a key nutrient for plant growth and thus its continued supply is critical for achieving global food security. More than 90% of raw material for P fertilizer is harvested from natural reserves of phosphate rock (PR), which is a non-renewable source (1, 2). The current global reserves of PR (16,000 MT), at the present consumption rate (160 MT year−1), are expected to deplete within the next 100 years (3, 4), which threatens sustainable food production. Low P use efficiency of crops (20–25%) exacerbates this problem, requiring constant addition of P fertilizers to meet their P demand. Better recycling of P from P-rich agricultural byproducts such as meat and bone meal (MBM) is a promising avenue to recapture and reuse the P in the agricultural production systems. The annual production of MBM is estimated to be 2.5 MT (5) and 18 MT (6) in the United State and Europe, respectively. Commercially available MBM is nutrient-rich and contains up to 8% nitrogen (N) and 5–10% P, and thus can be used as a potential N and P fertilizer (7). The N in MBM is in proteinaceous forms, which can be readily mineralized to and . However, more than 75% of P in MBM is calcium-bound P (as hydroxyapatite) with low bioavailability for plants (7). Application of MBM to supplement the N requirement of the crops could thus result in bioaccumulation of residual P in soil, which may facilitate the P contamination of water bodies from soil erosion. Thus, there is an urgent need to improve the mobilization and plant uptake of P from MBM for its sustainable, efficient, and economical use as an organic fertilizer.
Phosphorus acquisition and uptake by plants can be enhanced by modulating the activity of the microbial community in the rhizosphere (8). Soil microbiota with complementary functional traits can promote P mobilization and plant uptake through different mechanisms, including mineralization, solubilization of recalcitrant P by releasing organic acids, and mycorrhizae-mediated plant P uptake (8, 9). Among the potential P mobilizing soil microbes, mycorrhizal fungi are the most widely studied in relation to plant P nutrition (2). Arbuscular mycorrhizal fungi (AMF) are the most common mycorrhizal fungi, which form symbiotic associations with more than 80% of vascular plants (10). The AMF is an obligate biotroph and produces an extensive mycelial network in the soil, which plays an important role in plant uptake of soil nutrients, most notably P (10). Moreover, AMF provides additional benefits to plants through enhancing soil aggregate formation and stability (11), enhancing plant resistance to root pathogens (12), and improving plant-soil-water relations (13). The root-mycelial network explores a much larger soil volume than the roots alone, which is critical for the uptake of immobile nutrients like P (8, 10). Along with P foraging activity, AMF has also been reported to increase the mineralization rate of phosphate minerals through the release of chelating compounds and phosphatase enzymes (14, 15).
Apart from mycorrhizal fungi, phosphorus solubilizing microbes (PSM) can solubilize the recalcitrant P in the soil. Many of the calcium phosphates, including PR ores are insoluble in soil with respect to the release of inorganic P at rates necessary to support agronomic levels of plant productivity (16). A wide range of soil bacteria and fungi can solubilize the P from apatites and other mineral P forms (8, 17, 18). Soil bacteria and non-symbiotic fungi enhance the plant-available P in the soil through acidification, or by releasing organic acids or enzymes (19). Generally, the P solubilizing fungi produce more organic acids than bacteria per unit cell count and consequently exhibit greater P-solubilizing activity (20, 21). Among the P solubilizing fungi, Penicillium sp. are considered the most efficient P solubilizers. For example, Penicillium bilaiae (P. bilaiae), a native soil fungus that is commercially available (Jumpstart®) as a soil amendment, has the greater ability to increases P availability from PR through soil acidification as compared to other PSM's (22, 23). Solubility of apatites increases with a PSM-mediated decrease in soil pH (8). However, MBM mineralization increases the soil pH up to 8.5 (7), which can potentially hinder the acidification mediated P mobilization by PSM.
Soil inoculation with a combination of AMF and PSM can be a promising strategy for mobilization and uptake of residual plant unavailable soil P. The PSM can solubilize inorganic P minerals through acidification and chelation but lacks the ability to assist plant uptake of mobilized P from farther reaches of soil (24–27). On the other hand, AMF through their extensive hyphal network can facilitate the plant P uptake (28, 29) but have very limited ability to mobilize P from sparingly available P forms (30). Thus, both AMF and PSM can complement the plant uptake of soil P. The results from previous studies using a combination of AMF and PSM have been inconclusive, with neutral (24), synergistic (27) or antagonistic interactions (26). The AMF and PSM interact with each other for carbon (C) and P (31) and the outcome of this interaction (synergism or antagonism) depends upon the background P availability (26, 31). Under low soil C and P, AMF, and PSM can have antagonistic interactions for P mobilization from sparingly available P forms (26, 31). The MBM contains labile C and more than 10% of total P as labile organic P that can potentially alter the direction of AMF-PSM interactions toward synergism, which remains largely unexplored.
The objectives of the present work were to assess the potential of PSM (P. bilaiae) and AMF and their interactions in improving the P mobilization and plant uptake from the MBM. We hypothesize that, due to their complementary P mobilization and acquisition features, when applied together, the P. bilaiae and AMF will interact synergistically to improve the mobilization and uptake of P from MBM. We tested the hypothesis through lab incubation and greenhouse experiments with different combinations of AMF and P. bilaiae, using maize as the study species. We predicted that growth and tissue concentration of P of maize will be higher when MBM is applied in combination with AMF and P. bilaiae, than MBM with either one of the inoculums or MBM alone.
