- Institute for Nanotechnology and Water Sustainability (iNanoWS), College of Science, Engineering and Technology, University of South Africa, Johannesburg, South Africa
Exfoliated graphite is a 2D carbon material that has recently received great attention owing to its remarkable analytical merits such as fast electron migration, ease of surface regeneration, high-temperature resistance, ability to withstand high-current density, excellent conductivity, and sensitivity. Due to its excellent analytical signature, an exfoliated graphite electrode has been reportedly used in the construction of sensors and biosensors for various applications. This electrode can be used alone or blended with several nanomaterials/semiconductors for the degradation of various organic pollutants in wastewater. Despite the outstanding results reported in the use of exfoliated graphite electrodes for various analytical applications, very few reports were documented in the literature. Therefore, this review elaborated on the importance of exfoliated graphite electrodes for electrochemical analysis.
Introduction
In recent years, researchers have employed several methodologies and techniques to combat several issues, including water treatment, sensing of various toxic waste in water, and energy crisis to name a few (Dong et al., 2020; Bassi et al., 2021; Chen et al., 2021). Remarkable achievements have been reported in the literature in solving these problems using various analytical tools such as spectrophotometry (Al-Sulami et al., 2021), titrimetry (Basavaiah and Nagegowda, 2004), chemiluminescence (Sun et al., 2021), liquid chromatography (Alampanos and Samanidou, 2021), high-performance liquid chromatography (HPLC) (Zgagacz et al., 2020), and gas chromatography–mass spectrophotometry (GC–MS) (Xia et al., 2020). However, the shortcomings of these techniques include longer analysis time, tedious sample preparation, costly instrumentation, and non-portability of the instruments (Liang et al., 2019; Bagherinasab et al., 2020). On the other hand, the electrochemistry technique offers high sensitivity, selectivity, a higher degree of accuracy, precision, and real-time detection of environmental pollutants (Idris et al., 2020; Wu et al., 2020). Generally, electrochemistry makes use of electrodes as vehicles to carry out the electrochemical analysis. Also, electrodes are the channels where most electrochemical reactions occur and possess some interesting analytical properties such as fast electron transfer, lower toxicity, electrochemical stability, chemical stability, low background current, surface reproducibility, and amenable to functionalization (Asif et al., 2021; Liu et al., 2021). Unfortunately, most electrodes such as glassy carbon electrodes, gold electrodes, pencil graphite electrodes, and screen-printed carbon electrodes are not amenable to functionalization. Hence, a new class of electrodes known as exfoliated graphite electrode (EGE) was recently reported and can be functionalized with various nanomaterials and semiconductors for electrochemical analysis. This electrode possesses favorable analytical properties such as fast electron migration, ease of surface regeneration, excellent conductivity, and sensitivity (Yang et al., 2009).
The exfoliation of graphite has long been investigated and reported as far back as six decades ago. One of the earliest documented literatures on the exfoliation of graphite was the study of Brocklehurst, which reported that the exfoliation of graphite–Br2 began at around 300°C and expansion by about 2.1% (Brocklehurst, 1962). An expansion of approximately 1000% was reported 2 years later by Ubbelohde (Anderson and Chung, 1984). However, the mechanism of the exfoliation and intercalation processes was not fully understood. This prompted the study of Anderson and Chung on the exfoliation of intercalated graphite a few decades later, and they provided answers to questions surrounding the mechanisms of the exfoliation process (Anderson and Chung, 1984). It is worth noting that the first review article on the exfoliation of graphite by Chung presented the processes of exfoliation and the common uses of exfoliated graphite (Chung, 1987). Much attention has been given to the applications of exfoliated graphite as an electrode in recent years. However, most of its applications have been toward the construction of energy storage devices (Mitra et al., 2008; Wei et al., 2012; Sun et al., 2018).
For this reason, this review is centered on the applications of under-utilized exfoliated graphite electrodes as a surface renewed electrode for environmental applications. This review presents the discussions of recent articles (within the last 5 years) where exfoliated graphite was used to develop electrodes for the sensing of metals and organics. Also, the applications of exfoliated graphite-based photoanodes for photoelectrochemical wastewater treatments are presented. Though exfoliated graphite has also been used as a modifier for other electrodes, the articles discussed in this review are those that reported the use of exfoliated graphite as base electrodes. We believe the discussions presented in this review will be beneficial to researchers in the field of material science, electrochemistry, and wastewater treatments.
The Synthesis and Fabrication of an Exfoliated Graphite Electrode
The starting material for the preparation of exfoliated graphite or expanded graphite is usually natural graphite, and the preparation can be divided into two distinct processes, which are the intercalation process and the exfoliation process. The natural graphite first undergoes the intercalation process through treatment with chemical reagents called intercalates. Commonly, intercalates include sulfuric acid, nitric acid, alkylsulfonic acid, dichloroacetic, and bromine. When bromine is used as intercalate, the exfoliation process can be reversible at lower temperatures. However, irreversible exfoliation occurs when bromine is used at elevated temperatures (Chung, 1987). Nevertheless, a combination of concentrated nitric acid and sulfuric acid has attracted much attention as preferred intercalate because the higher expansion of graphite is often achieved when used (Chung, 2015). Perchloric acid serving as an oxidant and inserting agent has also been used as intercalate to produce sulfur-free exfoliated graphite (Zhao et al., 2014). Another approach to activate and facilitate the expansion of natural graphite is through electrochemical techniques. This is often achieved by the application of a specified potential to the graphite for a predefined duration (Palanisamy et al., 2014; Karuppiah et al., 2016). Electrochemically activated graphite is environmentally friendly, economical, and has a larger surface area than pristine graphite which is desirable (Karuppiah et al., 2014; Velmurugan et al., 2015). The exfoliation of intercalated graphite can be achieved through external heating, internal heating, and excessive electrolytic intercalation. Exfoliation through external heating is often carried out by placing the intercalated graphite in a furnace or a microwave at temperatures between 700 and 1200°C. Internal heating can be performed by applying an electric current through the intercalated graphite (Chung, 1987; Zhao et al., 2014).
