- 1Institute for Bioengineering of Catalonia (IBEC), The Barcelona Institute of Science and Technology, Barcelona, Spain
- 2ICREA-Institució Catalana de Recerca i Estudis Avançats, Barcelona, Spain
- 3Institute of Electronics, AGH University of Science and Technology, Kraków, Poland
Scientific research in plasmonic metasurfaces has been widely widespread in the last years, motivated by the recent advances in the nanofabrication field and the increasing demand for high throughput sensing platforms. The recent advances in electronics, microfluidics, and signal processing have enabled the complete development of highly integrated devices with broad application potential. However, the progress observed from a fabrication point of view has been remarkable, led by the potential benefits metamaterials can offer in plasmonic sensing: sensor miniaturization, multiplexing opportunities, and extreme sensitivity biodetection. Although conventional top-down approaches, i.e., electron-beam lithography, have been extensively employed to develop plasmonic metasurfaces for biosensing, lithography-free bottom-up nanofabrication strategies based on nano-patterned/sculpted thin-films are candidates to surpass the limitations of top-down lithographic techniques with large-scale and high-throughput fabrication processes for 2D and 3D plasmonic metasurfaces over a broad material set. This perspective paper focuses on the challenges and opportunities to achieve lithography-free plasmonic metasurfaces by nano-patterned/sculpted thin films to conduct scalable and high-throughput plasmonic metamaterials for sensitive biosensing platforms.
Introduction
Nowadays, plasmonic sensors are the most widely used and commercialized label-free optical biosensors and have become a widespread tool for studying chemical and biochemical interactions (Liu et al., 2020). The main advantages of plasmonic sensors are the potential for direct, label-free, and real-time monitoring of molecular interactions and high-throughput surface biofunctionalization strategies. They allow direct and label-free detection of analytes with real-time kinetic information from the molecular interaction under analysis without amplification or pretreatment of the sample (Lopez et al., 2017). These advantageous features have widely widespread the novel applications of plasmonic biosensors, including new research fields from cell culture and bioprocess monitoring (Vila et al., 2021) to theranostics (Hassan et al., 2021).
The working principle of plasmonic sensing has been extensively described and reviewed over the last years (Mejía-Salazar et al., 2018). Briefly, plasmons are collective oscillations of free electrons at the interface between a thin metallic layer and a dielectric. Plasmons occur when an incident light beam with a characteristic momentum, polarization, and wavelength hits a thin metallic layer, and a portion of the light energy couples with the surface electrons of the metallic layer, which move due to excitation. The plasmonic oscillation generates a highly sensitive electromagnetic field (EM) to surface changes that decay exponentially on adjacent media (Li et al., 2014). Usually, plasmonic biosensors based on planar thin films are based on the extensively described prism (Phan et al., 2020), grating (Rossi et al., 2018), and waveguide (Gauglitz, 2020) coupling configurations.
Unlike planar thin metallic films, it is possible to overcome the use of coupling elements to generate plasmons by using metallic nanostructures in the subwavelength size; these nanostructures can be localized or can be arranged on 2D arrays of nanoantennas (known as plasmonic metasurfaces), and can be fabricated by single-layer metallic films and a combination of metallic and dielectric films. The plasmon resonances in nanostructured materials arise from the light scattering of sub-wavelength conductive nanostructures with particular absorption/reflection bands that mainly depend on the geometry and material of the nanostructure. Analogous to plasmons in planar thin films, nano-plasmons are highly sensitive to the refractive index of the surrounding media and are suitable for direct, label-free, and sensitive biosensing applications (López-Muñoz et al., 2017). The shorter evanescent decay length (up to one order of magnitude shorter) of nano-plasmons makes them more sensitive to thin biorecognition events, making them superior for biodetection of low-molecular-weight analytes (Lopez-Muñoz et al., 2021). The last has been demonstrated over various plasmonic metasurfaces with a biosensing performance above one order of magnitude and further compared to conventional plasmonic sensors based on flat thin layers over the last years. Additionally, as has been previously demonstrated, it is possible to modulate the evanescent field decay length on arrays of nanoantennas varying the incident angle of light by Rayleigh-Wood anomalies photonic phenomena (Giannini et al., 2011). Consequently, plasmonic metasurfaces can detect different biorecognition events over a broader range of biolayer thicknesses (Lopez-Muñoz et al., 2021).
In the last years, the efforts in plasmonic metasurfaces for biosensing have been mainly focused on the design and nanofabrication of novel, high-throughput, and large-scale plasmonic nanoantennas with innovative designs that usually couple different photonic phenomena to enhance their sensing performance, which can be later incorporated into biosensing platforms. Highly ordered plasmonic nanostructures can promote the generation of plasmonic surface lattice resonances (SLR). SLR couple the localized plasmon resonances associated with individual metallic nanostructures, which leads to an exciting phenomenon that drastically narrows the plasmonic resonances due to the coupling with neighbor nanostructures reinforcing or enhancing the resonance between them (Kravets et al., 2018). Consequently, longer evanescent decay lengths and bulk refractive index sensitivity are achieved, which can be highly attractive to detecting large analyte targets (Li et al., 2015). Top-down nanofabrication methods (like laser/e-beam lithography) are still the gold standard for nanoantennas fabrication. Recent approaches offer scalable, high-throughput nanofabrication (see Figure 1A) like nanostencil lithography based on shadow-masked nano-pattering and nanoimprint lithography (Su et al., 2021), where nano-patterning is based on thermal or mechanical deformation of imprint materials (Oh D. K. et al., 2021). Although the previously described processes have a high potential to achieve scalable and cost-effective nanofabrication at the wafer scale, these processes maintain the following main challenge: a master nano-mold/pattern is required to transfer metasurfaces with an associated high cost.