The MBM was obtained from Valley Proteins Inc. (Wards, SC, USA). It was sieved to a diameter <0.5 mm and subjected to an autoclaving treatment at 133°C for 40 min. Lipids in the MBM were extracted in a Soxhlet extractor using n-hexane as the solvent. Lipids are extracted from MBM for their potential use in the biofuel, lubricant, and pharmaceutical industries. Moreover, such a process can decrease the volume of MBM, lower the C:N:P ratio, and increase the fertilizer value of MBM (7). Extracted and powdered MBM was dried and stored at room temperature before further use. The extracted MBM contains a total of 11.3% P, measuring by calcination at 600°C for 1 h followed by acid digestion. Soil samples were collected from Simpson Research and Education Center, Pendleton, South Carolina, USA (34.623350 N, −82.730030 W). The soil was collected from 0 to 15 cm depth, air-dried, passed through a 2 mm sieve, autoclaved at 121°C for 1 h, and stored until further use. The soil was Cecil clay loam with pH, CEC, base saturation, and organic matter content of 5.6, 3.2 meq/100 g, 59, and 0.62%, respectively. The soil contained 33.18 and 65.01 kg/ha of plant-available P and potassium (K), respectively. Two separate experiments were conducted to assess the rate of P mobilization and plant uptake from MBM: (1) a lab experiment to evaluate the P mobilization capacity of P. bilaiae from MBM and (2) greenhouse experiments to test the effect of P. bilaiae and AMF alone and in combination to assess the P mobilization and plant P uptake from MBM. Phosphorus solubilizing fungi (P. bilaiae) was obtained from a commercially available P solubilizer product named Jumpstart® (Monsanto, USA). We purchased the AMF mixture of four fungal species including Glomus intraradices, Glomus mosseae, Glomus aggregatum, and Glomus etunicatum, from the commercial product named Myco apply (Mycorrhizae Applications, Oregon, USA). Idaho PR was used to compare the P mobilization capacity of P. bilaiae from MBM and PR for the lab incubation experiment was obtained from the International Ag Labs, Fairmont, Minnesota.
The lab incubation study was conducted to measure the P mobilization capacity of the P. bilaiae from MBM as per the approach reported by Wakelin et al. (32) for tri-calcium phosphate. Finely ground (50–250 μm size) MBM (0.1 g) and Idaho PR (equivalent amount P) were mixed with 100 ml of sterilized culture medium (0.1 g L−1 NaCl, 0.4 g L−1 NH4Cl, 0.78 g L−1, KNO3, 0.5 g L−1 MgSO4, 0.1 g L−1 CaCl2, and 10 g L−1 sucrose) in 250 ml Erlenmeyer flasks. The P. bilaiae culture (200 μg) was inoculated into five replicate flasks and incubated at 25°C in dark with continuously gentle shaking. Six treatments were included in this experiment: Control, Control + P. bilaiae, MBM, MBM + P. bilaiae, Idaho PR, and Idaho PR + P. bilaiae in a completely randomized design. After 7 days of incubation, solution pH, soluble and ergosterol content were measured.
A greenhouse experiment was conducted to measure the effect of AMF, P. bilaiae, and AMF + P. bilaiae on the P mobilization and plant P uptake from MBM. The pots (12 L) were filled with a 12 kg sand-soil (9:1) mixture with a bulk density of 1.543 g cm−3. The sand and soil were steam autoclaved at 121°C for 1 h. The low proportion of soil in the substrate was to minimize the background P content and was based on preliminary greenhouse experiments. The nutrient free white sand with a bulk density of 1.72 g cm−3 and pH of 7.5 was used in the study. A total of 6 treatments (control, MBM, MBM + AMF, MBM + P. bilaiae, MBM + AMF + P. bilaiae, and KH2PO4) were tested in a greenhouse experiment and replicated five times in a completely randomized design. The MBM was applied at a rate of 800 μg P pot−1. The AMF and P. bilaiae culture were applied at a rate of 25 g pot−1 (132 spores g−1) and 0.5 g pot−1 (8.0 * 108 spores g−1), respectively. The pots were filled in three layers with the first 3 cm layer without any P fertilizers and P mobilizer inoculum, middle 15 cm layer mixed with P fertilizers and AMF and P. bilaiae inoculum, and top 3 cm layer again without any P fertilizers and P mobilizer inoculum. All the other essential macro and micro-nutrients were applied using the modified 1x Hoagland solution (P free; 300 ml after every 4 days). The maize (Zea mays) was used as a test plant species for this experiment. Three maize seeds were sown in each pot and after germination thinned to one plant per pot. Plants were harvested 40 days after planting. Plant shoot, root biomass, shoot, root P uptake, percent root mycorrhizae colonization, root morphology, plant photosynthesis rate, stomatal conductance, root acid phosphomonoesterase activity, soil P fractionation, and soil acid, alkaline phosphomonoesterase activity, were measured.
Ergosterol (lab incubation) was extracted as reported by (33). Thirty milliliters of the incubation solution was filtered through a 0.45 μm nitrocellulose filter paper. The filter paper along with filtrate was dissolved into 0.14 M methanolic KOH and incubated at 82°C for 50 min in dark. After the incubation, samples were placed on the ice for 5 min and acidified to a pH < 2 with 0.5 ml of 50% HCl. To the acidified samples, 2 ml of hexane was added and vortexed for 5 min under dark conditions. The top hexane phase (1.5 ml) was collected into the glass vials and dried under a vacuum. The dried contents were reconstituted in 20 μl of chloroform + 80 μl of methanol and transferred into glass vials with 200 μl inserts. The control samples were spiked with 0.5 μg/ml of ergosterol standard. Ergosterol was quantified using high-pressure liquid chromatography (HPLC). Separation of ergosterol compounds was performed on an Onyx C18 column (monolithic silica, 130 Å; 100 × 4.6 mm I.D.; Phenomenex, Torrance, CA) using a constant flow of methanol-water (99:1, v/v) solvent at a rate of 1 ml min−1. Ergosterol was detected at 282 nm absorbance using LC-UV and quantified using ergosterol standards.
