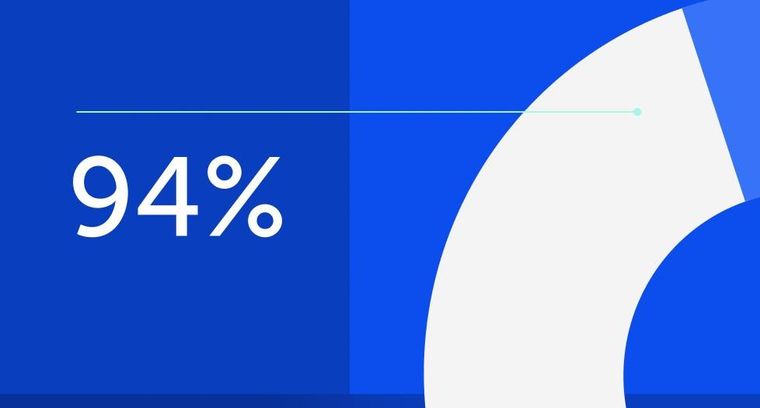
94% of researchers rate our articles as excellent or good
Learn more about the work of our research integrity team to safeguard the quality of each article we publish.
Find out more
REVIEW article
Front. Sens., 03 February 2023
Sec. Biosensors
Volume 4 - 2023 | https://doi.org/10.3389/fsens.2023.1015403
This article is part of the Research TopicHealth monitoring and intervention systems for space missionView all 4 articles
Human presence in space has uncovered several health concerns related to the space environment that need to be addressed for future space missions. The hostile space environment includes radiation and microgravity that cause various pathophysiological effects. Among them are conditions related to the cardiovascular system. The cardiovascular system shows a dysfunctional and deconditioning state, similar to ageing on Earth, once exposed to the space environment. As we aim for longer space missions to the Moon, Mars, and thus into deep space, better understanding, monitoring, and development of countermeasures for these accelerated ageing processes are necessary. Biomarkers and their integration into biosensors therefore become important tools to understand the underlying mechanisms, develop countermeasures and monitor accelerated cardiovascular ageing. In this review, we will provide a brief overview of the space environment and its effects on the human cardiovascular system. We list the known potential cardiovascular ageing biomarkers relevant to space along with our current knowledge of the underlying mechanisms of cardiovascular ageing. We also explore in more details about the various biosensors used, their specifications, and how lab-on-a-chip systems are crucial to the development of these biosensors for tracking cardiovascular ageing during upcoming space missions.
Exploration of our Solar System and beyond has been pursued by humanity for more than 60 years. Numerous societal benefits have been developed over the past decades from the research conducted to enable space exploration, improving our life here on Earth. Solar panels; meteorological forecasting; satellite communications; energy storage; recycling and waste management; advanced robotics; as well as distant medical intervention and monitoring are just a few examples of how space exploration has enhanced our everyday lives (ISECG, 2013; UNOOSA, 2022). Space exploration will continue to be an important driver for opening up new domains in science and technology, providing immediate benefits to Earth. Although the space environment enables innovations, it is also harmful to humans. One example of a health hazard encountered by astronauts is the ageing-like effects of the cardiovascular system during long-duration spaceflights (Baran et al., 2022). The presence of microgravity and ionizing radiation in space causes inflammation, DNA damage, as well as remodeling of the human heart and circulatory system (Hughson et al., 2018; Baran et al., 2022). In future and longer space missions, the impact of these factors will be important to consider. The use of biomarkers and biosensors that allow pre-screening of individual susceptibility preflight and monitoring of health effects during inflight missions will be crucial for successful space missions (Roda et al., 2018). It is therefore of importance to develop biosensors for monitoring cardiovascular ageing before, during, and after these missions to protect astronauts and gain a better understanding of the ageing effects (ISECG, 2013; Thales Group, 2020).
On Earth, population ageing is one of the most important demographic and social shifts observed in the last decade. Age is a major risk factor for cardiovascular diseases, which are Europe’s leading cause of death (17.9 million deaths, or 32 percent of all deaths) (WHO, 2022). Ageing is a complex process of slowly accumulating cellular and molecular events. Generally ageing is characterized by the nine hallmarks of ageing including, genomic instability, telomere attrition, epigenetic alterations, loss of proteostasis, dysregulated nutrient-sensing, mitochondrial dysfunction, cellular senescence, altered intercellular communication and stem cell exhaustion (López-Otín et al., 2013). Each hallmark is associated with several intrinsic and extrinsic factors and progression through each of these hallmarks contribute to the ageing process. Leading to decreased cellular fitness and eventually organ deconditioning and disease (López-Otín et al., 2013; Gude et al., 2018). Hence, biosensors for monitoring of cardiovascular ageing would both be a benefit for Earth and space exploration.
In this review, we briefly introduce the space environment and its effects on the human cardiovascular system. We summarize our current understanding of the underlying mechanisms of cardiovascular aging and the known potential biomarkers of cardiovascular ageing relevant to space. We further discuss the use of different biosensors, their requirements, and how lab-on-a-chip systems are playing a major role in the development of these biosensors for monitoring cardiovascular ageing during future space missions.
The term “space environment” refers to the expansive emptiness that permeates the (relatively) barren regions of the cosmos that lie beyond the atmosphere and surface of any planet or other celestial body (Horneck et al., 2015). It is a vacuum where objects encounter different radiation types and qualities as well as gravitational fields. Gravity levels and radiation doses encountered in space around Earth are indicated in Figure 1
FIGURE 1. Encountered levels of microgravity and radiation in space as well as the five major hazards of human spaceflight. Reproduced from Moroni et al. (2022) with permission from Elseviere.
Due to the mass of the Earth, objects experience a gravitational force pulling them towards the center of the Earth. In low earth orbit (200–2,000 km altitude) (Gheorghe and Yuchnovicz, 2015), such as on the international space station (ISS; 400 km average altitude), astronauts are still subjected to approximately 90% of the gravity force felt on the surface of the Earth (1 g) (Bambi, 2018). However, due to the orbital position and the speed of the spacecraft where the astronauts reside, this residual gravity force is counterbalanced, making the astronauts experience microgravity. The study of how cells are influenced by their physical environment is known as mechanobiology. A process in mechanobiology called mechanotransduction is the conversion of mechanical signals into biochemical signals that trigger subsequent signaling cascades. As humans have adapted and evolved to the gravity level found on Earth, the cells and tissue homeostasis are also dependent on that gravity. Hence, changes in gravity levels (microgravity, 0.17 g on the Moon or 0.376 g on Mars) will affect mechanotransduction which influences cellular function and dysfunction (Demontis et al., 2017; Bradbury et al., 2020). An example of microgravity’s impact on the cardiovascular system is the fluid redistribution towards the upper body and head, leading to cardiovascular deconditioning over time (Demontis et al., 2017), similar to accelerated ageing (Gallo et al., 2020).
Space radiation contains ionizing radiation in the form of protons, highly charged energy (HZE) nuclei, and electromagnetic radiation. Forming a complex spectrum of both high- and low-linear energy transfer (LET) radiation (Cucinotta et al., 2010; Baran et al., 2022). On Earth, life is protected from harmful ionizing space radiation through particle deflection due to the Earth’s magnetosphere and shielding by the atmosphere. The ISS is still under the protection of the geomagnetic field but is no longer shielded by the atmosphere. Therefore, the average dose rate measured inside the ISS is considerably higher than on Earth but still smaller than 1 mSv/day. The resulting biological effects are thus small, even for longer-term missions (Durante and Kronenberg, 2005). Beyond low earth orbit (LEO), space radiation is characterized by a constant, isotropic flow of galactic cosmic rays (GCR) and occasionally high dose rate of solar particle events (SPEs) (Boerma et al., 2015).
GCR is mainly composed of hydrogen (H; 87%), helium (He; 12%), and small fractions of neon (Ne), silicon (Si), calcium (Ca), and iron (Fe) (Durante and Kronenberg, 2005). Ne, Si, Ca, and Fe manifest as HZE nuclei that move at relativistic speed. He and HZE nuclei are high-LET radiation types with energies ranging from 10 MeV/n to 50 GeV/n (Norbury et al., 2016; Patel, 2020). The average nuclei can penetrate tens to hundreds of centimeters of water or aluminum and thus cannot be stopped by any reasonable shielding of a typical spacecraft (Zeitlin et al., 2013). Two different types of GCR can be distinguished: primary and secondary ions. When a primary GCR ion hits a target nucleus, a nuclear interaction causes a lighter secondary product to lose energy as well. This makes the secondary product able to penetrate even deeper into the material. In deep space, particle fluxes or tissue doses are between 0.3 and 0.6 mGy/day or 1 and 1.8 mSv/day (Norbury et al., 2016). The current recommendation for occupational exposure is limited to 20 mSv/year (Vetter, 2008). Thus, GCR forms a major health threat to future human exploration missions that go beyond LEO as GCR accounts for a significant portion of the radiation dose accumulated by astronauts (Chancellor et al., 2014).
SPEs occur when protons emitted by the Sun get accelerated due to a solar flare or coronal mass ejection shock. These events are responsible for unpredictable, large plasma clouds of heavy ions and highly energetic protons that cause sudden bursts of radiation in and outside of a spacecraft (Chancellor et al., 2014; Patel, 2020). Besides protons, SPEs can contain smaller fractions of He nuclei and HZE particles. The energies of the flow of protons can extend to a few hundred 100 MeV with speeds between 450 and 700 km/s (Miroshnichenko, 2015). However, the energy and speed varies considerably from event to event. Particle fluxes can fluctuate between 0–100 mGy/h and 0–0.5 Sv/h over hours to a few days (Boerma et al., 2015; Norbury et al., 2016). These particles have, compared to GCR, lower energies and LETs, allowing shielding to be more efficient against SPEs than GCR (Zeitlin et al., 2013). Although rare, five SPEs were recorded by the Mars Science Laboratory on its 253-day mission to Mars (Zeitlin et al., 2013). These findings raise concerns about human-rated missions to Mars and the permanent establishment on the Moon.
Furthermore, a spacecraft can also experience radiation from the Van Allen Belts when passing through them. The Van Allen belts consist of an inner and an outer belt. The inner belt (altitude of 1,000 to 9,650 km) is composed of high-energy protons and electrons produced by the Sun. The outer belt (altitude of 13,000 to 60,000 km) contains particles originating from cosmic rays that are trapped in those belt by the geomagnetic field (Li and Hudson, 2019).
The National Aeronautics and Space Administration (NASA) and the European Space Agency (ESA) have identified four of the most serious biomedical risks that humans face when exposed to space radiation. These main risks include degenerative tissue effects (including cardiovascular diseases), carcinogenesis, hereditary effects, and acute radiation syndrome (Chancellor et al., 2014; Nelson, 2016; Patel, 2020).