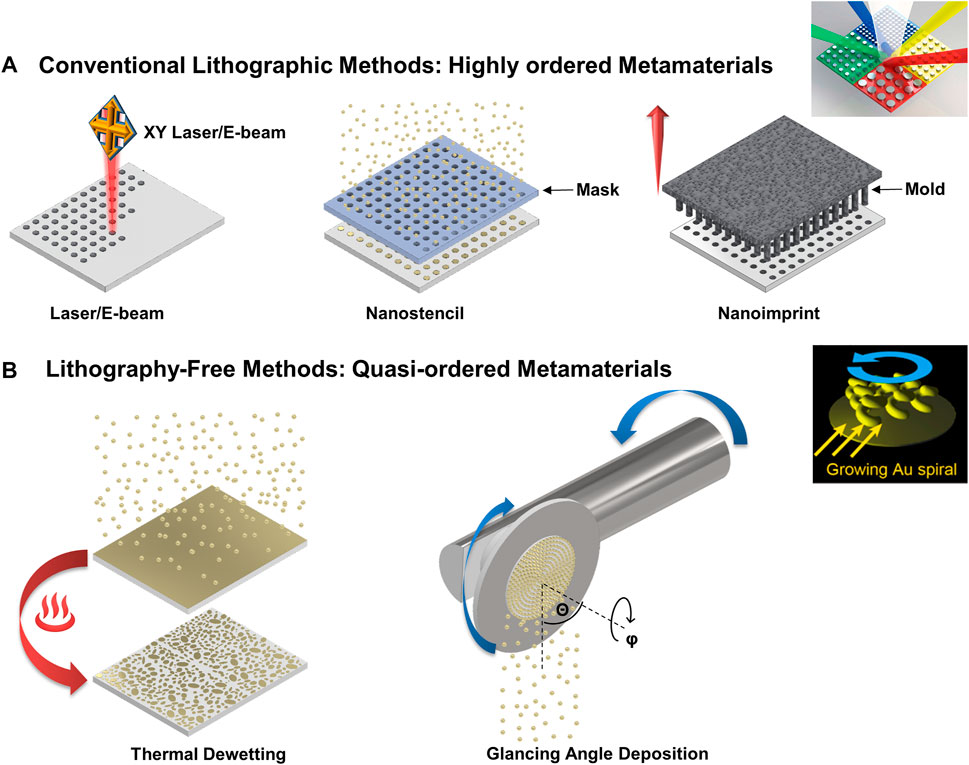
FIGURE 1. Schematic illustration of conventional lithographic nanofabrication to create highly ordered metamaterials and the lithography-free nanofabrication by nano-patterned/sculpted thin films to develop quasi-ordered metamaterials. (A) Schematic diagram representing different conventional lithographic methods from left to right: Laser/E-beam lithography, nanostencil lithography, and nanoimprint lithography. The insert shows an example of a plasmonic color filter based on highly ordered plasmonic metamaterials. Adapted and reproduced with permission from Zhao et al. (2017) and Si et al. (2013). Image © MDPI and RSC. (B) Schematic diagram representation of lithography free methods for developing quasi-ordered metamaterials from left to right: Thermal dewetting and glancing angle deposition. The insert shows the chiral plasmonic nanospirals fabrication by glanced angle deposition. Adapted and reproduced with permission from Furusawa and Kan (2020). Image © MDPI.
Thermal dewetting (TDW) and glancing angle deposition (GLAD) are alternative bottom-up nanofabrication techniques to nanopattern/sculpt thin films. These techniques do not require master nano-mold/patterns; consequently, they can achieve lithography-free large-scale plasmonic metasurfaces (Figure 1B). TDW and GLAD generate quasi-ordered plasmonic metasurfaces compared to high-order metasurfaces achieved by conventional lithographic methods. Quasi-ordered metasurfaces usually present wider plasmonic bands with shorter evanescent decay lengths and reduced bulk refractive index, the last due to multiple resonant coupling between the adjacent neighbors. Short evanescent decay length implies higher surface sensitivity for small analyte targets (Li et al., 2015). GLAD sculpts nanostructures in a single fabrication step; the nanostructures generate during the deposition of the material (Badshah et al., 2020). Meanwhile, thermal dewetting generates large-scale metallic nanostructures by post-thermal annealing of deposited metallic thin films (Qiu et al., 2018). Finally, the conventional lithographic techniques have benefited from GLAD and TDW in developing novel, highly ordered complex 3D metallic nanostructures with hyperbolic dispersion or chirality for biosensing (Ai et al., 2018). We highlight the most relevant advances in the last few years in developing plasmonic metasurfaces/nanosurfaces by nano-patter/sculpting of thin films with relevant biosensing applications. We discuss these techniques’ potential challenges and capabilities to achieve cost-effective, large-scale, high-throughput plasmonic metamaterials for biosensing on a single-step fabrication process.