Soil P fractionation was carried out according to the method reported by Hedley et al. (34). The soil at the time of harvest was subjected to sequential chemical extraction with 1 M NH4Cl, 0.5 M NaHCO3, chloroform fumigation + 0.5 M NaHCO3, 0.1 M NaOH, and 1 M HCl, in the specified order. Soil (4 g on a dry weight basis) was extracted with 40 ml of different extractants for 16 h. After extraction, the soil slurry was centrifuged at 1,509 g for 10 min and the supernatant was filtered through a Whatman No. 1 filter paper. The supernatant for 0.5 M NaHCO3 and 0.1 M NaOH extractions was further filtered through 0.45 μm syringe filters to remove humic acid. The clear filtrate was stored at −20°C until further use. The soluble P from each fraction was determined by the molybdenum blue/ ascorbic acid method (35). The extraction procedure fractionates the P into loosely sorbed-P (NH4Cl-P), olsen-P (NaHCO3-P), microbial bound P (chloroform fumigation + NaHCO3-P), Fe/Al-P (NaOH-P), and calcium bound-P [HCl-P (34)]. Soil pH was measured in water: soil solution with a 2:1 (v:w) ratio on the pH meter (Mettler Toledo, USA).
Soil acid and alkaline phosphomonoesterase activity were measured according to the method reported by Jatana et al. (7). Soil (1 g on a dry weight basis) immediately after harvest was mixed with 4 ml of modified universal buffer [MUB; 2.42 g tris(hydroxymethyl)aminomethane, 2.32 g maleic acid, 2.8 g citric acid, 1.26 g boric acid in 96.7 ml 1 N NaOH in total 1 L] of pH 6.5 (with 0.1 M HCl; acid phosphomonoesterase) or pH 11.0 (with 0.1 M NaOH; alkaline phosphomonoesterase). The soil buffer solution was incubated in the water bath for 1 h at 37°C after mixing with 1 ml of 0.05 M disodium p-nitrophenyl phosphate hexahydrate (prepared in the same MUB for acid and alkaline phosphomonoesterase). After the incubation, the reaction was immediately terminated by adding 1 ml of 0.5 M CaCl2 and 4 ml of 0.5 M NaOH. The soil suspension was then centrifuged at 1509 g for 10 min. The clear solution was filtered through a Whatman No. 1 filter paper. The absorbance of the clear supernatant was measured at 410 nm on a spectrophotometer (DU® 640 spectrophotometer, Beckman Coulter, Fullerton, CA, USA). The substrate solution (disodium p-nitrophenyl phosphate hexahydrate) was added to the control after the addition of 1 ml 0.5 M CaCl2 and 4 ml 0.5 M NaOH. Both enzyme activities were expressed as μg p-nitrophenol (PNP) g−1 dry soil h−1.
Root morphology of plants at harvest was evaluated by imaging the roots with Win-RHIZO version 3.9 (Regent Instruments Inc.). Specifically, roots were physically separated into different root types (primary, crown, and lateral roots) and the root images were taken in a plexiglass tray (0.2 × 0.3 m). The images were scanned through the Win-RHIZO system. After the root image analysis, the root samples were dried to calculate the specific root length (SRL; root length/dry weight of the roots).
Percent root mycorrhizal colonization was observed using the trypan blue staining procedure reported by Frater et al. (36). Roots were cleaned in a 10% KOH solution at 60°C for 24 h. After cleaning, the roots were washed with deionized water for 10 min followed by acidification in 1% HCl for 20 min. The acidified roots were soaked in trypan blue (0.05%, trypan blue powder) solution made in 1:1:1 (v:v:v) glycerol, lactic acid, water for 5 min on a hot plate set at 90°C. The roots were taken out of the trypan blue solution and washed gently under deionized water for 10 min. The washed roots were kept in deionized water at 4°C for a couple of days to remove the excess trypan dye present in the roots. We then assessed the percentage of root colonized by AMF using the gridline intercept technique (37). This technique determines the presence/absence of fungal organs (i.e., arbuscules/hyphae/vesicles) for each instance that a root intersected with a gridline.
Root phosphomonoesterase activity was measured as reported by Venterink (38). Roots tips were collected after thorough washing with deionized water. The washed root tips were immediately frozen in dry ice and stored at −80°C for later analysis. Frozen root tips were ground into a fine powder, weighed (250 mg), and homogenized at 6,000 rpm for 2 min in 500 μl (1:2, m/v) of MUB [100 mM tris(hydroxymethyl)aminomethane, 100 mM maleic acid, 5 mM citric acid, and 100 mM boric acid; pH 5.5 for acid phosphomonoesterase]. Homogenized root tissues were centrifuged at 14,000 g for 15 min at 4°C and the supernatant was collected. To the 300 μl of supernatant, 4 ml of MUB and 1 ml of 0.003 M p-nitrophenyl phosphate hexahydrate were added and vortexed for 30 s. The mixture was then incubated at 37°C for 10 min in a water bath. After the incubation, the reaction was terminated by adding 1 ml 0.5 M CaCl2 and 4 ml 0.5 M NaOH. The precipitates were recovered by centrifugation at 12,000 g for 15 min. The PNP content of the supernatant was quantified spectrophotometrically (DU® 640 spectrophotometer, Beckman Coulter, Fullerton, CA, USA) at 410 nm by using an authentic standard (PNP). Enzyme activity was expressed as μg pPNP g−1 root h−1.
Net photosynthetic rate and stomatal conductance of individual leaves were measured between 09:00 a.m. and 12:00 p.m. at 18 and 36 days after planting. Two recently fully opened leaves were selected from each plant and analyzed using a portable photosynthesis system (model LI-6400, LI-COR, Lincoln, NE, USA). The leaf blade width was measured before inserting the maize leaf into the chamber to account for the leaf area. The LI-6400 was programmed with a constant leaf chamber block temperature at 25°C. A fixed substrate level of 400 mm3 m−1 CO2 (12-g cartridge) and an LED 6,400 R/B light at 1,500 μmol m−2 s −1 were provided.