As outer space is difficult and very costly to reach, ground-based analogs are crucial for space research. Several ways to simulate microgravity on Earth are available, such as bed rest, dry immersion, drop towers, parabolic flights, clinostats, and random positioning machines (RPM) (Baran et al., 2022). Bed rest allows for prolonged exposure to reduced gravity on a human body through weight unloading and fluid shift using a head-down incline of the bed (Pavy-Le Traon et al., 2007; Lipnicki and Gunga, 2009; Tomilovskaya et al., 2019). During dry immersion, participants are immersed in water while avoiding wetting by using plastic sheets. These studies typically range from 5 to 56 days. Through weight unloading, this type of study can quickly and accurately induce most of the physiological effects experienced by astronauts during short-term flights (Neufeld and Charles, 2015; Tomilovskaya et al., 2019). However, bed rest and dry immersion do not exactly simulate microgravity as the human body merely experiences a type of “weight unloading.” A way to truly simulate microgravity is by using drop towers or parabolic flights as a spaceflight analog. The drop tower at the Center of Applied Space Technology and Microgravity in Bremen can offer a free fall period of 5 s from its 110 m height (Selig et al., 2010). Parabolic flights can obtain slightly longer periods of microgravity ranging from 20 to 25 s. Here, an aircraft flies repeated parabolic trajectories resulting in short-duration periods of hypergravity phases (1.8 g) and free fall phases (0 g) (Shelhamer, 2016). For relatively slow-responsive living objects, such as plants, microorganisms or cells, a longer periods of microgravity is possible which is provided with clinostats and the RPM. The RPM produces a continuous random change in the orientation. In this way, the average gravity vector experienced by the organism, over time, can reach 0.001 g (Borst and van Loon, 2009). Similarly, 2D and 3D clinostats work on the same principle, but they rotate in one or two axes (Russomano et al., 2005).
As the doses of radiation in LEO are very small (≤1 mSv/day), the biological effects due to space radiation on space laboratories such as the Space Shuttle and the ISS are small and even below the detection threshold for some experiments (Durante and Kronenberg, 2005). For this reason, most of the current knowledge about the biological effects of space radiation is based on experiments performed at ground-based accelerators. Such accelerators are capable of generating particle beams of protons, ions, neutrons, and photons (Hidding et al., 2011). With ground-based accelerators, it is possible to produce high-energy (<2 GeV/n), low-energy (>15 MeV/n) ions, and X-ray (<6 Gy/min) radiation (Durante et al., 2010). Other devices able to generate low-LET radiation such as gamma or X-rays further play a vital role in simulating space radiation in biological experiments (Delp et al., 2016; Norbury et al., 2016).
Although the impact of microgravity and ionizing radiation on living objects has been extensively studied in the past decades, simultaneous exposure to both, and especially chronic low dose exposure, is limited. We previously described such an experimental set-up using a 252Cf neutron source and a RPM (Pani et al., 2016), a concept further developed by Takahashi et al. (2020). Through the use of the hindlimb model as an analog for microgravity (Globus and Morey-Holton, 2016), simultaneous exposure of rodents to microgravity and radiation is also emerging (Bellone et al., 2016; Sadhukhan et al., 2021).
Ageing is a natural and multi-factorial process that includes the progressive deterioration of almost every organ system inside the human body over time (Sprott, 2010; Wagner et al., 2016). Therefore, ageing lies at the bottom of nearly every non-communicable disease, such as cancer, diabetes, neurological diseases, and cardiovascular diseases, the latter being the leading cause of disease burden in the world (Sprott, 2010; Wagner et al., 2016).
Cardiovascular diseases are the main causes of death in the industrialized world. In the elderly population, the prevalence of cardiovascular diseases and the rate of cardiovascular mortality rises exponentially. This suggests that age is a key risk factor for cardiovascular diseases (Dai et al., 2012a). Moreover, the worldwide decline in fertility is commonly believed to increase the life span due to energetic and metabolic costs during reproduction in combination with reproductive genes increasing mortality later in life (Kuningas et al., 2011). Together with an increase in longevity coupled to an improvement of diagnostic and therapeutic procedures, the world population is getting older (Steenman and Lande, 2017). Consequently, the worldwide prevalence of cardiovascular diseases is increasing (Lakatta, 2003). Cardiovascular ageing is defined as a slow, gradual structural change and functional decline of the heart with age (Dai et al., 2012b; Yan et al., 2021), brought on by both extrinsic (environmental and way of life) and intrinsic (e.g., genetic) factors (López-Otín et al., 2013). Since there is a clear link between age and cardiovascular diseases, a better insight into the biological mechanisms and processes behind cardiovascular ageing may help prevent and treat age-related cardiovascular diseases. In this way, it can help in extending the lifespan and lowering the increasing burden due to cardiovascular diseases experienced by the elderly population (Steenman and Lande, 2017).
Cardiovascular ageing seems to be specific to biological sexes (Keller and Howlett, 2016; Rodgers et al., 2019). For example, biological sex disparities in ageing are already evident in life expectancy, where women have on average an additional 4.8 years compared to men. Indeed, men and women have different physiological systems that regulate vascular function and sex hormones are responsible for the most pronounced endocrine alterations associated with ageing. It is well known that men display an increased amount of adverse cardiovascular events compared to pre-menopausal women. However, this statistic inverts after menopause due to the fall of protective female sex hormones such as estrogen (Dela Justina et al., 2021). Despite this observation, the mechanisms of biological sex-specific cardiovascular ageing remain unclear.
Intrinsic processes of cardiovascular ageing, inducing loss of homeostasis, are driven by chronic inflammation and oxidative stress, which exacerbate telomere shortening, epigenetic drift, senescence, mitochondrial dysfunction, stem cell exhaustion, and genomic instability (Gude et al., 2018) (Figure 2).
FIGURE 2. Interaction between the main cellular and molecular changes that causes cardiovascular ageing. Created with BioRender. Abbreviations: ROS, Reactive Oxygen Species; SASPs, Senescence Associated Secretory Phenotype; DAMPs, Damage-Associated Molecular Patterns.
Chronic inflammation, which typically manifests itself in a low-grade manner for a prolonged period, is associated with dysregulated pro-inflammatory mediators, cytokines, and chemokines, as well as changes in the cellular redox state and cell death signaling pathways (Chung et al., 2006). While the immune system is a key component of the inflammation process (Ferrucci and Fabbri, 2018), sterile inflammation also has an important role. The term “sterile inflammation” refers to inflammation that occurs in the absence of microorganisms and results from physically or chemically produced cellular injuries but can also originate from autoimmune diseases and exposure to allergens (Rock et al., 2010). Hence, sterile inflammation occurs when damage-associated molecular patterns (DAMPs) are released into the extracellular environment as a consequence of cell death (apoptosis or necrosis) (Chen and Nuñez, 2010).
Oxidative stress is defined as the increased production of reactive oxygen species (ROS) relative to the bioavailability of antioxidant defenses (Donato et al., 2015; Wray et al., 2017). These ROS have strong oxidizing traits, are highly unstable, and can easily react with other molecules within a few microseconds (Schmitt et al., 2014). Therefore, ROS causes damage to cellular components and the accumulation of damage is thought to be the main process that drives ageing (Dai et al., 2012a). Cellular antioxidant systems that counteract ROS include superoxide dismutase (SOD), catalase, and glutathione peroxidase. However, these systems are very complex, and genetic manipulation of these system components has resulted in many inconsistent results (Dai et al., 2012a). Still, through overexpression of catalase in the nucleus, peroxisome, or mitochondria of transgenic mice, it was seen that mice overexpressing mitochondrial catalase had a prolonged lifespan compared to control mice. As ROS are mainly produced in mitochondria, mitochondrial dysfunction as a result of free radical damage plays a central role in ageing (Schriner et al., 2005). Although low concentrations of ROS may be beneficial for cells by stimulating cell defense mechanisms to decrease cell damage, high levels of ROS lead to serious cell injury and inflammatory responses (Yun and Finkel, 2014). The heart is known to have a higher basal oxygen consumption and generate more ROS compared to other tissues (Goffart et al., 2004). Therefore, oxidative stress plays a crucial role in cardiovascular ageing (Wray et al., 2017).
Production of free radicals is a common source of oxidative stress. Production of free radicals leads to lipid peroxidation, generating a complex mix containing many electrophilic side products (Marnett, 1999). One of these products is malondialdehyde (MDA), which has commonly been used as a biomarker of lipid peroxidation and in extension, ageing (Jové et al., 2020). MDA has been shown to be genotoxic and mutagenic and further shown increased levels in patients with age related diseases (Mohanty et al., 2022), including cardiovascular ageing (Isola et al., 2019). MDA production has been shown to increase in hearts of mice experiencing myocardial dysfunction due to tail suspension (Liang et al., 2019; Liang et al., 2020) as well as in blood serum of rats experiencing radiation induced damage to the myocardium (Kura et al., 2019). Cellular senescence has also been associated with ageing in general, including cardiovascular ageing (Campisi, 2013). The function of cellular senescence is to induce tissue remodeling to remove unwanted cells. This is typically achieved through first, proliferative arrest followed by recruitment of immune cells through secretion of senescence associated secretory phenotype (SASP). Sequentially, progenitor cells mobilize and remodel the tissue (Muñoz-Espín and Serrano, 2014). Cellular senescence is therefore a normal and necessary defense mechanism after tissue damage to combat disease. However, during late stage disease senescent cells are not efficiently cleared, leading to a buildup of unwanted less functional cells over time, promoting disease (Campisi, 2013; Muñoz-Espín and Serrano, 2014). Cellular senescence can be induced by various factors. Among the most common ones are DNA damage (Zglinicki et al., 2005), telomere shortening (Bernadotte et al., 2016), CDKN2A expression (Krishnamurthy et al., 2004), reactive oxygen species induced stress (Chen et al., 1995), oncogene activation (Serrano et al., 1997) and as previously mentioned SASP secretion (Kuilman and Peeper, 2009). Cellular senescence is often associated with the misfolding of proteins and dysfunction of cellular organelles, leading to oxidative stress, cell death, and inflammation (Muñoz-Espín and Serrano, 2014). As mentioned above, mitochondrial dysfunction is one of the common causes and pathological features of cardiovascular ageing. The mitochondrion is the major site of aerobic respiration and the production of energy in the form of ATP (van der Bliek et al., 2017). Both endogenous and external stimuli can cause mitochondria to get damaged or mtDNA to become mutated. Because mitochondria are a major source of intracellular ROS and the SASP, mitochondrial dysfunction leads to an increase in ROS production (Schriner et al., 2005; Correia-Melo et al., 2016). Consequently, this results in increased levels of oxidative stress. Further damaging more mitochondria, leading to a vicious circle driving cardiac cell senescence (Muñoz-Espín and Serrano, 2014).
In addition, another molecular mechanism of cardiovascular ageing is impaired autophagy. Autophagy is known as a biological process responsible for the degradation and recycling of damaged organelles and misfolded proteins. It aides cell survival by removing damaged cell components. Excessive levels of autophagy can, on the other hand, promote cell death (Shirakabe et al., 2016). Cardiovascular ageing is associated with an impaired level of autophagy. Therefore, an ageing heart is characterized by an accumulation of misfolded proteins and mitochondrial dysfunction (Taneike et al., 2010).
As mentioned above, telomere shortening is a known biomarker of ageing. Telomeres are positioned at the ends of chromosomes in eukaryotic cells and consist of short DNA repeats. This forms a special cap that provides genome stability and protection from degradation (Blackburn, 1991). Telomeres shorten when cells divide, eventually leading to permanent DNA damage and cellular senescence, consequently contributing to ageing (Zhan and Hägg, 2019). However, cardiomyocytes are known to be postmitotic, meaning they have little ability to divide during the lifespan of the organism, suggesting that telomere shortening does not contribute vastly to cardiomyocyte ageing (Woodcock and Matkovich, 2005; Baran et al., 2022). Nevertheless, it was found that oxidative stress causes single-strand breaks in telomeres and, furthermore, accumulates with age and contributes to cardiovascular ageing (von Zglinicki et al., 1995).
Finally, genomic instability comprises three main alterations resulting from: 1) DNA damage; 2) mutations; and 3) epigenetic changes, which modify gene activity without affecting DNA sequence (Paneni et al., 2017). Hence, changes in gene expression (Herman et al., 2021) as well as non-coding RNAs have been linked to causes of cardiovascular ageing (Jusic et al., 2022).