Breakthroughs in Plasmonic Metasurfaces by Glancing Angle Deposition and Thermal Dewetting for Biosensing
Plasmonic Metasurfaces by Thermal Dewetting for Biosensing
TDW represents the most straightforward approach to nanopattern metallic thin films. TDW promotes nanoislands formation, taking advantage of the poor thin metallic films’ wettability/adhesion on dielectric substrates. Metallic atoms in thin metallic films tend to bind to each other rather than the substrate atoms; this behavior favors the growth of three-dimensional islands by a thermal annealing process (Bhalla et al., 2019a). The nanoislands’ geometry, size, and distribution depend mainly on the following aspects: used metal, layer thicknesses, and temperature (Aráujo et al., 2016; Quan et al., 2017). Plasmonic metasurfaces by TDW for biosensing usually use gold as plasmonic material mainly due to their excellent chemical stability and well-established biointerface generation by thiol chemistry. However, silver possesses the highest plasmonic activity among all the noble metals despite its limited chemical stability (Cheng et al., 2015). Silver represents an economically attractive material for generating plasmonic metasurfaces by TDW due to noticeable differences in materials costs and energy costs, with lower annealing times and temperatures to form low dispersion nano-domes/islands than gold (Oh H. et al., 2021; Quan et al., 2017; Sudheer et al., 2017). There have been proposed different strategies to enhance the chemical stability of silver while taking advantage of its potential benefits compared to gold; these strategies are mainly based on protective layers or alloys.
Protective layers have to be carefully selected, considering these layers can severely decrease the sensing performance of the silver nanostructures mainly due to plasmonic damping (Therrien et al., 2019). Recently, a titanium overlayer has been proposed to overcome the oxidative susceptibility of silver nanoislands with a slight increase in plasmonic sensing performance. The proposed titanium protective layer promotes galvanic coupling in the interface between the metals to minimize silver oxidation while increasing the adhesion of silver nanostructures to the dielectric substrate (Bhalla et al., 2019b). This approach achieved a biosensing performance up to the femtomolar level using physical adsorption for biodetection. Physical absorption biofunctionalization was selected mainly due to the titanium oxide formation by the galvanic coupling effect. However, as previously described, physical absorption biofunctionalization lacks high-throughput biosensing assays (Zhang et al., 2016). A thin gold overlayer below 4 nm could enable thiol chemistry on the silver-titanium nanostructures to generate robust biointerfaces with minimal impact on plasmonic sensing performance (Guner et al., 2017).
On the other hand, gold-silver alloys improve the chemical stability of silver-based plasmonic nanostructures and allow spectral tunability of the plasmonic band. The silver-gold mass ratio influences the spectral position of the plasmonic band (Qiu et al., 2018; Hwang et al., 2019). In gold-silver alloy-based plasmonic metasurfaces, plasmon-enhanced fluorescence phenomena can exploit its spectral tunability. Achieving the maximum fluorescent enhancement requires the full coupling between the plasmonic band of the nanostructure and the fluorescent label excitation band (Li et al., 2017). Gold-silver nano-domes/islands with a high silver or copper content could be used as building blocks to obtain nanoporous plasmonic metamaterials. They can enhance more than one order of magnitude biosensing performance, mainly due to a higher surface area and a high density of hot spots (Garoli et al., 2019). Nanoporous gold can be spectrally tuned by varying the etching conditions like temperature, time, or etchant concentration (Koya et al., 2021).
Plasmonic Metasurfaces by Glancing Angle Deposition for Biosensing
GLAD is a thin film deposition technique where the atoms flux of the deposited material arrives in a tilted substrate under rotation; the tilt angle is usually above 75° to maximize the self-shadowing effect and promotes nanodomes/islands nucleation (Bronicki et al., 2022). This shadowing effect causes nanopillar-like growth of the material layer (Taschuk et al., 2010). GLAD allows the fabrication of many plasmonic nanostructures by controlling different deposition parameters, such as the deposition angle of the substrate, the direction and velocity of substrate rotation, and deposition rate. The substrate’s tilt angle mainly affects the plasmonic nanostructures ' size, interspace, and growth angle for a fixed substrate rotation speed (2–20 rpm); meanwhile, a low substrate rotation rate promotes the formation of nanohelix with chiral plasmonic activity (Abbasian et al., 2016; Jen et al., 2016). The precise control and the combination of the different deposition parameters allow changing the final geometry of the nanostructures. Consequently, GLAD is a highly scalable and high-throughput technique that allows the nanosculpt of plasmonic metamaterials in a single process.