The laboratory and greenhouse experiments were conducted in a completely randomized design with five replicates. The one-way analysis of variance was performed on the data using JMP Pro 13.1.0 (SAS Institute Inc., Cary NC). Different treatment pairs were compared using the Tukey HSD test. The treatments were considered significantly different at the P < 0.05 level. The data for the percent mycorrhizae colonization were log-transformed to meet the assumption of normality before the analysis of variance.
The MBM contained three major mineral forms of P, which were water-soluble P, organic P, and calcium bound P (Figure 1). The major mineral form of P in MBM was calcium bound P (>75%). Approximately 15% of the P was present as organic P in MBM (Figure 1). MBM mineralization increased the pH of the incubation solution from 5.9 to 7.1. The increase in soil pH was largely due to the N mineralization from MBM. However, MBM + P. bilaiae significantly decreased the solution pH to 4.2 (Figure 2A). In the absence of P. bilaiae, we observed a similar amount of P mobilization from MBM and Idaho PR. By contrast, when P. bilaiae was present in the incubation solution, 34% higher amount of P mobilized from MBM + P. bilaiae as compared to Idaho PR + P. bilaiae (Figure 2B). Moreover, the highest ergosterol content was observed in the MBM + P. bilaiae (Figure 2C).
Figure 1. Different mineral forms of phosphorus (P) in MBM. Different alphabetic letters signify the statistical difference (Tukey test, p < 0.05) across the different mineral forms of P. Error bar represents the mean ± SD (n = 5).
Figure 2. Solution pH (A), PO4− (B), and ergosterol (C) content as affected by different P fertilizers and P. bilaiae at 7 days after incubation. Different alphabetical letters signify the statistical difference (Tukey test, p < 0.05) across the treatments. Error bar represents the mean ± SD (n = 5).
The application of KH2PO4 recorded the highest shoot and root biomass and tissue P uptake (Figure 3). The MBM treatment recorded significantly higher shoot, root biomass, and tissue uptake of P into shoot and root, as compared to control (p < 0.0001; Figure 3). The application of MBM + AMF + P. bilaiae recorded significantly higher plant shoot (24%), root (29%) biomass, and shoot and root uptake of P as compared to MBM alone (Figure 3). However, the application of MBM + P. bilaiae did not have any effect on the shoot, root biomass, and tissue P uptake (Figure 3).
Figure 3. Plant shoot (A), root biomass (B), shoot P (C), and root P uptake (D) as affected by different P fertilizers and P mobilizers (arbuscular mycorrhizae fungi (AMF) and P. bilaiae) at 40 days after planting. Different alphabetical letters signify the statistical difference (Tukey test, p < 0.05) across the treatments. Error bar represents the mean ± SD (n = 5).
The highest SRL for the primary roots of the maize was recorded in control and MBM + different P mobilizers (AMF, P. bilaiae, AMF + P. bilaiae) as compared to KH2PO4 treatment (Figure 4A). The MBM with different P mobilizers (AMF, P. bilaiae, AMF + P. bilaiae) had significantly higher SRL (195, 163, and 162% higher, respectively) for the primary roots as compared to MBM alone (Figure 4A). However, the MBM treatment did not have any effect on the SRL of the crown roots (Figure 4B). The highest SRL for the lateral roots was observed in MBM with and without different P mobilizers as compared to KH2PO4 (Figure 4C). The highest root acid phosphomonoesterase activity was observed in the MBM with different P mobilizers (AMF, P. bilaiae, AMF + P. bilaiae) as compared to MBM alone, control, and KH2PO4 treatment (Figure 4D). Application of different P mobilizers (AMF, P. bilaiae, AMF + P. bilaiae) with MBM significantly increased the acid phosphomonoesterase activity by 24.5, 25.4, and 26.2%, respectively, as compared to MBM alone (Figure 4D). The root AMF colonization was significantly higher in the MBM + AMF and MBM + AMF + P. bilaiae as compared to all other treatments (Figure 4E).
Figure 4. Specific root length for primary (A), crown (B), lateral (C) roots, root acid phosphomonoesterase activity (D), and percent root arbuscular mycorrhizae colonization (E) as affected by different P fertilizers and P mobilizers (arbuscular mycorrhizae fungi (AMF) and P. bilaiae) at 40 days after planting. Different alphabetical letters signify the statistical difference (Tukey test, p < 0.05) across the treatments. Error bar represents the mean ± SD (n = 5).
The KH2PO4 treatment recorded the highest photosynthetic rate and stomatal conductance as compared to MBM and control at 18 days after sowing (Figure 5). However, at 36 days after sowing, the photosynthetic rate and stomatal conductance of the maize in the MBM alone treatment was similar to that in the KH2PO4 (Figure 5). The MBM + AMF treatment recorded significantly higher plant photosynthetic rate and stomatal conductance as compared to MBM alone at both 18 and 36 days after sowing and as compared to KH2PO4 at 36 days after sowing (Figure 5).
Figure 5. Plant photosynthesis rate (A) and stomatal conductance (B) as affected by different P fertilizers and P mobilizers (arbuscular mycorrhizae fungi (AMF) and P. bilaiae). Different alphabetical letters signify the statistical difference (Tukey test, p < 0.05) across the treatments. Error bar represents the mean ± SD (n = 5).