Ageing and spaceflight affect human physiology in a similar way. Ageing and prolonged exposure to microgravity cause a decline in functionality of almost every human organ system, including cardio-vascular deconditioning. Suggesting that astronauts, when in spaceflight, age quicker than on Earth (Nicogossian et al., 2016; Hughson et al., 2018). For example, 6 months of spaceflight increased the carotid arterial stiffness in astronauts, consistent with one or two decades of ageing observed on Earth (Patel, 2020). However, astronauts rapidly recovered from those symptoms after returning to Earth (Nicogossian et al., 2016). Though, the rate of the observed decline in functionality and recovery was highly individual and depended to a large extent on genetics, lifestyle, gender and metabolism (Vernikos and Schneider, 2010).
Exposure to space radiation is a well-known factor that increases the chances of developing cancer or even acute radiation illness when passing beyond the Earth’s magnetosphere (Nicogossian et al., 2016). The 24 lunar Apollo astronauts are the only humans that have traveled beyond Earth’s protective magnetosphere. Their health inspection revealed increased mortality due to cardiovascular disease (43%), compared to astronauts that resided in LEO (11%) or humans that never took part in any spaceflight (9%) (Delp et al., 2016). The increased mortality is potentially due to a higher exposure to GCR, radiation from which astronauts in LEO are protected by the Earth’s magnetosphere (Patel, 2020). To investigate the underlying mechanisms, mice were exposed to simulated microgravity and radiation. The results indicated that exposure to HZE particles (and not weightlessness) caused sustained vascular endothelial cell dysfunction, likely as a result of the genetic changes and perturbations to the redox metabolism caused by the HZE nuclei, which lead to an increased level of oxidative stress (Delp et al., 2016; Patel, 2020). Again, increased oxidative stress levels have been demonstrated to stimulate degenerative tissue changes, generally associated with ageing symptoms such as cataract formation and cardiovascular disease (Chancellor et al., 2014). Furthermore, it raises awareness of the importance of performing long-term studies concerning the effect of radiation on the cardiovascular system. Especially when considering that the most detrimental effects on the heart and blood vessels are only observed years or even decades after exposure to ionizing radiation (Boerma et al., 2015). A detailed review of how the cardiovascular system is impacted by space is described by Baran et al. (2022).
A biomarker of ageing is defined as a biological parameter that, alone or as part of a multivariate panel, can monitor an underlying age-related biological process rather than the development of a disease. Biomarkers of ageing also have the ability to predict ageing rates better than the chronological age of an organism and the capacity to test the presence of the biomarker repeatedly without affecting the organism (Sprott, 2010; Justice et al., 2018). In this way, disease-free subjects can be tested for single parameters in a reasonable period (less than the lifespan of that organism). However, the quest for biomarkers of ageing is not always easy as they need to be separated from biomarkers of disease. Furthermore, as ageing affects almost every body system, a single biomarker that can predict the physiological age of an organism will presumably not be found. It is more likely that a panel of biomarkers will be needed (Sprott, 2010). Wagner et al. reviewed a panel of possible biomarkers, including ones related to physical function such as Body Mass Index and standing balance of the person as well as blood-based markers such as interleukin-6 and thyroid hormones, and molecular or DNA-based markers including telomere length or DNA repair (Wagner et al., 2016). Furthermore, Justice et al. investigated 258 blood-based candidate biomarkers for ageing to use in an experiment to target ageing with metformin. Some of the suggested biomarkers included IL-6, TNF-α receptor I or II, GDF15, and CRP (Justice et al., 2018). On the other hand, senescent cells secrete cell-type specific molecules (SASPs). For instance, senescent fibroblasts secrete epithelial growth factors, inflammatory cytokines and several matrix metalloproteinases (Krtolica and Campisi, 2002). While cardiomyocytes and endothelial cells are also known to secrete some cell-specific inflammatory cytokines (Bochenek et al., 2016; Tang et al., 2020; Mehdizadeh et al., 2022). A list of potential biomarkers can be found in Table 1.
A biosensor is defined as a self-contained integrated device which is capable of providing specific quantitative or semi-quantitative analytical information using a biological recognition element (biochemical receptor) which is retained in direct spatial contact with an electrochemical transduction element (Thevenot et al., 1999). Therefore, the two main components of a biosensor are a biochemical recognition system and a physicochemical transducer. The biochemical recognition system translates input from the biochemical domain (e.g., ionophores, enzymes, antibodies and cytokines, amongst others) into a chemical or physical output signal. The transducer transforms the information from the biochemical receptor into a signal that is proportional to the amount of analyte present in the sample. The different types of transducers are based on electrochemical principles (amperometric, potentiometric, or conductometric), optical principles (absorbance, reflectance, fluorescence), mass-sensitivity (piezo-electric), or thermal principles (calorimetric, pyroelectric) (Thevenot et al., 1999) (Figure 3). Biosensors are an important diagnostic tool already in use nowadays (e.g., pregnancy tests, rapid antigen tests for COVID-19) and have received increased interest for non-invasive wireless point of care applications (Arumugam et al., 2020; Heidt et al., 2020).
FIGURE 3. Components of a biosensor - Typically, biosensor is made up of a biological component (a biochemical receptor like an enzyme, antibody, cell, nucleic acid, or aptamer), a transducer (like a semi-conducting material or nanomaterial), which is a physical part that takes the biochemical signal from the detector, amplifies it, changes it into an electrical signal, and shows it in a way that people can understand, and an electronic system with a signal amplifier, processor, and display. Created with BioRender.
Early detection of age-related diseases for early treatment, in particular related to cardiovascular ageing, has received rising interest due to the population ageing shift that is currently observed worldwide. Present biosensors of cardiovascular ageing focus on non-invasive procedures that uses physical function (such as blood pressure, body mass index, and electrocardiogram analysis) (De Cannière et al., 2020; Zhu et al., 2022) or physiological fluids (mostly blood-based) (Falk et al., 2020; Ouyang et al., 2021) that are coupled to a machine learning algorithm for predictive cardiovascular risk assessment (Arpaia et al., 2021). Blood-based biomarkers for biosensors of cardiovascular diseases that are currently available have recently been extensively reviewed by Ouyang et al. (2021) and are therefore not further elaborated here. Although the described biosensors are promising and allow partial monitoring of cardiovascular diseases in patients, there is still a need for novel biomarkers and sensor technology for sensitive, specific, easy-to-use, real-time monitoring and affordable biosensors (Wagner et al., 2016). Furthermore, biosensors that are specific to the induction of cardiovascular ageing due to exposure to microgravity and/or radiation have not yet been extensively developed and warrant further research (Kanapskyte et al., 2021). In Table 1, a summary of potential biomarkers for space-induced cardiovascular ageing can be found, together with available detection methods and biological induction by ionizing radiation and microgravity.
Lab-on-a-chip systems are integrated micro-electro-mechanical systems used to miniaturize biological and chemical processes. Lab-on-a-chip platforms typically use microfluidic networks to enable miniaturization and integration of complex functions that allow for automation and replacement of bulky lab equipment (Abgrall and Gué, 2007). Furthermore, the use of small volumes in microfluidics allows for reduced sample consumption and shortened transport times, leading to faster reaction times. Moreover, the physically smaller footprint of the device simplifies point-of-care applications and enables field applications. Microfluidic networks are composed of channels in the range of a few micrometers to a few hundred micrometers (Abgrall and Gué, 2007). Commonly, these devices are made of a combination of glass, polymers, and silicon. Their fabrication uses cleanroom facilities and microchip fabrication techniques. As a result, complex micro- and nano-scale structures can be incorporated into the design. However, recently the use of more widely available techniques such as 3D printing has been used to fabricate micro-devices (Ren et al., 2013). Through integration of pumps, valves, sensors, and optics, to name a few, complex systems can be put together that can be used for numerous purposes.
Lab-on-a-chip systems have been extensively used in biomedical research for several different purposes (Dittrich and Manz, 2006; Neužil et al., 2012). Miniaturization allows for parallelization and combination of many processes while also facilitating small volume handling, proving highly useful for biological processes. Therefore, lab-on-a-chip systems were originally used for applications like electrophoresis, where decreased sample volume was desirable (Dittrich and Manz, 2006; Neužil et al., 2012). As lab-on-a-chip systems were more frequently integrated into biomedical research, their applications expanded and are today frequently used for screening, cell sorting, cell encapsulation, and single cell analysis, among others (Gupta et al., 2016). Biosensors are one specifically well-suited application of lab-on-chips (Luka et al., 2015; Sengupta et al., 2019). For cardiac applications, immunological assays have been incorporated into microfluidic devices for the detection of biomarkers of cardiovascular disease (Mohammed and Desmulliez, 2011; Wu et al., 2017). Standard immunoassays can be automated on a lab-on-chip and have been used for the detection of several cardiac markers such as myoglobin, cardiac troponin I and CRP (Mohammed and Desmulliez, 2014; Weng et al., 2016; Wu et al., 2017). By incorporating optics into the devices, detection can be added onto the same chip. Furthermore, lab-on-a-chip systems can be utilized to further simplify standard immunoassays. Blocking free and label free immunoassays of cardiac biomarkers can be realized using lab-on-a-chip devices (Pekin et al., 2011). Another application case of lab-on-a-chip systems is based on the detection of certain cell types as biomarkers. As an example, coronary disease can be predicted from an increasing white blood cell count (Grimm et al., 1985). Using a paper-based platform, Zhang et al. (2015) quantified pre-stained white blood cells using colorimetric intensity, allowing for substantially smaller sample volumes to be used compared to standard detection methods.
Organ-on-chip systems are micro-physiological systems that can mimic organ functions and can thus be used as in vitro models of the human organ. Allowing for e.g., more accurate disease modeling and drug testing (Zhang et al., 2018). Biosensors can be integrated onto the organ-on-chip similarly as on lab-on-a-chips, allowing for real time monitoring of organ function and response (Kratz et al., 2019). Commonly integrated biosensors can measure oxygen, glucose, lactate, cytokines, trans endothelial- and epithelial resistance, electric- and mechanical activity (Kratz et al., 2019). Heart-on-chips have been used as a model of the human microenvironment of the heart, as it allows for exposure of several physiological cues (Agarwal et al., 2013; Wang et al., 2014; Marsano et al., 2016; Zhang et al., 2016; Zhao et al., 2020). Biosensors are commonly integrated into heart-on-chips to monitor the contractility or electric activity of the cardiac construct. Lai et al. (2017) used a force probe to measure the deflection of a cantilever based heart-on-chip to record the cardiac activity Likewise, Chen et al. (2019) modified polydimethylsiloxane (PDMS) cantilevers in a heart-on-chip with a color changing material that would change color upon exposure to strain Furthermore, using a hydrogel based chamber specific heart-on-chip, Zhao et al. (2020) investigated the chamber specific response to different drugs by monitoring the displacement of an anchoring electrode using fluorescence microscopy.