Although there are limited reports of GLAD’s plasmonic nanostructures compared to TDW for biosensing, some demonstrate GLAD’s potential performance and benefits. The most remarkable report from Zandieh et al. (2018) utilizes silver nanocolumns obtained by GLAD to detect endotoxins in buffer media with a competitive limit of detection in the pM range. They generate a self-assembled monolayer to protect silver from oxidation and create a biointerface by carbodiimide crosslinking. This approach demonstrates high stability over time with a robust biointerface with high specificity. Another exciting report from Kim et al. (2015) implies using oblique angle deposition, a variation of GLAD without azimuthal rotation at a fixed tilt sample angle of 80° to create nanoporous gold or nanoroughness in conventional thin flat films. These plasmonic substrates with top nanoroughness demonstrate a 2-fold improvement in biosensing performance under attenuated total internal reflection configuration due to the generation of nanoplasmons. These results show the potential benefits even for conventional plasmonics of GLAD.
Bioanalytical Applications
Although many of the reported biosensing applications based on plasmonic metamaterials obtained by GLAD and TDW still remain at a proof-of-concept level (mainly GLAD), promising results have been recently reported in fields ranging from food/agricultural monitoring to disease diagnostics. Besides the sensitivity issues that may be a limiting factor in some applications, especially those related to clinical diagnosis, the main problems are usually associated with the specificity and selectivity of the bioassays and, most important, the application to the biodetection in whole complex samples without previous pretreatment steps. The last advances in surface chemistry and biotechnology can help solve these challenges to achieve real-life applications with novel biointerfaces with superior antifouling properties and new recognition biomolecules.
The use of metaplasmonic-based biosensors for food, environmental, and pharmaceutical analysis is desirable for day-to-day decentralized monitoring and their desirable benefits for developing Lab-on-a-chip (LOC) and Point-of-Care (POC) devices. Mycotoxins are a relevant detection target in food safety, considering they negatively impact human and animal health. Nabok et al. (2019) proposed a combination of attenuated total internal reflection (prism coupling) with gold plasmonic metasurfaces obtained by TDW for the detection of mycotoxins, with a highly competitive limit of detection (LOD) of only 0.01 ng/ml in a direct immunoassay using “half” antibodies by cutting di-sulfide bonds with 2-mercaptoethylamine. These “half” antibodies can be directly covalent immobilized to the gold surface, taking advantage of the thiol groups simplifying the creation of the biointerfaces, and enhancing the biodetection sensitivity by decreasing the biorecognition events thickness. In environmental analysis, Qiu et al. (2020) have recently demonstrated the detection of total bioaerosols from real samples obtained in different locations around Switzerland. Bioaerosols are a complex mixture of viruses, toxins, and allergens, with a relevant influence on human health. TDW gold nanostructures were coupled in a differential interferometric detection scheme and possess succinimidyl ester groups. In contact with amine groups present in bioaerosols components, these functional groups crosslink and create amide bonds linking the bioaerosols to the gold nanostructures. They achieved a LOD of up to ≈0.5 cells/ml using bacteria such as E. coli as a model.
On the other hand, the results recently published in the clinical diagnosis are encouraging for developing biosensors for the biomarkers detection of cardiovascular diseases, e.g. myocardial infarction. Xu et al. (2020) have demonstrated the detection of cardiac troponin I by an infrared plasmon-enhanced fluorescent platform using gold plasmonic nanostructures by TDW. The approach detected concentrations down to 0.01 ng/ml in 10 µl human serum samples with a sensitivity of 100% and specificity of 95.54% in myocardial infarction patients and negative control samples. These results contribute to the design of POC devices and novel plasmonic metamaterials for precision medicine. Finally, more than 2 years ago have been since the World Health Organization (WHO) declared the novel COVID-19 as a pandemic. During this challenging period, the scientific community and pharmaceutical companies focused on the development of fast and sensitive POC platforms that could compete with the gold standard detection technique RT-PCR (reverse transcription-polymerase chain reaction) and their clear disadvantages for prompt and massive biodetection (e.g., screening test). Over time, different approaches based on plasmonic metamaterials were reported. Among, Qiu et al. (2021) presented a novel approach based on plasmonic gold nanostructures by TDW, which combines the plasmons for biodetection and plasmons for heat generation by thermoplasmonic effect. The thermoplasmonic phenomena promoted the specific nucleic acid hybridization and achieved a sensitive direct detection of nonamplified nucleic acid sequences of the SARS-CoV-2 virus. The proposed biosensor was validated with clinical samples from COVID-19 patients demonstrating their potential application.
Table 1 summarizes most of the plasmonic biosensors by GLAD and TDW reported to date, including relevant technical information like the detection method, the target biomolecule, their performance, and the advantages and disadvantages of the proposed sensing technology.