The soil content of loosely sorbed P (Figure 6A) and olsen P (Figure 6B) was higher in the KH2PO4 treatment as compared to MBM and control. The MBM + P. bilaiae recorded significantly higher loosely sorbed (194%) and olsen P (61%) content as compared to MBM alone (Figure 6). The MBM fertilization significantly increased the microbial P content (p < 0.0001; Figure 6D) in the soil fraction as compared to control and KH2PO4 treatments. The highest amount of microbial P was observed in the MBM + P. bilaiae treatment followed by the treatment with the mixture of MBM + AMF + P. bilaiae as compared to MBM alone (Figure 6D). The calcium bound P was the predominant form of P in MBM (118 μg PO4 g−1 soil) treatment as compared to KH2PO4 (>10 μg PO4 g−1 soil) and control (Figure 6E). However, the treatments of MBM + P. bilaiae (87 μg PO4 g−1 soil) and MBM + AMF + P. bilaiae (85 μg PO4 g−1 soil), had significantly lower soil content of calcium bound P (p < 0.0001), as compared to MBM alone (Figure 6E). The major P fraction in KH2PO4 treatments was tissue uptake P (approximately 23%; Figure 7). However, in the MBM treatments, the major fraction of P was calcium bound P (46% of total applied P) that was unavailable to plants (Figure 7). Approximately 20–25% of P applied to pots did not attribute to any fraction (Figure 7). The application of MBM + P. bilaiae significantly decreased the pH of the soil (5.4) as compared to all other treatments (Figure 6F).
Figure 6. Mineral P forms, loosely sorbed P (A), olsen P (B), Fe/Al bound P (C), microbial biomass P (D), Ca bound P (E), and soil pH (F) as affected by different P fertilizers and P mobilizers (arbuscular mycorrhizae fungi (AMF) and P. bilaiae) at 40 days after planting. Different alphabetical letters signify the statistical difference (Tukey test, p < 0.05) across the treatments. Error bar represents the mean ± SD (n = 5).
Figure 7. Soil P fractionation (% of total P applied) as affected by different P fertilizers and P mobilizers (arbuscular mycorrhizae fungi (AMF) and P. bilaiae) at 40 days after planting.
The application of MBM significantly increased the acid phosphomonoesterase activity as compared to control and KH2PO4 (p < 0.0001; Figure 8A). The application of different P mobilizers (AMF, P. bilaiae, and AMF + P. bilaiae) with MBM significantly increased the acid phosphomonoesterase activity by 61, 44, and 55%, respectively as compared to MBM alone (Figure 8A). However, different treatments had no significant effect on the soil's alkaline phosphomonoesterase activity (Figure 8B).
Figure 8. Soil potential acid (A) and alkaline (B) phosphomonoesterase activity as affected by different P fertilizers and P mobilizers (arbuscular mycorrhizae fungi (AMF) and P. bilaiae) at 40 days after planting. Different alphabetical letters signify the statistical difference (Tukey test, p < 0.05) across the treatments. Error bar represents the mean ± SD (n = 5).
A higher proportion of P is present as calcium bound P in the MBM (Figure 1), which is unavailable to plants. Due to the potential to increase the soil pH during initial N mineralization, the application of concentrated organic N and P fertilizes such as MBM can hamper the P mobilization from Ca-bound P fractions. Hence, P. bilaiae that counteracts the pH increase, can increase the rate of P mineralization from MBM, and has been considered as one of the most important P mobilizers for the field-grown crop plants (32). In our lab incubation study, mineralization of MBM increased the pH of the incubation media, which is consistent with previous observations (7). As reported from previous studies with calcium phosphate (32), the application of P. bilaiae increased the P mobilization rate from MBM (Figure 2A). Even though the exact mechanism of P mobilization by P. bilaiae in our study is not evident, based on the previous studies (8, 32, 39, 40), we propose that H+ and organic acids released by the P. bilaiae to facilitate this process. This is partly evident from the lower soil solution pH in MBM + P. bilaiae treatments (Figure 2B). The labile C in MBM could act as an energy source for P. bilaiae and stimulate a greater fungal biomass production, which in turn will facilitate greater mobilization of P from MBM. This in turn is supported by the higher soil ergosterol content (Figure 2C), a fungal biomarker, in MBM + P. bilaiae treatments. Thus, higher P. bilaiae biomass primed by C availability could have resulted in higher P mobilization from MBM.
In accordance with our hypothesis, the application of P. bilaiae + AMF in MBM treatments significantly increased the P uptake (Figures 3C,D). The synergistic interaction between the PSMs and AMF for the plant P uptake has been reported in previous studies (27, 41, 42). Our study showed that the synergistic interaction of AMF and PSMs for plant P uptake from MBM might be largely related to their complementary functional traits. Phosphorus solubilizing microbes possessed the capacity to mobilize the P from recalcitrant P fractions, while AMF could help plants to explore the soil for mobilized P that are beyond the root system (27, 41). This notion is supported by the similar tissue P content of maize in MBM + P. bilaiae as that of the non-inoculated MBM (Figure 3), despite a higher soil labile P and lower Ca bound P (Figure 6). On the other hand, MBM + AMF + P. bilaiae treatment resulted in a higher tissue P content despite having similar soil labile P, and Ca-bound P fraction as that of MBM + P. bilaiae treatment.
Along with mycorrhizae and PSM mediated P mobilization and uptake, plants also mobilize P from sparingly available P forms by modulating their root morphology and physiology (43–45). In our study, we observed higher SRL of the primary and lateral roots (morphological response) and root acid phosphomonoesterase activity (physiological response) in MBM treatment (Figure 4). Thinner roots under P deficiency are efficient at exploring larger soil volume per unit root biomass (46–48). Also, plant root morphology is closely linked to the plant root exudation such as the release of organic acids and phosphatases (43–45). Fine roots are known to exude organic acids and phosphomonoesterases, phosphodiesterases, and phytases for the mobilization and mineralization of P (49). Such relations between thinner roots and activity of phoshomonoesterases would work synergistically under P deficiency in maximizing the acquisition of immobile P (47, 49). The P mobilizers (AMF, P. bilaiae, and AMF + P. biliaiae) contributed to the highest SRL for primary roots and root acid phosphomonoesterase activity in MBM treatment (Figure 4), which indicates that the interaction effect of sparingly available P and P mobilizers might further regulate the plant root morphological and physiological response to P availability. The ability of plants to modify the composition of root exudation as a function of the chemical form of P source has been recently reported (2, 50, 51).