Exposure to the space environment causes several negative effects on the human body. Among many other organs, the heart and cardiovascular system are especially affected (Hughson et al., 2018). Exposure to chronic microgravity and space radiation causes ageing of the cardiovascular system, substantially negatively impacting human health (Baran et al., 2022). Forty years after the Apollo program, Delp et al. (2016) showed in their study that the cardiovascular disease mortality rate was 4–5 times higher among Apollo lunar astronauts than in non-flight and LEO astronauts. Astronauts showed “aged-like” deconditioning, including loss of physical fitness, arterial stiffening and development of insulin resistance. This is further strengthened by epidemiological studies, which have shown that doses of >0.5 Gy significantly increase the risk of both stroke and heart disease without the mitigation of possible adverse effects at lower doses (Hughson et al., 2018). Therefore, to keep future astronauts healthy, it will be crucial to be able to predict and monitor the effects of exposure to the space environment through biomarkers of cardiovascular ageing before, during, and after flight. Considering the continuously changing radiation environment in space, monitoring of these biomarkers during flight may prove especially important to be able to properly diagnose and protect astronauts during flight (Cucinotta et al., 2010; Boerma et al., 2015; Demontis et al., 2017). Therefore, sample collection and analysis are required to be as straightforward as possible. Many of the current available biomarkers of cardiovascular ageing are therefore not ideal candidates for in-flight monitoring, as they either require complex sample collection or difficult analysis (Durante, 2005). These could, however, be evaluated pre-flight to predict individual susceptibility more accurately. During flight, though, blood or saliva based biomarkers are of higher interest because of simple sample collection and high availability (Zuidema et al., 2022). SASPs therefore become interesting candidates because of their close association with the ageing phenotype and ease of detection. Non-invasive biomarkers, such as blood pressure, body-mass index, and electrocardiogram analysis, may also be important, as they would allow for frequent monitoring (De Cannière et al., 2020; Zhu et al., 2022). Although we listed potential biomarkers that are sensitive to microgravity and radiation, care should be taken during interpretation. These biomarkers are based on experiments conducted on Earth under conditions that partially simulate the space environment. Selection of adequate biomarkers should be therefore validated in experiments on the international space station.
Another important aspect to consider is the biosensor used for detection and analysis. Ease of use and portability will be highly prioritized characteristics of the device as point-of-care testing will be necessary on the space station and future space missions to the Moon and Mars (Roda et al., 2018). Rapid antigen tests and lab-on-a-chip systems minimize the use of equipment and facilitate automation and point-of-care testing (Gubala et al., 2012; Heidt et al., 2020). Therefore, fulfilling many of the requirements. Development of these types of platforms for space purposes will have high potential. Also, motivated by the need to sustain astronaut health, numerous biomedical and human physiological studies in space have provided significant discoveries, including a better knowledge of basic physiological processes, the creation of novel medical technologies and protocols that are now in use on Earth. At current, there is still a need for the development of novel and specific biosensors for cardiovascular ageing. Research and development of a specific and sensitive biomarker (set) and accompanying biosensor will be highly beneficial and crucial for future space missions as well as on Earth (Wagner et al., 2016). However, due to the scarcity of in-flight human samples the discovery of new biomarkers and testing of novel biosensors is hampered. Development of model systems such as human organ-on-a-chip systems may therefore help both during the discovery of biomarkers and the development of biosensors for cardiovascular ageing in space and on Earth (Zhang et al., 2018; Low and Giulianotti, 2020). Lastly, creating a model system of cardiovascular ageing in space here on Earth using ground based facilities will prove important for development and validation of such a biosensor as well as designed countermeasures.
ER and KQ wrote the original draft. BB and NR revised the interim drafts and the final version. TS, LM, and SB supervised the writing and revised the final version. SB warranted funding. KT streamlined the topic, prepared the figures and the final version of the original text. All authors contributed to the article and approved the submitted version.
This work is supported by an ESA PRODEX grant IMPULSE (PEA4000134310).
The authors declare that the research was conducted in the absence of any commercial or financial relationships that could be construed as a potential conflict of interest.
All claims expressed in this article are solely those of the authors and do not necessarily represent those of their affiliated organizations, or those of the publisher, the editors and the reviewers. Any product that may be evaluated in this article, or claim that may be made by its manufacturer, is not guaranteed or endorsed by the publisher.
Abgrall, P., and Gué, A. (2007). Lab-on-chip technologies: Making a microfluidic network and coupling it into a complete microsystem-a review. J. Micromech. Microeng. 17 (5), R15–R49. doi:10.1088/0960-1317/17/5/r01
Agarwal, A., Goss, J. A., Cho, A., McCain, M. L., and Parker, K. K. (2013). Microfluidic heart on a chip for higher throughput pharmacological studies. Lab. Chip 13 (18), 3599–3608. doi:10.1039/c3lc50350j
Aladağ, N., Asoğlu, R., Ozdemir, M., Asoğlu, E., Derin, A. R., Demir, C., et al. (2021). Oxidants and antioxidants in myocardial infarction (MI): Investigation of ischemia modified albumin, malondialdehyde, superoxide dismutase and catalase in individuals diagnosed with ST elevated myocardial infarction (STEMI) and non-STEMI (NSTEMI). J. Med. Biochem. 40 (3), 286–294.
An, Y. (2016). Sirtuin 1 evaluation with a novel immunoassay approach based on TiO2-Au label and hyperbranched polymer hybrid. Anal. Biochem. 507, 58–65. doi:10.1016/j.ab.2016.05.020
Arpaia, P., Cuocolo, R., Donnarumma, F., Esposito, A., Moccaldi, N., Natalizio, A., et al. (2021). Conceptual design of a machine learning-based wearable soft sensor for non-invasive cardiovascular risk assessment. Measurement 169, 108551. doi:10.1016/j.measurement.2020.108551
Arumugam, S., Colburn, D. A. M., and Sia, S. K. (2020). Biosensors for personal mobile health: A system architecture perspective. Adv. Mat. Technol. 5 (3), 1900720. doi:10.1002/admt.201900720
Au - Noren Hooten, N., and Au - Evans, M. K. (2017). Techniques to induce and quantify cellular senescence. JoVE 123, e55533. doi:10.3791/55533
Baran, R., Marchal, S., Garcia Campos, S., Rehnberg, E., Tabury, K., Baselet, B., et al. (2022). The cardiovascular system in space: Focus on in vivo and in vitro studies. Biomedicines 10 (1), 59.
Baselet, B., Belmans, N., Coninx, E., Lowe, D., Janssen, A., Michaux, A., et al. (2017). Functional gene analysis reveals cell cycle changes and inflammation in endothelial cells irradiated with a single X-ray dose. Front. Pharmacol. 8, 213. doi:10.3389/fphar.2017.00213
Basirun, C., Ferlazzo, M. L., Howell, N. R., Liu, G. J., Middleton, R. J., Martinac, B., et al. (2021). Microgravity × radiation: A space mechanobiology approach toward cardiovascular function and disease. Front. Cell. Dev. Biol. 9, 750775. doi:10.3389/fcell.2021.750775
Bellone, J. A., Gifford, P. S., Nishiyama, N. C., Hartman, R. E., and Mao, X. W. (2016). Long-term effects of simulated microgravity and/or chronic exposure to low-dose gamma radiation on behavior and blood-brain barrier integrity. npj Microgravity 2 (1), 16019. doi:10.1038/npjmgrav.2016.19
Bernadotte, A., Mikhelson, V. M., and Spivak, I. M. (2016). Markers of cellular senescence. Telomere shortening as a marker of cellular senescence. Aging 8 (1), 3–11. doi:10.18632/aging.100871
Bernotiene, E., Bagdonas, E., Kirdaite, G., Bernotas, P., Kalvaityte, U., Uzieliene, I., et al. (2020). Emerging technologies and platforms for the immunodetection of multiple biochemical markers in osteoarthritis research and therapy. Front. Med. (Lausanne) 7, 572977. doi:10.3389/fmed.2020.572977
Blaber, E. A., Dvorochkin, N., Torres, M. L., Yousuf, R., Burns, B. P., Globus, R. K., et al. (2014). Mechanical unloading of bone in microgravity reduces mesenchymal and hematopoietic stem cell-mediated tissue regeneration. Stem Cell. Res. 13 (2), 181–201. doi:10.1016/j.scr.2014.05.005
Blackburn, E. H. (1991). Structure and function of telomeres. Nature 350 (6319), 569–573. doi:10.1038/350569a0
Bochenek, M. L., Schütz, E., and Schäfer, K. (2016). Endothelial cell senescence and thrombosis: Ageing clots. Thrombosis Res. 147, 36–45. doi:10.1016/j.thromres.2016.09.019
Boerma, M., Nelson, G. A., Sridharan, V., Mao, X-W., Koturbash, I., and Hauer-Jensen, M. (2015). Space radiation and cardiovascular disease risk. Wjc 7 (12), 882. doi:10.4330/wjc.v7.i12.882
Borst, A., and van Loon, J. J. (2009). Technology and developments for the random positioning machine, RPM. Microgravity Sci. Technol. 21 (4), 287–292. doi:10.1007/s12217-008-9043-2
Bradbury, P., Wu, H., Choi, J. U., Rowan, A. E., Zhang, H., Poole, K., et al. (2020). Modeling the impact of microgravity at the cellular level: Implications for human disease. Front. Cell. Dev. Biol. 8, 96. doi:10.3389/fcell.2020.00096
Campisi, J. (2013). Aging, cellular senescence, and cancer. Annu. Rev. Physiol. 75, 685–705. doi:10.1146/annurev-physiol-030212-183653
Cazzaniga, A., Locatelli, L., Castiglioni, S., and Maier, J. A. M. (2019). The dynamic adaptation of primary human endothelial cells to simulated microgravity. FASEB J. 33 (5), 5957–5966. doi:10.1096/fj.201801586rr
Cesari, M., Pahor, M., and Incalzi, R. A. (2010). Review: Plasminogen activator inhibitor-1 (PAI-1): A key factor linking fibrinolysis and age-related subclinical and clinical conditions. Cardiovasc. Ther. 28 (5), e72–e91. doi:10.1111/j.1755-5922.2010.00171.x
Chancellor, J. C., Scott, G. B., and Sutton, J. P. (2014). Space radiation: The number one risk to astronaut health beyond low Earth orbit. Life 4 (3), 491–510. doi:10.3390/life4030491
Chen, G. Y., and Nuñez, G. (2010). Sterile inflammation: Sensing and reacting to damage. Nat. Rev. Immunol. 10 (12), 826–837. doi:10.1038/nri2873
Chen, Q., Fischer, A., Reagan, J. D., Yan, L. J., and Ames, B. N. (1995). Oxidative DNA damage and senescence of human diploid fibroblast cells. Proc. Natl. Acad. Sci. U.S.A. 92 (10), 4337–4341. doi:10.1073/pnas.92.10.4337
Chen, Z., Fu, F., Yu, Y., Wang, H., Shang, Y., and Zhao, Y. (2019). Cardiomyocytes-actuated morpho butterfly wings. Adv. Mat. 31 (8), 1805431. doi:10.1002/adma.201805431
Christodoulides, N., Dharshan, P., Wong, J., Floriano, P. N., Neikirk, D., and McDevitt, J. T. (2007). A microchip-based assay for interleukin-6. Methods Mol. Biol. Clift. NJ) 385, 131–144. doi:10.1007/978-1-59745-426-1_10
Chung, H. Y., Sung, B., Jung, K. J., Zou, Y., and Yu, B. P. (2006). The molecular inflammatory process in aging. Antioxid. Redox Signal 8 (3-4), 572–581. doi:10.1089/ars.2006.8.572
Correia-Melo, C., Marques, F. D., Anderson, R., Hewitt, G., Hewitt, R., Cole, J., et al. (2016). Mitochondria are required for pro-ageing features of the senescent phenotype. EMBO J. 35 (7), 724–742. doi:10.15252/embj.201592862
Cronstein, B. N. (2007). Interleukin-6--a key mediator of systemic and local symptoms in rheumatoid arthritis. Bull. NYU Hosp. Jt. Dis. 65 (1), S11–S15.