Conclusion and Future Perspective
Metaplasmonic biosensors based on nano-patterned/nanosculpted thin films by GLAD and TDW represent an enormous and promising research field that faces many unmet challenges. They are impulsed by their potential benefits and the constant growth in the market’s decentralized analysis and POC platforms. Metaplasmonic biosensors by GLAD and TDW can achieve a prominent role in these areas; meanwhile, scalability, sensitivity, and miniaturization could be met. However, surpassing one of the main challenges involves improving the nucleation distribution to achieve highly ordered and homogeneous plasmonic metasurfaces. Although several template-assisted methods have been described to reach this challenge using colloidal lithography or nanoimprint, we consider combining TDW + GLAD in a single process could improve the nucleation distribution and homogeneity. There have been described approaches that have improved the lattice and size distribution of plasmonic nanostructures using sacrificial layers of materials by TDW (Farzinpour et al., 2012). Combining TDW and GLAD in a single process can solve problems related to using silver. It seems to be feasible to TDW thin silver films in a vacuum or argon atmosphere, reducing the potential oxidation of silver during the process and continuing with the deposition of subsequent functional layers.
Although we push up to achieve fully nanotemplate-free plasmonic metasurfaces, we cannot discard a future combination of ready-to-use nanotemplates with GLAD and TDW to fabricate a wide variety of plasmonic metasurfaces in a high-throughput way. Extreme/deep UV lithography also called projection lithography (Fruncillo et al., 2021), is a nanofabrication technique that could fully benefit from TDW + GLAD to achieve complex chiral and novel functional plasmonic metamaterials. There is still a long way ahead for these technologies; the different deposition conditions possess a deep synergy, influencing the resultant metasurface. The final performance of a plasmonic biosensor involves a synergy between the biointerface and the plasmonic transducer. This correlation is rarely analyzed; the match between the biotarget size and the evanescent field decay length will severely affect the final sensitivity of the plasmonic metasurface. Considering this correlation enables the appropriate design and optimization of the nanostructures to achieve maximum performance on the desired application. In the end, GLAD and TDW-based metaplasmonic biosensors possess all the necessary features to become a competitive and outstanding option to succeed. The combination of these two methods will finally pave the way for biosensors fabrication with enhanced transducing parameters that would face the demand in rapid and screening clinical applications.
Data Availability Statement
The original contributions presented in the study are included in the article/Supplementary Material, further inquiries can be directed to the corresponding authors.
Author Contributions
GL-M, JR-A, and AR conceived this work. GL-M prepared the manuscript with the bibliographic and graphical support of AC-R under the supervision of JR-A and AR and input from all the authors.
Funding
This project received financial support from the European Research Council program under grants ERC-StG-DAMOC (714317), H2020 EU framework FET-open BLOC (863037), the Spanish Ministry of Science, Innovation and Universities through the “Severo Ochoa” Program for Centres of Excellence in R&D (CEX2018-000789-S), the CERCA Programme/Generalitat de Catalunya (2017-SGR-1079) and “la Caixa” Foundation (ID 100010434) under the agreement HR17-00268 to Javier Ramon-Azcon, and by the Polish National Agency for Academic Exchange under Bekker Program: BPN/BEK/2021/1/00015/DEC/1.
Conflict of Interest
The authors declare that the research was conducted in the absence of any commercial or financial relationships that could be construed as a potential conflict of interest.
Publisher’s Note
All claims expressed in this article are solely those of the authors and do not necessarily represent those of their affiliated organizations, or those of the publisher, the editors and the reviewers. Any product that may be evaluated in this article, or claim that may be made by its manufacturer, is not guaranteed or endorsed by the publisher.