Similar to that observed in the roots, we observed higher soil acid phosphomonoesterase activity in MBM treatment. Phosphomonoesterases, which are exudated by plants and microbes, can mineralize the P from organic fractions of MBM (Figure 9). Higher activity of phosphomonoesterases can indicate the higher mineralization rate of organic P into H2/ (32). However, the increase in the activity of acid phosphomonoesterases, but not the alkaline phosphomonoesterases, might be due to lower soil pH, as acid phosphomonoesterase are more active at soil pH lower than 7 (52).
Figure 9. Schematic diagram representing the different MBM_ phosphorous fractions and arbuscular mycorrhizae fungi, Penicillium bilaiae and their interaction effect for the mobilization of different MBM_P fractions.
Our study highlights the synergistic effects of AMF and P. bilaiae in improving the mobilization and plant uptake of P from MBM. The synergistic effect of the AMF and P. bilaiae for P mobilization from MBM was attributed to their complementary functional traits and through the modulation of the root morphology and biochemical properties of roots and soil pH, which in turn lead to a greater P acquisition of maize from MBM. The MBM formulations with AMF and P. bilaiae can potentially increase the P fertilization value of MBM apart from its already established N fertilization potential. Such a strategy can help in maximizing the MBM utilization along with minimizing the potential of MBM in creating residual P pollution in the soils.
The original contributions presented in the study are included in the article/supplementary material, further inquiries can be directed to the corresponding author/s.
BJ and NT designed the experiment. BJ conducted the experiment, collected, analyzed the data, and wrote the first draft of the manuscript. All authors contributed to the manuscript revisions.
This research project was funded by the United States Department of Agriculture (USDA NIFA award: 2016-67020-25264).
The authors declare that the research was conducted in the absence of any commercial or financial relationships that could be construed as a potential conflict of interest.
All claims expressed in this article are solely those of the authors and do not necessarily represent those of their affiliated organizations, or those of the publisher, the editors and the reviewers. Any product that may be evaluated in this article, or claim that may be made by its manufacturer, is not guaranteed or endorsed by the publisher.
We would like to thank Ziliang Zhang for his feedback on the earlier version of the manuscript.
1. Cordell D, Drangert JO, White S. The story of phosphorus: global food security and food for thought. Glob Environ Change. (2009) 19:292–305. doi: 10.1016/j.gloenvcha.2008.10.009
2. Efthymiou A, Jensen B, Jakobsen I. The roles of mycorrhiza and Penicillium inoculants in phosphorus uptake by biochar-amended wheat. Soil Biol Biochem. (2018) 127:168–77. doi: 10.1016/j.soilbio.2018.09.027
3. Cooper J, Lombardi R, Boardman D, Carliell-Marquet C. The future distribution and production of global phosphate rock reserves. Resour Conserv Recycl. (2011) 57:78–86. doi: 10.1016/j.resconrec.2011.09.009
4. Vassilev N, Martos E, Mendes G, Martos V, Vassileva M. Biochar of animal origin: a sustainable solution to the global problem of high-grade rock phosphate scarcity? J. Sci Food Agri. (2013) 93:1799–804. doi: 10.1002/jsfa.6130
5. Swisher K. Market report: prices are down but demand remains strong. Render Magazine. (2016) 45:10–5.
6. Moller K, Oberson A, Bünemann EK, Cooper J, Friedel JK, Glaesner N, et al. Improved phosphorus recycling in organic farming: navigating between constraints. Adv Agron. (2018) 147:159–237. doi: 10.1016/bs.agron.2017.10.004
7. Jatana BS, Kitchens C, Ray C, Tharayil N. Regulating the nutrient release rates from proteinaceous agricultural byproducts using organic amendments and its effect on soil chemical and microbiological properties. Biol Fert Soils. (2020) 56:747–58. doi: 10.1007/s00374-020-01446-z
8. Sánchez-Esteva S, Gómez-Muñoz B, Jensen LS, de Neergaard A, Magid J. The effect of Penicillium bilaii on wheat growth and phosphorus uptake as affected by soil pH, soil P and application of sewage sludge. Chem Biol Tech Agric. (2016) 3:21. doi: 10.1186/s40538-016-0075-3
9. Richardson AE. Prospects for using soil microorganisms to improve the acquisition of phosphorus by plants. Australian J Plant Physiol. (2001) 28:897–906. doi: 10.1071/PP01093
10. Smith SE, Read DJ. Mycorrhizal Symbiosis. 3rd ed. New York, NY: Academic Press. (2008) p. 1–769. doi: 10.1016/B978-012370526-6.50002-7
11. Rillig MC, Mummey DL. Mycorrhizas and soil structure. New Phytol. (2006) 17:41–53. doi: 10.1111/j.1469-8137.2006.01750.x
12. Borowicz VA. Do arbuscular mycorrhizal fungi alter plant–pathogen relations?. Ecology. (2001) 8:23057–68. doi: 10.2307/2679834
13. Auge RM. Water relations, drought and vesicular-arbuscular mycorrhizal symbiosis. Mycorrhiza. (2001) 11:3–42. doi: 10.1007/s005720100097
14. Joner EJ, Johansen A. Phosphatase activity of external hyphae of two arbuscular mycorrhizal fungi. Mycological Res. (2000) 104:81–6. doi: 10.1017/S0953756299001240
15. Wang W, Shi J, Xie Q, Jiang Y, Yu N, Wang E. Nutrient exchange and regulation in arbuscular mycorrhizal symbiosis. Mol plant. (2017) 10:1147–58. doi: 10.1016/j.molp.2017.07.012
16. Khan AA, Jilani G, Akhtar MS, Naqvi SMS, Rasheed M. Phosphorus solubilizing bacteria: occurrence, mechanisms and their role in crop production. J Agric Biol Sci. (2009) 1:48–58.