Cucinotta, F. A., Hu, S., Schwadron, N. A., Kozarev, K., Townsend, L. W., and Kim, M. H. Y. (2010). Space radiation risk limits and Earth-Moon-Mars environmental models. Space weather. 8 (12), 572. doi:10.1029/2010sw000572
Dai, D-F., Rabinovitch, P. S., and Ungvari, Z. (2012). Mitochondria and cardiovascular aging. Circ. Res. 110 (8), 1109–1124. doi:10.1161/circresaha.111.246140
Dai, D. F., Chen, T., Johnson, S. C., Szeto, H., and Rabinovitch, P. S. (2012). Cardiac aging: From molecular mechanisms to significance in human health and disease. Antioxidants Redox Signal. 16 (12), 1492–1526. doi:10.1089/ars.2011.4179
D’Amore, P. A., and Sakurai, M. K. (2006). “ANGIOGENESIS, angiogenic growth factors and development factors,” in Encyclopedia of respiratory medicine. Editors G. J. Laurent,, and S. D. Shapiro (Oxford: Academic Press), 110–115.
De Cannière, H., Smeets, C. J. P., Schoutteten, M., Varon, C., Van Hoof, C., Van Huffel, S., et al. (2020). Using biosensors and digital biomarkers to assess response to cardiac rehabilitation: Observational study. J. Med. Internet Res. 22 (5), e17326. doi:10.2196/17326
de Mera-Rodríguez, J. A., Álvarez-Hernán, G., Gañán, Y., Martín-Partido, G., Rodríguez-León, J., and Francisco-Morcillo, J. (2021). Is senescence-associated β-galactosidase a reliable in vivo marker of cellular senescence during embryonic development? Front. Cell. Dev. Biol. 9. doi:10.3389/fcell.2021.623175
de Souza, R. R. (2002). Aging of myocardial collagen. Biogerontology 3 (6), 325–335. doi:10.1023/a:1021312027486
Debacq-Chainiaux, F., Erusalimsky, J. D., Campisi, J., and Toussaint, O. (2009). Protocols to detect senescence-associated beta-galactosidase (SA-βgal) activity, a biomarker of senescent cells in culture and in vivo. Nat. Protoc. 4 (12), 1798–1806. doi:10.1038/nprot.2009.191
Dela Justina, V., Miguez, J. S. G., Priviero, F., Sullivan, J. C., Giachini, F. R., and Webb, R. C. (2021). Sex differences in molecular mechanisms of cardiovascular aging. Front. Aging 2, 725884. doi:10.3389/fragi.2021.725884
Delp, M. D., Charvat, J. M., Limoli, C. L., Globus, R. K., and Ghosh, P. (2016). Apollo lunar astronauts show higher cardiovascular disease mortality: Possible deep space radiation effects on the vascular endothelium. Sci. Rep. 6 (1), 29901. doi:10.1038/srep29901
Demontis, G. C., Germani, M. M., Caiani, E. G., Barravecchia, I., Passino, C., and Angeloni, D. (2017). Human pathophysiological adaptations to the space environment. Front. Physiol. 8, 547. doi:10.3389/fphys.2017.00547
Dittrich, A., Grimm, D., Sahana, J., Bauer, J., Krüger, M., Infanger, M., et al. (2018). Key proteins involved in spheroid formation and angiogenesis in endothelial cells after long-term exposure to simulated microgravity. Cell. Physiol. Biochem. 45 (2), 429–445. doi:10.1159/000486920
Dittrich, P. S., and Manz, A. (2006). Lab-on-a-chip: Microfluidics in drug discovery. Nat. Rev. Drug Discov. 5 (3), 210–218. doi:10.1038/nrd1985
Donato, A. J., Morgan, R. G., Walker, A. E., and Lesniewski, L. A. (2015). Cellular and molecular biology of aging endothelial cells. J. Mol. Cell. Cardiol. 89, 122–135. doi:10.1016/j.yjmcc.2015.01.021
Durante, M. (2005). Biomarkers of space radiation risk. Radiat. Res. 164 (4), 467–473. doi:10.1667/rr3359.1
Durante, M., and Kronenberg, A. (2005). Ground-based research with heavy ions for space radiation protection. Adv. Space Res. 35 (2), 180–184. doi:10.1016/j.asr.2004.12.034
Durante, M., Reitz, G., and Angerer, O. (2010). Space radiation research in Europe: Flight experiments and ground-based studies. Radiat. Environ. Biophys. 49, 295–302. doi:10.1007/s00411-010-0300-6
Eletxigerra, U., Martinez-Perdiguero, J., and Merino, S. (2015). Disposable microfluidic immuno-biochip for rapid electrochemical detection of tumor necrosis factor alpha biomarker. Sensors Actuators B Chem. 221, 1406–1411. doi:10.1016/j.snb.2015.08.026
Emmerson, P. J., Duffin, K. L., Chintharlapalli, S., and Wu, X. (2018). GDF15 and growth control. Front. Physiol. 9, 1712. doi:10.3389/fphys.2018.01712
Falk, M., Psotta, C., Cirovic, S., and Shleev, S. (2020). Non-invasive electrochemical biosensors operating in human physiological fluids. Sensors 20 (21), 6352. doi:10.3390/s20216352
Ferrara, N. (2009). Vascular endothelial growth factor. Atvb 29 (6), 789–791. doi:10.1161/atvbaha.108.179663
Ferrucci, L., and Fabbri, E. (2018). Inflammageing: Chronic inflammation in ageing, cardiovascular disease, and frailty. Nat. Rev. Cardiol. 15 (9), 505–522. doi:10.1038/s41569-018-0064-2
Folden, D. V., Gupta, A., Sharma, A. C., Li, S. Y., and Saari, J. (2003). Malondialdehyde inhibits cardiac contractile function in ventricular myocytes via a p38 mitogen-activated protein kinase-dependent mechanism. Br. J. Pharmacol. 139 (7), 1310–1316. doi:10.1038/sj.bjp.0705384
Fuentes, T. I., Appleby, N., Raya, M., Bailey, L., Hasaniya, N., Stodieck, L., et al. (2015). Simulated microgravity exerts an age-dependent effect on the differentiation of cardiovascular progenitors isolated from the human heart. PLoS One 10 (7), e0132378. doi:10.1371/journal.pone.0132378
Fűtő, P., Markus, G., Kiss, A., and Adányi, N. (2012). Development of a catalase-based amperometric biosensor for the determination of increased catalase content in milk samples. Electroanalysis 24 (1), 107–113.
Gallo, C., Ridolfi, L., and Scarsoglio, S. (2020). Cardiovascular deconditioning during long-term spaceflight through multiscale modeling. npj Microgravity 6 (1), 27. doi:10.1038/s41526-020-00117-5
Gheorghe, A. V., and Yuchnovicz, D. E. (2015). The space infrastructure vulnerability cadastre: Orbital debris critical loads. Int. J. Disaster Risk Sci. 6 (4), 359–371. doi:10.1007/s13753-015-0073-2
Globus, R. K., and Morey-Holton, E. (2016). Hindlimb unloading: Rodent analog for microgravity. J. Appl. Physiology 120 (10), 1196–1206. doi:10.1152/japplphysiol.00997.2015
Goffart, S., Vonkleistretzow, J-C., and Wiesner, R. J. (2004). Regulation of mitochondrial proliferation in the heart: Power-plant failure contributes to cardiac failure in hypertrophy. Cardiovasc. Res. 64 (2), 198–207. doi:10.1016/j.cardiores.2004.06.030
Grimm, R. H., Neaton, J. D., and Ludwig, W. (1985). Prognostic importance of the white blood cell count for coronary, cancer, and all-cause mortality. Jama 254 (14), 1932–1937. doi:10.1001/jama.1985.03360140090031
Gubala, V., Harris, L. F., Ricco, A. J., Tan, M. X., and Williams, D. E. (2012). Point of care diagnostics: Status and future. Anal. Chem. 84 (2), 487–515. doi:10.1021/ac2030199
Gude, N. A., Broughton, K. M., Firouzi, F., and Sussman, M. A. (2018). Cardiac ageing: Extrinsic and intrinsic factors in cellular renewal and senescence. Nat. Rev. Cardiol. 15 (9), 523–542. doi:10.1038/s41569-018-0061-5
Guo, L. Y., Yang, F., Peng, L. J., Li, Y. B., and Wang, A. P. (1993). CXCL2, a new critical factor and therapeutic target for cardiovascular diseases. Clin. Exp. Hypertens., 42, 428–437. doi:10.1080/10641963.2019.1693585
Gupta, S., Ramesh, K., Ahmed, S., and Kakkar, V. (2016). Lab-on-chip technology: A review on design trends and future scope in biomedical applications. Ijbsbt 8, 311–322. doi:10.14257/ijbsbt.2016.8.5.28
Hadar, A., Gozes, I., and Gurwitz, D. (2017). “RGS2 and SIRT1 link renin angiotensin aldosterone system to alzheimer's disease,” in Neuroprotection in alzheimer's disease. Editor I. Gozes (Amsterdam: Elsevier), 239–251. doi:10.1016/b978-0-12-803690-7.00012-0
Heidt, B., Siqueira, W. F., Eersels, K., Diliën, H., van Grinsven, B., Fujiwara, R. T., et al. (2020). Point of care diagnostics in resource-limited settings: A review of the present and future of PoC in its most needed environment. Biosensors 10 (10), 133. doi:10.3390/bios10100133
Herman, A. B., Occean, J. R., and Sen, P. (2021). Epigenetic dysregulation in cardiovascular aging and disease. J. Cardiovasc Aging 1 (1), 10. doi:10.20517/jca.2021.16
Hidding, B., Königstein, T., Willi, O., Rosenzweig, J. B., Nakajima, K., and Pretzler, G. (2011). Laser-plasma-accelerators-A novel, versatile tool for space radiation studies. Nucl. Instrum. Methods Phys. Res. Sect. A Accel. Spectrom. Detect. Assoc. Equip. 636 (1), 31–40. doi:10.1016/j.nima.2011.01.090
Hinghofer-Szalkay, H. G., Noskov, V., Jezova, D., Sauseng-Fellegger, G., Fueger, G. F., Sukhanov, Y., et al. (1993). Hormonal changes with lower body negative pressure on the 6th day in microgravity in one cosmonaut. Aviat. Space Environ. Med. 64 (11), 1000–1005.