References
Abbasian, S., Moshaii, A., Vayghan, N. S., and Nikkhah, M. (2016). Ag Nanostructures Produced by Glancing Angle Deposition with Remarkable Refractive Index Sensitivity. Plasmonics 12 (3), 631–640. doi:10.1007/s11468-016-0308-0
Ai, B., and Zhao, Y. (2018). Glancing Angle Deposition Meets Colloidal Lithography: a New Evolution in the Design of Nanostructures. Nanophotonics 8 (1), 1–26. doi:10.1515/nanoph-2018-0105
Araújo, A., Mendes, M. J., Mateus, T., Vicente, A., Nunes, D., Calmeiro, T., et al. (2016). Influence of the Substrate on the Morphology of Self-Assembled Silver Nanoparticles by Rapid Thermal Annealing. J. Phys. Chem. C 120 (32), 18235–18242. doi:10.1021/acs.jpcc.6b04283
Badshah, M. A., Michel, D., Alam, N. E., Madni, I., Abbas, N., Alameh, K., et al. (2020). Enhancing the Sensitivity of a Surface Plasmon Resonance Sensor with Glancing Angle Deposited Nanostructures. Plasmonics 15 (6), 2161–2168. doi:10.1007/s11468-020-01245-0
Bhalla, N., Jain, A., Lee, Y., Shen, A. Q., and Lee, D. (2019a). Dewetting Metal Nanofilms-Effect of Substrate on Refractive Index Sensitivity of Nanoplasmonic Gold. Nanomaterials 9 (11), 1530. [online]. doi:10.3390/nano9111530
Bhalla, N., Jamshaid, A., Leung, M. H. M., Ishizu, N., and Shen, A. Q. (2019b). Electrical Contact of Metals at the Nanoscale Overcomes the Oxidative Susceptibility of Silver-Based Nanobiosensors. ACS Appl. Nano Mat. 2 (4), 2064–2075. doi:10.1021/acsanm.9b00066
Bronicki, J., Grochala, D., and Rydosz, A. (2022). Developing GLAD Parameters to Control the Deposition of Nanostructured Thin Film. Sensors 22 (2), 651. [online]. doi:10.3390/s22020651
Cheng, Z., Wang, Z., Gillespie, D. E., Lausted, C., Zheng, Z., Yang, M., et al. (2015). Plain Silver Surface Plasmon Resonance for Microarray Application. Anal. Chem. 87 (3), 1466–1469. doi:10.1021/ac504110t
Farzinpour, P., Sundar, A., Gilroy, K. D., Eskin, Z. E., Hughes, R. A., and Neretina, S. (2012). Altering the Dewetting Characteristics of Ultrathin Gold and Silver Films Using a Sacrificial Antimony Layer. Nanotechnology 23 (49), 495604. doi:10.1088/0957-4484/23/49/495604
Fruncillo, S., Su, X., Liu, H., and Wong, L. S. (2021). Lithographic Processes for the Scalable Fabrication of Micro- and Nanostructures for Biochips and Biosensors. ACS Sens. 6 (6), 2002–2024. [online]. doi:10.1021/acssensors.0c02704
Furusawa, G., and Kan, T. (2020). Au Nanospirals Transferred onto PDMS Film Exhibiting Circular Dichroism at Visible Wavelengths. Micromachines 11 (7), 641. doi:10.3390/mi11070641
Garoli, D., Calandrini, E., Giovannini, G., Hubarevich, A., Caligiuri, V., and De Angelis, F. (2019). Nanoporous Gold Metamaterials for High Sensitivity Plasmonic Sensing. Nanoscale Horiz. 4 (5), 1153–1157. doi:10.1039/c9nh00168a
Gauglitz, G. (2020). Critical Assessment of Relevant Methods in the Field of Biosensors with Direct Optical Detection Based on Fibers and Waveguides Using Plasmonic, Resonance, and Interference Effects. Anal. Bioanal. Chem. 412 (14), 3317–3349. doi:10.1007/s00216-020-02581-0
Giannini, V., Fernández-Domínguez, A. I., Heck, S. C., and Maier, S. A. (2011). Plasmonic Nanoantennas: Fundamentals and Their Use in Controlling the Radiative Properties of Nanoemitters. Chem. Rev. 111 (6), 3888–3912. doi:10.1021/cr1002672
Gish, D. A., Nsiah, F., McDermott, M. T., and Brett, M. J. (2007). Localized Surface Plasmon Resonance Biosensor Using Silver Nanostructures Fabricated by Glancing Angle Deposition. Anal. Chem. 79 (11), 4228–4232. doi:10.1021/ac0622274
Guner, H., Ozgur, E., Kokturk, G., Celik, M., Esen, E., Topal, A. E., et al. (2017). A Smartphone Based Surface Plasmon Resonance Imaging (SPRi) Platform for On-Site Biodetection. Sensors Actuators B Chem. 239, 571–577. [online]. doi:10.1016/j.snb.2016.08.061
Hassan, M. M., Sium, F. S., Islam, F., and Choudhury, S. M. (2021). A Review on Plasmonic and Metamaterial Based Biosensing Platforms for Virus Detection. Sens. Bio-Sensing Res. 33, 100429. doi:10.1016/j.sbsr.2021.100429
Hwang, C. S. H., Ahn, M. S., Lee, Y., Chung, T., and Jeong, K. H. (2019). Ag/Au Alloyed Nanoislands for Wafer-Level Plasmonic Color Filter Arrays. Sci. Rep. 9 (1), 9082. [online]. doi:10.1038/s41598-019-45689-9