17. Rodriguez H, Fraga R. Phosphate solubilizing bacteria and their role in plant growth promotion. Biotech Adv. (1999) 17:319–39. doi: 10.1016/S0734-9750(99)00014-2
18. Whitelaw MA. Growth promotion of plants inoculated with phosphate solubilizing fungi. Adv Agron. (2000) 69:99–151. doi: 10.1016/S0065-2113(08)60948-7
19. Zaidi A, Khan SM, Ahemad M. Recent advances in plant growth promotion by phosphate-solubilizing microbes. In: Khan MS, editor. Microbial Strategies for Crop Improvement. New York, NY: Springer (2009). p. 325–51. doi: 10.1007/978-3-642-01979-1_2
20. Reddy MS, Kumar SK, Babita MSR. Biosolubilization of poorly soluble rock hosphatesby Aspergillus tubingensis and Aspergillus niger. Bioresource Technol. (2002) 84:187–9. doi: 10.1016/S0960-8524(02)00040-8
21. Venkateswarlu B, Rao AV, Raina P, Ahmad N. Evaluation of phosphorus solubilization by microorganisms isolated from arid soil. J Indian Soc Soil Sci. (1984) 32:273–7.
22. Cunningham JE, Kuiack C. Production of citric and oxalic acids and solubilization of calcium phosphate by Penicillium bilaii. Appl Environ Microbiol. (1992) 58:1451–8. doi: 10.1128/aem.58.5.1451-1458.1992
23. Vessey JK, Heisinger KG. Effect of Penicillium bilaii inoculation and phosphorus fertilization on root and shoot parameters of field-grown pea. Canadian J Plant Sci. (2001) 31:361–6. doi: 10.4141/P00-083
24. Chandanie WA, Kubota M, Hyakumachi M. Interactions between the arbuscular mycorrhizal fungus Glomus mosseae and plant growth-promoting fungi and their significance for enhancing plant growth and suppressing damping-off of cucumber (Cucumis sativus L.). Appl. Soil. Ecol. (2009) 41:336–41. doi: 10.1016/j.apsoil.2008.12.006
25. Omar SA. The role of rock-phosphate-solubilizing fungi and vesicular–arbusular-mycorrhiza (VAM) in growth of wheat plants fertilized with rock phosphate. World J Microbiol Biotech. (1997) 14:211–8.
26. Osorio NW, Habte M. Effect of a phosphate-solubilizing fungus and an arbuscular mycorrhizal fungus on leucaena seedlings in tropical soils with contrasting phosphate sorption capacity. Plant Soil. (2015) 389:375–85. doi: 10.1007/s11104-014-2357-5
27. Singh S, Kapoor KK. Inoculation with phosphate-solubilizing microorganisms and a vesicular-arbuscular mycorrhizal fungus improves dry matter yield and nutrient uptake by wheat grown in a sandy soil. Biol Fertil Soils. (1999) 28:139–44. doi: 10.1007/s003740050475
28. Barea JM, Pozo MJ, Azcón R, Azcón-Aguilar C. Microbial co-operation in the rhizosphere. J Exp Bot. (2005) 56:1767–78. doi: 10.1093/jxb/eri197
29. Toro M, Azcón R, Barea JM. Improvement of arbuscular mycorrhiza development by inoculation of soil with phosphate-solubilizing rhizobacteria to improve rock phosphate bioavailability (32P) and nutrient cycling. Appl Environ Microb. (1997) 63:4408–12. doi: 10.1128/aem.63.11.4408-4412.1997
30. Javaid A. Arbuscular mycorrhizal mediated nutrition in plants. J Plant Nutr. (2009) 32:1595–618. doi: 10.1080/01904160903150875
31. Zhang L, Xu M, Liu Y, Zhang F, Hodge A, Feng G. Carbon and phosphorus exchange may enable cooperation between an arbuscular mycorrhizal fungus and a phosphate-solubilizing bacterium. New Phytol. (2016) 210:1022–32. doi: 10.1111/nph.13838
32. Wakelin SA, Warren RA, Harvey PR, Ryder MH. Phosphate solubilization by Penicillium spp. closely associated with wheat roots. Biol Fertil Soils. (2004) 40:36–43. doi: 10.1007/s00374-004-0750-6
33. Gessner MO, Chauvet E. Ergosterol-to-biomass conversion factors for aquatic hyphomycetes. Appl Environ Microbiol. (1993) 59:502–7. doi: 10.1128/aem.59.2.502-507.1993
34. Hedley MJ, Stewart JWB, Chauhan B. Changes in inorganic and organic soil phosphorus fractions induced by cultivation practices and by laboratory incubations. Soil Sci Soc America J. (1982) 46:970–6. doi: 10.2136/sssaj1982.03615995004600050017x
35. Kaiserli A, Voutsa D, Samara C. Phosphorus fractionation in lake sediments–Lakes Volvi and Koronia, N. Greece. Chemosphere. (2002) 46:1147–55. doi: 10.1016/S0045-6535(01)00242-9
36. Frater PN, Borer ET, Fay PA, Jin V, Knaeble B, Seabloom E, et al. Nutrients and environment influence arbuscular mycorrhizal colonization both independently and interactively in Schizachyrium scoparium. Plant Soil. (2018) 425:493–506. doi: 10.1007/s11104-018-3597-6
37. Giovannetti M, Mosse B. An evaluation of techniques for measuring vesicular arbuscular mycorrhizal infection in roots. New phytologist. (1980) 1:489–500. Available online at: https://www.jstor.org/stable/2432123
38. Venterink HO. Legumes have a higher root phosphatase activity than other forbs, particularly under low inorganic P and N supply. Plant Soil. (2011) 347:137–46. doi: 10.1007/s11104-011-0834-7
39. Adhya TK, Kumar N, Reddy G, Podile AR, Bee H, Samantaray B. Microbial mobilization of soil phosphorus and sustainable P management in agricultural soils. Curr Sci. (2015) 108:1280–7.