Horneck, G. (2015). “Space environment,” in Encyclopedia of astrobiology. M. Gargaud, W. M. Irvine, R. Amils, H. J. Cleaves, D. L. Pinti, and J. C. Quintanilla (Berlin, Heidelberg: Springer Berlin Heidelberg), 2293–2299. doi:10.1007/978-3-662-44185-5_1469
Hu, Y., Xia, W., and Hou, M. (2018). Macrophage migration inhibitory factor serves a pivotal role in the regulation of radiation-induced cardiac senescencethrough rebalancing the microRNA-34a/sirtuin 1 signaling pathway. Int. J. Mol. Med. 42 (5), 2849–2858. doi:10.3892/ijmm.2018.3838
Huang, J., Chen, H., Niu, W., Fam, D. W. H., Palaniappan, A., Larisika, M., et al. (2015). Highly manufacturable graphene oxide biosensor for sensitive Interleukin-6 detection. RSC Adv. 5 (49), 39245–39251. doi:10.1039/c5ra05854f
Hughson, R. L., Helm, A., and Durante, M. (2018). Heart in space: Effect of the extraterrestrial environment on the cardiovascular system. Nat. Rev. Cardiol. 15 (3), 167–180. doi:10.1038/nrcardio.2017.157
Infanger, M., Kossmehl, P., Shakibaei, M., Baatout, S., Witzing, A., Grosse, J., et al. (2006). Induction of three-dimensional assembly and increase in apoptosis of human endothelial cells by simulated microgravity: Impact of vascular endothelial growth factor. Apoptosis 11 (5), 749–764. doi:10.1007/s10495-006-5697-7
Isola, G., Polizzi, A., Santonocito, S., Alibrandi, A., and Ferlito, S. (2019). Expression of salivary and serum malondialdehyde and lipid profile of patients with periodontitis and coronary heart disease. Ijms 20 (23), 6061. doi:10.3390/ijms20236061
Jové, M., Mota-Martorell, N., Pradas, I., Martín-Gari, M., Ayala, V., and Pamplona, R. (2020). The advanced lipoxidation end-product malondialdehyde-lysine in aging and longevity. Antioxidants 9 (11), 1132. doi:10.3390/antiox9111132
Jusic, A., Thomas, P. B., Wettinger, S. B., Dogan, S., Farrugia, R., Gaetano, C., et al. (2022). Noncoding RNAs in age-related cardiovascular diseases. Ageing Res. Rev. 77, 101610. doi:10.1016/j.arr.2022.101610
Justice, J. N., Ferrucci, L., Newman, A. B., Aroda, V. R., Bahnson, J. L., Divers, J., et al. (2018). A framework for selection of blood-based biomarkers for geroscience-guided clinical trials: Report from the TAME biomarkers workgroup. Geroscience 40 (5), 419–436. doi:10.1007/s11357-018-0042-y
Kanapskyte, A., Hawkins, E. M., Liddell, L. C., Bhardwaj, S. R., Gentry, D., and Santa Maria, S. R. (2021). Space biology research and biosensor technologies: Past, present, and future. Biosensors 11 (2), 38. doi:10.3390/bios11020038
Keller, K. M., and Howlett, S. E. (2016). Sex differences in the biology and pathology of the aging heart. Can. J. Cardiol. 32 (9), 1065–1073. doi:10.1016/j.cjca.2016.03.017
Kim, E. J., Lee, J., Jung, Y. R., Park, J-J., Park, M-J., Lee, J-S., et al. (2015). Involvement of corin downregulation in ionizing radiation-induced senescence of myocardial cells. Int. J. Mol. Med. 35 (3), 731–738. doi:10.3892/ijmm.2014.2048
Kratz, S. R. A., Höll, G., Schuller, P., Ertl, P., and Rothbauer, M. (2019). Latest trends in biosensing for microphysiological organs-on-a-chip and body-on-a-chip systems. Biosensors 9 (3), 110. doi:10.3390/bios9030110
Krishnamurthy, J., Torrice, C., Ramsey, M. R., Kovalev, G. I., Al-Regaiey, K., Su, L., et al. (2004). Ink4a/Arf expression is a biomarker of aging. J. Clin. Invest. 114 (9), 1299–1307. doi:10.1172/jci22475
Krismastuti, F. S. H., Pace, S., and Voelcker, N. H. (2014). Porous silicon resonant microcavity biosensor for matrix metalloproteinase detection. Adv. Funct. Mat. 24 (23), 3639–3650. doi:10.1002/adfm.201304053
Krtolica, A., and Campisi, J. (2002). Cancer and aging: A model for the cancer promoting effects of the aging stroma. Int. J. Biochem. Cell. Biol. 34 (11), 1401–1414. doi:10.1016/s1357-2725(02)00053-5
Krüger, M., Pietsch, J., Bauer, J., Kopp, S., Carvalho, D. T. O., Baatout, S., et al. (2019). Growth of endothelial cells in space and in simulated microgravity - a comparison on the secretory level. Cell. Physiol. Biochem. 52 (5), 1039–1060. doi:10.33594/000000071
Kuilman, T., and Peeper, D. S. (2009). Senescence-messaging secretome: SMS-ing cellular stress. Nat. Rev. Cancer 9 (2), 81–94. doi:10.1038/nrc2560
Kuningas, M., Altmäe, S., Uitterlinden, A. G., Hofman, A., van Duijn, C. M., and Tiemeier, H. (2011). The relationship between fertility and lifespan in humans. AGE 33 (4), 615–622. doi:10.1007/s11357-010-9202-4
Kura, B., Kalocayova, B., LeBaron, T. W., Frimmel, K., Buday, J., Surovy, J., et al. (2019). Regulation of microRNAs by molecular hydrogen contributes to the prevention of radiation-induced damage in the rat myocardium. Mol. Cell. Biochem. 457 (1), 61–72. doi:10.1007/s11010-019-03512-z
Kuruvilla, L., Nair, R. R., Arthur, U. P. R., Lal, V., and Kartha, C. C. (2007). Endocardial endothelial celsl stimulate proliferation and collagen synthesis of cardiac fibroblasts. Cell. Biochem. Biophys. 47 (1), 65–72. doi:10.1385/cbb:47:1:65
Lai, B. F. L., Huyer, L. D., Lu, R. X. Z., Drecun, S., Radisic, M., and Zhang, B. (2017). InVADE: Integrated vasculature for assessing dynamic events. Adv. Funct. Mat. 27 (46), 1703524. doi:10.1002/adfm.201703524
Lakatta, E. G. (2003). Arterial and cardiac aging: Major shareholders in cardiovascular disease enterprises. Circulation 107 (3), 490–497. doi:10.1161/01.cir.0000048894.99865.02
Li, W., and Hudson, M. (2019). Earth's van allen radiation belts: From discovery to the van allen probes era. JGR Space Phys. 124 (11), 8319–8351. doi:10.1029/2018ja025940
Liang, L., Li, H., Cao, T., Qu, L., Zhang, L., Fan, G-C., et al. (2020). Calpain activation mediates microgravity-induced myocardial abnormalities in mice via p38 and ERK1/2 MAPK pathways. J. Biol. Chem. 295 (49), 16840–16851. doi:10.1074/jbc.ra119.011890
Liang, L., Yuan, W., Qu, L., Li, H., Zhang, L., Fan, G-C., et al. (2019). Administration of losartan preserves cardiomyocyte size and prevents myocardial dysfunction in tail-suspended mice by inhibiting p47phox phosphorylation, NADPH oxidase activation and MuRF1 expression. J. Transl. Med. 17 (1), 279. doi:10.1186/s12967-019-2021-1
Lijnen, P. J., van Pelt, J. F., and Fagard, R. H. (2012). Stimulation of reactive oxygen species and collagen synthesis by angiotensin II in cardiac fibroblasts. Cardiovasc. Ther. 30 (1), e1–e8. doi:10.1111/j.1755-5922.2010.00205.x
Linsenmayer, T. F. (1991). “Collagen,” in Cell biology of extracellular matrix. Editor E. D. Hay (Boston, MA: Springer US), 7–44. doi:10.1007/978-1-4615-3770-0_2
Lipnicki, D. M., and Gunga, H-C. (2009). Physical inactivity and cognitive functioning: Results from bed rest studies. Eur. J. Appl. Physiol. 105 (1), 27–35. doi:10.1007/s00421-008-0869-5
Liu, L., Cheng, Y., Wang, J., Ding, Z., Halim, A., Luo, Q., et al. (2020). Simulated microgravity suppresses osteogenic differentiation of mesenchymal stem cells by inhibiting oxidative phosphorylation. Ijms 21 (24), 9747. doi:10.3390/ijms21249747
Liu, Y., Zhou, Q., and Revzin, A. (2013). An aptasensor for electrochemical detection of tumor necrosis factor in human blood. Analyst 138 (15), 4321–4326. doi:10.1039/c3an00818e
López-Otín, C., Blasco, M. A., Partridge, L., Serrano, M., and Kroemer, G. (2013). The hallmarks of aging. Cell. 153 (6), 1194–1217. doi:10.1016/j.cell.2013.05.039
Low, L. A., and Giulianotti, M. A. (2020). Tissue chips in space: Modeling human diseases in microgravity. Pharm. Res. 37 (1), 8–6. doi:10.1007/s11095-019-2742-0
Lu, Y., Wan, S., Ruan, X., Liang, H., Su, J., Wang, Z., et al. (2022). Phosphoric acid induced controllable nanoparticle aggregation for ultrasensitive SERS detection of malondialdehyde in a microfluidic chip. Chemosensors 10 (12), 524. doi:10.3390/chemosensors10120524
Lubos, E., Loscalzo, J., and Handy, D. E. (2011). Glutathione peroxidase-1 in health and disease: From molecular mechanisms to therapeutic opportunities. Antioxidants Redox Signal. 15 (7), 1957–1997. doi:10.1089/ars.2010.3586
Luka, G., Ahmadi, A., Najjaran, H., Alocilja, E., DeRosa, M., Wolthers, K., et al. (2015). Microfluidics integrated biosensors: A leading technology towards lab-on-a-chip and sensing applications. Sensors 15 (12), 30011–30031. doi:10.3390/s151229783
Maier, J. A., Cialdai, F., Monici, M., and Morbidelli, L. (2015). The impact of microgravity and hypergravity on endothelial cells. Biomed. Res. Int. 2015, 434803. doi:10.1155/2015/434803
Marnett, L. J. (1999). Lipid peroxidation-DNA damage by malondialdehyde. Mutat. Res. 424 (1), 83–95. doi:10.1016/s0027-5107(99)00010-x
Marsano, A., Conficconi, C., Lemme, M., Occhetta, P., Gaudiello, E., Votta, E., et al. (2016). Beating heart on a chip: A novel microfluidic platform to generate functional 3D cardiac microtissues. Lab. Chip 16 (3), 599–610. doi:10.1039/c5lc01356a
Mehdizadeh, M., Aguilar, M., Thorin, E., Ferbeyre, G., and Nattel, S. (2022). The role of cellular senescence in cardiac disease: Basic biology and clinical relevance. Nat. Rev. Cardiol. 19 (4), 250–264. doi:10.1038/s41569-021-00624-2
Miroshnichenko, L. (2015). Solar Cosmic Rays Fundamentals and Applications (New York, NY: Springer Cham), 1–15.
Mohammed, M., and Desmulliez, M. (2014). Autonomous capillary microfluidic system with embedded optics for improved troponin I cardiac biomarker detection. Biosens. Bioelectron. 61, 478–484. doi:10.1016/j.bios.2014.05.042
Mohammed, M-I., and Desmulliez, M. P. Y. (2011). Lab-on-a-chip based immunosensor principles and technologies for the detection of cardiac biomarkers: A review. Lab. Chip 11 (4), 569–595. doi:10.1039/c0lc00204f
Mohanty, S., and Ahmad, Y. (2022). “Biosensors in the detection of oxidative stress using discovered biomarkers,” in Novel therapeutic approaches targeting oxidative stress. Editors P. K. Maurya,, and I. Qamar (Elsevier: Amsterdam), 155–170. doi:10.1016/b978-0-323-90905-1.00008-0
Moroni, L., Tabury, K., Stenuit, H., Grimm, D., Baatout, S., and Mironov, V. (2022). What can biofabrication do for space and what can space do for biofabrication? Trends Biotechnol 40 (4), 398–411.
Muñoz-Espín, D., and Serrano, M. (2014). Cellular senescence: From physiology to pathology. Nat. Rev. Mol. Cell. Biol. 15 (7), 482–496. doi:10.1038/nrm3823
Nanduri, V., Balasubramanian, S., Sista, S., Vodyanoy, V. J., and Simonian, A. L. (2007). Highly sensitive phage-based biosensor for the detection of β-galactosidase. Anal. Chim. Acta 589 (2), 166–172. doi:10.1016/j.aca.2007.02.071
Nelson, G. A. (2016). Space radiation and human exposures, a primer. Radiat. Res. 185 (4), 349–358. doi:10.1667/rr14311.1
Neufeld, M. J., and Charles, J. B. (2015). Practicing for space underwater: Inventing neutral buoyancy training, 1963-1968. Endeavour 39 (3-4), 147–159. doi:10.1016/j.endeavour.2015.05.006
Neužil, P., Giselbrecht, S., Länge, K., Huang, T. J., and Manz, A. (2012). Revisiting lab-on-a-chip technology for drug discovery. Nat. Rev. Drug Discov. 11 (8), 620–632.