Jen, Y.-J., Huang, J.-W., Liu, W.-C., Chan, S., and Tseng, C.-H. (2016). Glancing Angle Deposited Gold Nanohelix Arrays on Smooth Glass as Three-Dimensional SERS Substrates. Opt. Mat. Express 6 (3), 697. doi:10.1364/ome.6.000697
Kim, N.-h., Choi, M., Leem, J. W., Yu, J. S., Kim, T. W., Kim, T.-S., et al. (2015). Improved Biomolecular Detection Based on a Plasmonic Nanoporous Gold Film Fabricated by Oblique Angle Deposition. Opt. Express 23 (14), 18777. doi:10.1364/oe.23.018777
Koya, A. N., Zhu, X., Ohannesian, N., Yanik, A. A., Alabastri, A., Proietti Zaccaria, R., et al. (2021). Nanoporous Metals: From Plasmonic Properties to Applications in Enhanced Spectroscopy and Photocatalysis. ACS Nano 15 (4), 6038–6060. doi:10.1021/acsnano.0c10945
Kravets, V. G., Kabashin, A. V., Barnes, W. L., and Grigorenko, A. N. (2018). Plasmonic Surface Lattice Resonances: A Review of Properties and Applications. Chem. Rev. 118 (12), 5912–5951. [online]. doi:10.1021/acs.chemrev.8b00243
Li, J.-F., Li, C.-Y., and Aroca, R. F. (2017). Plasmon-enhanced Fluorescence Spectroscopy. Chem. Soc. Rev. 46 (13), 3962–3979. [online]. doi:10.1039/c7cs00169j
Li, J., Chen, C., Lagae, L., and Van Dorpe, P. (2015). Nanoplasmonic Sensors with Various Photonic Coupling Effects for Detecting Different Targets. J. Phys. Chem. C 119 (52), 29116–29122. doi:10.1021/acs.jpcc.5b10436
Li, M., Cushing, S. K., and Wu, N. (2014). Plasmon-enhanced Optical Sensors: a Review. Analyst 140 (2), 386–406. [online]. doi:10.1039/C4AN01079E
Liu, J., Jalali, M., Mahshid, S., and Wachsmann-Hogiu, S. (2020). Are Plasmonic Optical Biosensors Ready for Use in Point-Of-Need Applications? Analyst 145 (2), 364–384. doi:10.1039/c9an02149c
Lopez, G. A., Estevez, M.-C., Soler, M., and Lechuga, L. M. (2017). Recent Advances in Nanoplasmonic Biosensors: Applications and Lab-On-A-Chip Integration. Nanophotonics 6 (1), 123–136. doi:10.1515/nanoph-2016-0101
López-Muñoz, G. A., Estevez, M.-C., Peláez-Gutierrez, E. C., Homs-Corbera, A., García-Hernandez, M. C., Imbaud, J. I., et al. (2017). A Label-free Nanostructured Plasmonic Biosensor Based on Blu-Ray Discs with Integrated Microfluidics for Sensitive Biodetection. Biosens. Bioelectron. 96, 260–267. [online]. doi:10.1016/j.bios.2017.05.020
Lopez-Muñoz, G. A., Fernández-Costa, J. M., Ortega, M. A., Balaguer-Trias, J., Martin-Lasierra, E., and Ramón-Azcón, J. (2021). Plasmonic Nanocrystals on Polycarbonate Substrates for Direct and Label-free Biodetection of Interleukin-6 in Bioengineered 3D Skeletal Muscles. Nanophotonics 10 (18), 4477–4488. doi:10.1515/nanoph-2021-0426
Mejía-Salazar, J. R., and Oliveira, O. N. (2018). Plasmonic Biosensing. Chem. Rev. 118 (20), 10617–10625. doi:10.1021/acs.chemrev.8b00359
Nabok, A., Al-Rubaye, A. G., Al-Jawdah, A. M., Tsargorodska, A., Marty, J.-L., Catanante, G., et al. (2019). [INVITED] Novel Optical Biosensing Technologies for Detection of Mycotoxins. Opt. Laser Technol. 109, 212–221. [online]. doi:10.1016/j.optlastec.2018.07.076
Oh, D. K., Lee, T., Ko, B., Badloe, T., Ok, J. G., and Rho, J. (2021). Nanoimprint Lithography for High-Throughput Fabrication of Metasurfaces. Front. Optoelectron. 14 (2), 229–251. doi:10.1007/s12200-021-1121-8
Oh, H., Pyatenko, A., and Lee, M. (2021). A Hybrid Dewetting Approach to Generate Highly Sensitive Plasmonic Silver Nanoparticles with a Narrow Size Distribution. Appl. Surf. Sci. 542, 148613. [online]. doi:10.1016/j.apsusc.2020.148613
Phan, Q.-H., Lai, Y.-R., Xiao, W.-Z., Pham, T.-T. -H., and Lien, C.-H. (2020). Surface Plasmon Resonance Prism Coupler for Enhanced Circular Birefringence Sensing and Application to Non-invasive Glucose Detection. Opt. Express 28 (17), 24889. doi:10.1364/oe.400721
Qiu, G., Gai, Z., Saleh, L., Tang, J., Gui, T., Kullak-Ublick, G. A., et al. (2021). Thermoplasmonic-Assisted Cyclic Cleavage Amplification for Self-Validating Plasmonic Detection of SARS-CoV-2. ACS Nano 15 (4), 7536–7546. doi:10.1021/acsnano.1c00957
Qiu, G., Ng, S. P., and Wu, C.-M. L. (2018). Bimetallic Au-Ag Alloy Nanoislands for Highly Sensitive Localized Surface Plasmon Resonance Biosensing. Sensors Actuators B Chem. 265, 459–467. doi:10.1016/j.snb.2018.03.066
Qiu, G., Ng, S. P., and Wu, L. C.-M. (2016). Dielectric Functionalization for Differential Phase Detecting Localized Surface Plasmon Resonance Biosensor. Sensors Actuators B Chem. 234, 247–254. [online]. doi:10.1016/j.snb.2016.04.151