40. Shen J, Yuan L, Zhang J, Li H, Bai Z, Chen X, et al. Phosphorus dynamics: from soil to plant. Plant Physiol. (2011) 156:997–1005. doi: 10.1104/pp.111.175232
41. Dutta SC, Neog B. Inoculation of arbuscular mycorrhizal fungi and plant growth promoting rhizobacteria in modulating phosphorus dynamics in turmeric rhizosphere. Natl Acad Sci Lett. (2017) 40:445–9. doi: 10.1007/s40009-017-0582-1
42. Mahanta D, Rai RK, Dhar S, Varghese E, Raja A, Purakayastha TJ. Modification of root properties with phosphate solubilizing bacteria and arbuscular mycorrhiza to reduce rock phosphate application in soybean-wheat cropping system. Ecol Eng. (2018) 111:31–43. doi: 10.1016/j.ecoleng.2017.11.008
43. Ismail AM, Heuer S, Thomson MJ, Wissuwa M. Genetic and genomic approaches to develop rice germplasm for problem soils. Plant Mol Biol. (2007) 65:547–70. doi: 10.1007/s11103-007-9215-2
44. Jain A, Vasconcelos MJ, Raghothama KG, Sahi SV. Molecular mechanisms of plant adaptation to phosphate deficiency. In: Janick J, editor. Plant Breeding Reviews. Vol. 29. (2007) p. 359–419. doi: 10.1002/9780470168035.ch7
45. Lynch JP. Roots of the second green revolution. Aust J Bot. (2007) 55:493–512. doi: 10.1071/BT06118
46. Liu B, Li H, Zhu B, Koide RT, Eissenstat DM, Guo D. Complementarity in nutrient foraging strategies of absorptive fine roots and arbuscular mycorrhizal fungi across 14 coexisting subtropical tree species. New Phytol. (2015) 208:125–36. doi: 10.1111/nph.13434
47. Lugli LF, Andersen KM, Aragão LE, Cordeiro AL, Cunha HF, Fuchslueger L, et al. Multiple phosphorus acquisition strategies adopted by fine roots in low-fertility soils in Central Amazonia. Plant Soil. (2020) 450:49–63. doi: 10.1007/s11104-019-03963-9
48. Rubio G, Oesterheld M, Alvarez CR, Lavado RS. Mechanisms for the increase in phosphorus uptake of waterlogged plants: soil phosphorus availability, root morphology and uptake kinetics. Oecologia. (1997) 112:150–5. doi: 10.1007/s004420050294
49. Richardson AE, Hadobas P. A., Hayes J. E. (2000). Acid phosphomonoesterase and phytase activities of wheat (Triticum aestivum L.) roots and utilization of organic phosphorus substrates by seedlings grown in sterile culture. Plant Cell Environ. 23, 397–405. doi: 10.1046/j.1365-3040.2000.00557.x
50. Watts-Williams SJ, Smith FA, Jakobsen I. Soil phosphorus availability is a driver of the responses of maize (Zea mays) to elevated CO2 concentration and arbuscular mycorrhizal colonization. Symbiosis. (2019) 77:73–82. doi: 10.1007/s13199-018-0573-0
51. Edayilam N, Montgomery D, Ferguson B, Maroli AS, Martinez N, Powell BA, Tharayil N. Phosphorus stress-induced changes in plant root exudation could potentially facilitate uranium mobilization from stable mineral forms. Environ Sci Technol. (2018) 52:7652–62. doi: 10.1021/acs.est.7b05836
Keywords: arbuscular mycorrhizal fungi, maize, meat and bone meal, phosphorus solubilizing microbes, soil phosphorus, synergistic effect
Citation: Jatana BS, Kitchens C, Ray C, Gerard P and Tharayil N (2021) Dual Inoculation With Arbuscular Mycorrhizal Fungi and Phosphorus Solubilizing Fungi Synergistically Enhances the Mobilization and Plant Uptake of Phosphorus From Meat and Bone Meal. Front. Soil Sci. 1:757839. doi: 10.3389/fsoil.2021.757839
Received: 12 August 2021; Accepted: 24 September 2021;
Published: 30 November 2021.
Edited by:
Tales Tiecher, Federal University of Rio Grande Do Sul, BrazilReviewed by:
Mahaveer P. Sharma, ICAR Indian Institute of Soybean Research, IndiaCopyright © 2021 Jatana, Kitchens, Ray, Gerard and Tharayil. This is an open-access article distributed under the terms of the Creative Commons Attribution License (CC BY). The use, distribution or reproduction in other forums is permitted, provided the original author(s) and the copyright owner(s) are credited and that the original publication in this journal is cited, in accordance with accepted academic practice. No use, distribution or reproduction is permitted which does not comply with these terms.
*Correspondence: Nishanth Tharayil, bnRoYXJheUBjbGVtc29uLmVkdQ==
†ORCID: Bhupinder Singh Jatana orcid.org/0000-0001-6250-8342
Disclaimer: All claims expressed in this article are solely those of the authors and do not necessarily represent those of their affiliated organizations, or those of the publisher, the editors and the reviewers. Any product that may be evaluated in this article or claim that may be made by its manufacturer is not guaranteed or endorsed by the publisher.
Research integrity at Frontiers
Learn more about the work of our research integrity team to safeguard the quality of each article we publish.