Nicogossian, A. E., Williams, R. S., Huntoon, C. L., Doarn, C. R., Polk, J. D., and Schneider, V. S. (2016). Space physiology and medicine: From evidence to practice. New York, NY: Springer. doi:10.1007/978-1-4939-6652-3
Norbury, J. W., Schimmerling, W., Slaba, T. C., Azzam, E. I., Badavi, F. F., Baiocco, G., et al. (2016). Galactic cosmic ray simulation at the NASA space radiation laboratory. Life Sci. space Res. 8, 38–51. doi:10.1016/j.lssr.2016.02.001
Ouyang, M., Tu, D., Tong, L., Sarwar, M., Bhimaraj, A., Li, C., et al. (2021). A review of biosensor technologies for blood biomarkers toward monitoring cardiovascular diseases at the point-of-care. Biosens. Bioelectron. 171, 112621. doi:10.1016/j.bios.2020.112621
Pan, Y., Liang, H., Liu, H., Li, D., Chen, X., Li, L., et al. (2014). Platelet-Secreted MicroRNA-223 promotes endothelial cell apoptosis induced by advanced glycation end products via targeting the insulin-like growth factor 1 receptor. J. Immunol. 192 (1), 437–446. doi:10.4049/jimmunol.1301790
Paneni, F., Diaz Cañestro, C., Libby, P., Lüscher, T. F., and Camici, G. G. (2017). The aging cardiovascular system. J. Am. Coll. Cardiol. 69 (15), 1952–1967. doi:10.1016/j.jacc.2017.01.064
Pani, G., Verslegers, M., Quintens, R., Samari, N., de Saint-Georges, L., van Oostveldt, P., et al. (2016). Combined exposure to simulated microgravity and acute or chronic radiation reduces neuronal network integrity and survival. PLoS One 11 (5), e0155260. doi:10.1371/journal.pone.0155260
Pardo, A., and Selman, M. (2005). MMP-1: The elder of the family. Int. J. Biochem. Cell. Biol. 37 (2), 283–288. doi:10.1016/j.biocel.2004.06.017
Patel, S. (2020). The effects of microgravity and space radiation on cardiovascular health: From low-Earth orbit and beyond. IJC Heart & Vasc. 30, 100595. doi:10.1016/j.ijcha.2020.100595
Pavy-Le Traon, P-L., Heer, M., Narici, M. V., Rittweger, J., and Vernikos, J. (2007). From space to earth: Advances in human physiology from 20 years of bed rest studies (1986-2006). Eur. J. Appl. Physiol. 101 (2), 143–194. doi:10.1007/s00421-007-0474-z
Pekin, D., Skhiri, Y., Baret, J-C., Le Corre, D., Mazutis, L., Ben Salem, C. B., et al. (2011). Quantitative and sensitive detection of rare mutations using droplet-based microfluidics. Lab. Chip 11 (13), 2156–2166. doi:10.1039/c1lc20128j
Peoples, J. N., Saraf, A., Ghazal, N., Pham, T. T., and Kwong, J. Q. (2019). Mitochondrial dysfunction and oxidative stress in heart disease. Exp. Mol. Med. 51 (12), 1–13. doi:10.1038/s12276-019-0355-7
Reich, R., Supuran, C. T., and Breuer, E. (2014). “Tumor microenvironment as target in cancer therapy,” in Annual reports in medicinal chemistry. Editor M. C. Desai (Amsterdam: Elsevier), 269–284. doi:10.1016/b978-0-12-800167-7.00017-1
Remy, J., Wegrowski, J., Crechet, F., Martin, M., and Daburon, F. (1991). Long-term overproduction of collagen in radiation-induced fibrosis. Radiat. Res. 125 (1), 14–19. doi:10.2307/3577976
Ren, K., Zhou, J., and Wu, H. (2013). Materials for microfluidic chip fabrication. Acc. Chem. Res. 46 (11), 2396–2406. doi:10.1021/ar300314s
Rezaei, B., Majidi, N., Rahmani, H., and Khayamian, T. (2011). Electrochemical impedimetric immunosensor for insulin like growth factor-1 using specific monoclonal antibody-nanogold modified electrode. Biosens. Bioelectron. 26 (5), 2130–2134. doi:10.1016/j.bios.2010.09.020
Rock, K. L., Latz, E., Ontiveros, F., and Kono, H. (2010). The sterile inflammatory response. Annu. Rev. Immunol. 28, 321–342. doi:10.1146/annurev-immunol-030409-101311
Roda, A., Mirasoli, M., Guardigli, M., Zangheri, M., Caliceti, C., Calabria, D., et al. (2018). Advanced biosensors for monitoring astronauts' health during long-duration space missions. Biosens. Bioelectron. 111, 18–26. doi:10.1016/j.bios.2018.03.062
Rodgers, J. L., Jones, J., Bolleddu, S. I., Vanthenapalli, S., Rodgers, L. E., Shah, K., et al. (2019). Cardiovascular risks associated with gender and aging. J. Cardiovasc Dev. Dis. 6 (2). doi:10.3390/jcdd6020019
Russomano, T., Cardoso, R., Falcao, F., Dalmarco, G., Dos Santos, C., Dos Santos, L., et al. (2005). Development and validation of a 3D clinostat for the study of cells during microgravity simulation. Conf. Proc. IEEE Eng. Med. Biol. Soc. 2006, 564–566. doi:10.1109/IEMBS.2005.1616474
Sadhukhan, R., Majumdar, D., Garg, S., Landes, R. D., McHargue, V., Pawar, S. A., et al. (2021). Simultaneous exposure to chronic irradiation and simulated microgravity differentially alters immune cell phenotype in mouse thymus and spleen. Life Sci. space Res. 28, 66–73. doi:10.1016/j.lssr.2020.09.004
Sato, Y., Tsuboi, R., Lyons, R., Moses, H., and Rifkin, D. B. (1990). Characterization of the activation of latent TGF-beta by co-cultures of endothelial cells and pericytes or smooth muscle cells: A self-regulating system. J. Cell. Biol. 111 (2), 757–763. doi:10.1083/jcb.111.2.757
Schmitt, F-J., Renger, G., Friedrich, T., Kreslavski, V. D., Zharmukhamedov, S. K., Los, D. A., et al. (2014). Reactive oxygen species: Re-evaluation of generation, monitoring and role in stress-signaling in phototrophic organisms. Biochimica Biophysica Acta (BBA) - Bioenergetics 1837 (6), 835–848. doi:10.1016/j.bbabio.2014.02.005
Schriner, S. E., Linford, N. J., Martin, G. M., Treuting, P., Ogburn, C. E., Emond, M., et al. (2005). Extension of murine life span by overexpression of catalase targeted to mitochondria. science 308 (5730), 1909–1911. doi:10.1126/science.1106653
Selig, H., Dittus, H., and Lämmerzahl, C. (2010). Drop tower microgravity improvement towards the nano-g level for the MICROSCOPE payload tests. Microgravity Sci. Technol. 22 (4), 539–549. doi:10.1007/s12217-010-9210-0
Sengupta, P., Khanra, K., Chowdhury, A. R., and Datta, P. (2019). “Lab-on-a-chip sensing devices for biomedical applications,” in Bioelectronics and medical devices. Editors K. Pal, H-B. Kraatz, A. Khasnobish, S. Bag, I. Banerjee, and U. Kuruganti (Amsterdam: Elsevier), 47–95. doi:10.1016/b978-0-08-102420-1.00004-2
Serrano, M., Lin, A. W., McCurrach, M. E., Beach, D., and Lowe, S. W. (1997). Oncogenic ras provokes premature cell senescence associated with accumulation of p53 and p16INK4a. Cell. 88 (5), 593–602. doi:10.1016/s0092-8674(00)81902-9
Shelhamer, M. (2016). Parabolic flight as a spaceflight analog. J. Appl. Physiology 120 (12), 1442–1448. doi:10.1152/japplphysiol.01046.2015
Shirakabe, A., Zhai, P., Ikeda, Y., Saito, T., Maejima, Y., Hsu, C-P., et al. (2016). Drp1-Dependent mitochondrial autophagy plays a protective role against pressure overload-induced mitochondrial dysfunction and heart failure. Circulation 133 (13), 1249–1263. doi:10.1161/circulationaha.115.020502
Siddique, T., Deng, H. X., and Ajroud-Driss, S. (2013). “Motor neuron disease,” in Emery and rimoin's principles and practice of medical genetics. Editors D. Rimoin, R. Pyeritz, and B. Korf (Oxford: Academic Press), 1–22. doi:10.1016/b978-0-12-383834-6.00141-5
Sithara, T., and Drosatos, K. (2021). Metabolic complications in cardiac aging. Front. Physiol. 12, 669497. doi:10.3389/fphys.2021.669497
Son, K. J., Gheibi, P., Stybayeva, G., Rahimian, A., and Revzin, A. (2017). Detecting cell-secreted growth factors in microfluidic devices using bead-based biosensors. Microsyst. Nanoeng. 3, 25. doi:10.1038/micronano.2017.25
Soumetz, F. C., Pastorino, L., and Ruggiero, C. (2009). Development of a piezoelectric immunosensor for matrix metalloproteinase-1 detection. Annu. Int. Conf. IEEE Eng. Med. Biol. Soc. 2009, 2775–2778. doi:10.1109/IEMBS.2009.5333860
Sprott, R. L. (2010). Biomarkers of aging and disease: Introduction and definitions. Exp. Gerontol. 45 (1), 2–4. doi:10.1016/j.exger.2009.07.008
Steenman, M., and Lande, G. (2017). Cardiac aging and heart disease in humans. Biophys. Rev. 9 (2), 131–137. doi:10.1007/s12551-017-0255-9
Strait, J. B., and Lakatta, E. G. (2012). Aging-associated cardiovascular changes and their relationship to heart failure. Heart Fail. Clin. 8 (1), 143–164. doi:10.1016/j.hfc.2011.08.011
Suzuki, Y., Ruiz-Ortega, M., Lorenzo, O., Ruperez, M., Esteban, V., and Egido, J. (2003). Inflammation and angiotensin II. Int. J. Biochem. Cell. Biol. 35 (6), 881–900. doi:10.1016/s1357-2725(02)00271-6
Takahashi, A., Yamanouchi, S., Takeuchi, K., Takahashi, S., Tashiro, M., Hidema, J., et al. (2020). Combined environment simulator for low-dose-rate radiation and partial gravity of Moon and Mars. Life 10 (11), 274. doi:10.3390/life10110274
Taneike, M., Yamaguchi, O., Nakai, A., Hikoso, S., Takeda, T., Mizote, I., et al. (2010). Inhibition of autophagy in the heart induces age-related cardiomyopathy. Autophagy 6 (5), 600–606. doi:10.4161/auto.6.5.11947
Tang, X., Li, P-H., and Chen, H-Z. (2020). Cardiomyocyte senescence and cellular communications within myocardial microenvironments. Front. Endocrinol. 11, 280. doi:10.3389/fendo.2020.00280
Tapio, S. (2016). Pathology and biology of radiation-induced cardiac disease. J. Radiat. Res. 57 (5), 439–448. doi:10.1093/jrr/rrw064
Thevenot, D. R., Tóth, K., Durst, R. A., and Wilson, G. S. (1999). Electrochemical biosensors: Recommended definitions and classification. Pure Appl. Chem. 71 (12), 2333–2348. doi:10.1351/pac199971122333
Tomilovskaya, E., Shigueva, T., Sayenko, D., Rukavishnikov, I., and Kozlovskaya, I. (2019). Dry immersion as a ground-based model of microgravity physiological effects. Front. Physiol. 10, 284. doi:10.3389/fphys.2019.00284