Qiu, G., Yue, Y., Tang, J., Zhao, Y.-B., and Wang, J. (2020). Total Bioaerosol Detection by a Succinimidyl-Ester-Functionalized Plasmonic Biosensor to Reveal Different Characteristics at Three Locations in Switzerland. Environ. Sci. Technol. 54 (3), 1353–1362. doi:10.1021/acs.est.9b05184
Quan, J., Zhang, J., Qi, X., Li, J., Wang, N., and Zhu, Y. (2017). A Study on the Correlation between the Dewetting Temperature of Ag Film and SERS Intensity. Sci. Rep. 7 (1), 14771. [online]. doi:10.1038/s41598-017-15372-y
Rossi, S., Gazzola, E., Capaldo, P., Borile, G., and Romanato, F. (2018). Grating-Coupled Surface Plasmon Resonance (GC-SPR) Optimization for Phase-Interrogation Biosensing in a Microfluidic Chamber. Sensors 18 (5), 1621. doi:10.3390/s18051621
Si, G., Zhao, Y., Lv, J., Lu, M., Wang, M., Liu, H., et al. (2013). Reflective Plasmonic Color Filters Based on Lithographically Patterned Silver Nanorod Arrays. Nanoscale 5 (14), 6243. doi:10.1039/c3nr01419c
Su, P., Shalaginov, M., Gu, T., An, S., Li, D., Li, L., et al. (2021). Large-area Optical Metasurface Fabrication Using Nanostencil Lithography. Opt. Lett. 46 (10), 2324–2327. [online]. doi:10.1364/OL.424535
Sudheer, , Mondal, P., Rai, V. N., and Srivastava, A. K. (2017). A Study of Growth and Thermal Dewetting Behavior of Ultra-thin Gold Films Using Transmission Electron Microscopy. AIP Adv. 7 (7), 075303. doi:10.1063/1.4989823
Taschuk, M. T., Hawkeye, M. M., and Brett, M. J. (2010). Glancing Angle Deposition. Handb. Deposition Technol. Films Coatings 2010, 621–678. doi:10.1016/b978-0-8155-2031-3.00013-2
Thakur, A., Qiu, G., Ng, S.-P., Guan, J., Yue, J., Lee, Y., et al. (2017). Direct Detection of Two Different Tumor-Derived Extracellular Vesicles by SAM-AuNIs LSPR Biosensor. Biosens. Bioelectron. 94, 400–407. [online] 94. doi:10.1016/j.bios.2017.03.036
Therrien, A. J., Kale, M. J., Yuan, L., Zhang, C., Halas, N. J., and Christopher, P. (2019). Impact of Chemical Interface Damping on Surface Plasmon Dephasing. Faraday Discuss. 214 (0), 59–72. [online]. doi:10.1039/C8FD00151K
Vila, J. C., Castro-Aguirre, N., López-Muñoz, G. A., Ferret-Miñana, A., De Chiara, F., and Ramón-Azcón, J. (2021). Disposable Polymeric Nanostructured Plasmonic Biosensors for Cell Culture Adhesion Monitoring. Front. Bioeng. Biotechnol. 9. doi:10.3389/fbioe.2021.799325
Xu, W., Wang, L., Zhang, R., Sun, X., Huang, L., Su, H., et al. (2020). Diagnosis and Prognosis of Myocardial Infarction on a Plasmonic Chip. Nat. Commun. 11 (1), 1654. [online]. doi:10.1038/s41467-020-15487-3
Zandieh, M., Hosseini, S. N., Vossoughi, M., Khatami, M., Abbasian, S., and Moshaii, A. (2018). Label-free and Simple Detection of Endotoxins Using a Sensitive LSPR Biosensor Based on Silver Nanocolumns. Anal. Biochem. 548, 96–101. doi:10.1016/j.ab.2018.02.023
Zhang, P., Chen, Y.-P., Wang, W., Shen, Y., and Guo, J.-S. (2016). Surface Plasmon Resonance for Water Pollutant Detection and Water Process Analysis. TrAC Trends Anal. Chem. 85, 153–165. doi:10.1016/j.trac.2016.09.003
Keywords: plasmonic, metasurfaces, biosensor, glancing angle deposition, thermal dewetting, lithography-free
Citation: López-Muñoz GA, Cortés-Reséndiz A, Ramón-Azcón J and Rydosz A (2022) Scalable, Lithography-Free Plasmonic Metasurfaces by Nano-Patterned/Sculpted Thin Films for Biosensing. Front. Sens. 3:945525. doi: 10.3389/fsens.2022.945525
Received: 16 May 2022; Accepted: 03 June 2022;
Published: 27 June 2022.
Edited by:
Jungyul Park, Sogang University, South KoreaReviewed by:
Yue Wang, Xi’an University of Technology, ChinaCopyright © 2022 López-Muñoz, Cortés-Reséndiz, Ramón-Azcón and Rydosz. This is an open-access article distributed under the terms of the Creative Commons Attribution License (CC BY). The use, distribution or reproduction in other forums is permitted, provided the original author(s) and the copyright owner(s) are credited and that the original publication in this journal is cited, in accordance with accepted academic practice. No use, distribution or reproduction is permitted which does not comply with these terms.
*Correspondence: Gerardo A. López-Muñoz, Z2xvcGV6QGliZWNiYXJjZWxvbmEuZXU=; Javier Ramón-Azcón, anJhbW9uQGliZWNiYXJjZWxvbmEuZXU=; Artur Rydosz, cnlkb3N6QGFnaC5lZHUucGw=