Valera, E., Shia, W. W., and Bailey, R. C. (2016). Development and validation of an immunosensor for monocyte chemotactic protein 1 using a silicon photonic microring resonator biosensing platform. Clin. Biochem. 49 (1), 121–126. doi:10.1016/j.clinbiochem.2015.09.001
van der Bliek, A. M., Sedensky, M. M., and Morgan, P. G. (2017). Cell biology of the mitochondrion. Genetics 207 (3), 843–871. doi:10.1534/genetics.117.300262
Vernikos, J., and Schneider, V. S. (2010). Space, gravity and the physiology of aging: Parallel or convergent disciplines? A mini-review. Gerontology 56 (2), 157–166. doi:10.1159/000252852
Versari, S., Longinotti, G., Barenghi, L., Maier, J. A., and Bradamante, S. (2013). The challenging environment on board the international space station affects endothelial cell function by triggering oxidative stress through thioredoxin interacting protein overexpression: The ESA-SPHINX experiment. FASEB J. 27 (11), 4466–4475. doi:10.1096/fj.13-229195
Vetter, R. J. (2008). ICRP publication 103, the recommendations of the international commission on radiological protection. Health Phys. 95 (4), 445–446. doi:10.1097/01.hp.0000324200.73903.5b
Vieira Dias, J., Gloaguen, C., Kereselidze, D., Manens, L., Tack, K., and Ebrahimian, T. G. (2018). Gamma low-dose-rate ionizing radiation stimulates adaptive functional and molecular response in human aortic endothelial cells in a threshold-, dose-, and dose rate-dependent manner. Dose Response 16 (1), 1559325818755238. doi:10.1177/1559325818755238
von Zglinicki, T., Saretzki, G., Döcke, W., and Lotze, C. (1995). Mild hyperoxia shortens telomeres and inhibits proliferation of fibroblasts: A model for senescence? Exp. Cell. Res. 220 (1), 186–193. doi:10.1006/excr.1995.1305
Wagner, K-H., Cameron-Smith, D., Wessner, B., and Franzke, B. (2016). Biomarkers of aging: From function to molecular biology. Nutrients 8 (6), 338. doi:10.3390/nu8060338
Wang, G., McCain, M. L., Yang, L., He, A., Pasqualini, F. S., Agarwal, A., et al. (2014). Modeling the mitochondrial cardiomyopathy of Barth syndrome with induced pluripotent stem cell and heart-on-chip technologies. Nat. Med. 20 (6), 616–623. doi:10.1038/nm.3545
Wang, L., Ma, W., Markovich, R., Chen, J-W., and Wang, P. H. (1998). Regulation of cardiomyocyte apoptotic signaling by insulin-like growth factor I. Circulation Res. 83 (5), 516–522. doi:10.1161/01.res.83.5.516
Wang, X., and Lin, Y. (2008). Tumor necrosis factor and cancer, buddies or foes? Acta Pharmacol. Sin. 29 (11), 1275–1288. doi:10.1111/j.1745-7254.2008.00889.x
Wang, Y., Boerma, M., and Zhou, D. (2016). Ionizing radiation-induced endothelial cell senescence and cardiovascular diseases. Radiat. Res. 186 (2), 153–161. doi:10.1667/rr14445.1
Wang, Y., Shen, P., Li, C., Wang, Y., and Liu, Z. (2012). Upconversion fluorescence resonance energy transfer based biosensor for ultrasensitive detection of matrix metalloproteinase-2 in blood. Anal. Chem. 84 (3), 1466–1473. doi:10.1021/ac202627b
Weng, S., Li, X., Niu, M., Ge, B., and Yu, H-Z. (2016). Blu-ray technology-based quantitative assays for cardiac markers: From disc activation to multiplex detection. Anal. Chem. 88 (13), 6889–6896. doi:10.1021/acs.analchem.6b01604
Woodcock, E. A., and Matkovich, S. J. (2005). Cardiomyocytes structure, function and associated pathologies. Int. J. Biochem. Cell. Biol. 37 (9), 1746–1751. doi:10.1016/j.biocel.2005.04.011
Wray, D. W., Amann, M., and Richardson, R. S. (2017). Peripheral vascular function, oxygen delivery and utilization: The impact of oxidative stress in aging and heart failure with reduced ejection fraction. Heart Fail Rev. 22 (2), 149–166. doi:10.1007/s10741-016-9573-4
Wu, C-L., Yin, R., Wang, S-N., and Ying, R. (2021). A review of CXCL1 in cardiac fibrosis. Front. Cardiovasc Med. 8, 674498. doi:10.3389/fcvm.2021.674498
Wu, J., Dong, M., Santos, S., Rigatto, C., Liu, Y., and Lin, F. (2017). Lab-on-a-Chip platforms for detection of cardiovascular disease and cancer biomarkers. Sensors 17 (12), 2934. doi:10.3390/s17122934
Wu, R., and Zeng, Y. (2009). Does angiotensin II-aldosterone have a role in radiation-induced heart disease? Med. Hypotheses 72 (3), 263–266. doi:10.1016/j.mehy.2008.09.051
Yadav, A., Saini, V., and Arora, S. (2010). MCP-1: Chemoattractant with a role beyond immunity: A review. Clin. Chim. Acta 411 (21), 1570–1579. doi:10.1016/j.cca.2010.07.006
Y. Aghdam, Y., Kenchegowda, D., Holmes-Hampton, G. P., Moroni, M., and P. Ghosh, P. (2021). Impairment of IGF-1 signaling and antioxidant response are associated with radiation sensitivity and mortality. Ijms 22 (1), 451. doi:10.3390/ijms22010451
Yan, M., Sun, S., Xu, K., Huang, X., Dou, L., Pang, J., et al. (2021). Cardiac aging: From basic research to therapeutics. Oxid. Med. Cell. Longev. 2021, 9570325. doi:10.1155/2021/9570325
Yang, W., Zhang, G., Weng, W., Qiu, B., Guo, L., Lin, Z., et al. (2014). Signal on fluorescence biosensor for MMP-2 based on FRET between semiconducting polymer dots and a metal organic framework. RSC Adv. 4 (102), 58852–58857. doi:10.1039/c4ra12478b
Yin, H., Zhao, Y., Sun, Y., Liu, J., Han, Y., and Dai, Z. (2022). Effectiveness of proanthocyanidin plus trimetazidine in the treatment of non-small-cell lung cancer with radiation heart injury. Evidence-Based Complementary Altern. Med. 2022, 2338622. doi:10.1155/2022/2338622
Yuan, Q., Li, L., Tang, Y., and Zhang, X. (2020). A facile Pt-doped g-C3N4 photocatalytic biosensor for visual detection of superoxide dismutase in serum samples. Sensors Actuators B Chem. 318, 128238. doi:10.1016/j.snb.2020.128238
Yun, J., and Finkel, T. (2014). Mitohormesis. Cell. Metab. 19 (5), 757–766. doi:10.1016/j.cmet.2014.01.011
Yunt, Z. X., Aschner, Y., and Brown, K. K. (2019). Chapter 10 biomarkers in IPF. Amsterdam: Elsevier, 99–112.
Zeitlin, C., Hassler, D., Cucinotta, F., Ehresmann, B., Wimmer-Schweingruber, R., Brinza, D., et al. (2013). Measurements of energetic particle radiation in transit to Mars on the Mars science laboratory. science 340 (6136), 1080–1084. doi:10.1126/science.1235989
Zglinicki, Z., Saretzki, G., Ladhoff, J., Fagagna, F., and Jackson, S. P. (2005). Human cell senescence as a DNA damage response. Mech. Ageing Dev. 126 (1), 111–117. doi:10.1016/j.mad.2004.09.034
Zhan, Y., and Hägg, S. (2019). Telomere length and cardiovascular disease risk. Curr. Opin. Cardiol. 34 (3), 270–274. doi:10.1097/hco.0000000000000613
Zhang, B., Korolj, A., Lai, B. F. L., and Radisic, M. (2018). Advances in organ-on-a-chip engineering. Nat. Rev. Mater 3 (8), 257–278. doi:10.1038/s41578-018-0034-7
Zhang, Y., Bai, J., Wu, H., and Ying, J. Y. (2015). Trapping cells in paper for white blood cell count. Biosens. Bioelectron. 69, 121–127. doi:10.1016/j.bios.2015.02.019
Zhang, Y. S., Arneri, A., Bersini, S., Shin, S-R., Zhu, K., Goli-Malekabadi, Z., et al. (2016). Bioprinting 3D microfibrous scaffolds for engineering endothelialized myocardium and heart-on-a-chip. Biomaterials 110, 45–59. doi:10.1016/j.biomaterials.2016.09.003
Zhao, Y., Czilwik, G., Klein, V., Mitsakakis, K., Zengerle, R., and Paust, N. (2017). C-reactive protein and interleukin 6 microfluidic immunoassays with on-chip pre-stored reagents and centrifugo-pneumatic liquid control. Lab. Chip 17 (9), 1666–1677. doi:10.1039/c7lc00251c
Zhao, Y., Rafatian, N., Wang, E. Y., Wu, Q., Lai, B. F., Lu, R. X., et al. (2020). Towards chamber specific heart-on-a-chip for drug testing applications. Adv. drug Deliv. Rev. 165-166, 60–76. doi:10.1016/j.addr.2019.12.002
Zhu, P., Peng, H., and Rwei, A. Y. (2022). Flexible, wearable biosensors for digital health. Med. Nov. Technol. Devices 14, 100118. doi:10.1016/j.medntd.2022.100118
Keywords: biosensors, space exploration, biomarkers, lab-on-chip, cardiovascular ageing
Citation: Rehnberg E, Quaghebeur K, Baselet B, Rajan N, Shazly T, Moroni L, Baatout S and Tabury K (2023) Biomarkers for biosensors to monitor space-induced cardiovascular ageing. Front. Sens. 4:1015403. doi: 10.3389/fsens.2023.1015403
Received: 09 August 2022; Accepted: 17 January 2023;
Published: 03 February 2023.
Edited by:
Marcelo Vazquez, Loma Linda University, United StatesReviewed by:
Suchart Kothan, Chiang Mai University, ThailandCopyright © 2023 Rehnberg, Quaghebeur, Baselet, Rajan, Shazly, Moroni, Baatout and Tabury. This is an open-access article distributed under the terms of the Creative Commons Attribution License (CC BY). The use, distribution or reproduction in other forums is permitted, provided the original author(s) and the copyright owner(s) are credited and that the original publication in this journal is cited, in accordance with accepted academic practice. No use, distribution or reproduction is permitted which does not comply with these terms.
*Correspondence: Kevin Tabury, a3RhYnVyeUBzY2tjZW4uYmU=
†These authors have contributed equally to this work and share first authorship
Disclaimer: All claims expressed in this article are solely those of the authors and do not necessarily represent those of their affiliated organizations, or those of the publisher, the editors and the reviewers. Any product that may be evaluated in this article or claim that may be made by its manufacturer is not guaranteed or endorsed by the publisher.
Research integrity at Frontiers
Learn more about the work of our research integrity team to safeguard the quality of each article we publish.