In our previous work, the exfoliated graphite electrode was synthesized by adding approximately a 300 µM particle size of natural graphite flakes to a mixture containing 1:3 volume ratio of nitric acid and sulfuric acid for 24 h to form a graphite-intercalated compound (GIC). The intercalated material (IM) was meticulously washed with de-ionized water until a pH of 6.5 was attained. Thereafter, the material was exposed to thermal shock at 800 C for 30 s to compel the IM out of the graphite lattice, thus rupturing the layers (Idris et al., 2019). Hence, a puffed carbon material known as exfoliated graphite was formed. The exfoliated graphite electrode (EGE) was constructed by compressing approximately 0.4 g EG to a pellet using a pressure of 70 kPa for 4 h. The EGE was fabricated from the compressed pellet using a glass rod, copper wire, and conductive silver paint. A puncher was used to cut the electrode into various diameters depending on the application. For sensor development, it was punctured at 3–5 mm diameter for degradation studies. A blade was used to scrape off the top layer of the copper wire to remove any oxide on its surface, and the other end was coiled to form a circular shape. Thereafter, conductive silver paint was applied on the circular coil to stabilize the pellet on its surface and dried at ambient temperature. After this, the electrode was inserted into a glass tube and coated with an insulator (Araldite epoxy resin), leaving only the basal plane of the pellet. The fabricated EGE was polished with P1500-grit emery sheets to obtain a smooth uniform surface. The sequential steps for the preparation of exfoliated graphite, exfoliated graphite electrode, and exfoliated graphite composite for the construction of various photoanodes are shown in Figures 1A–C.
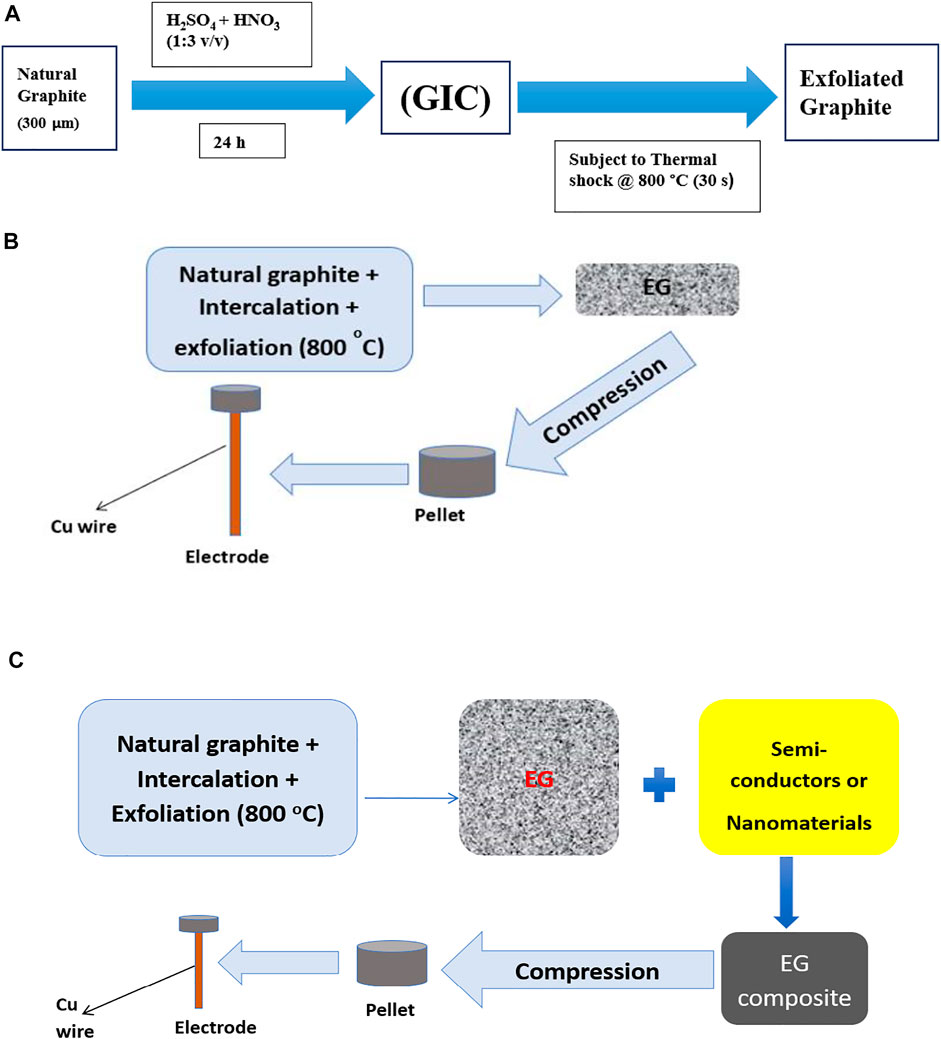
FIGURE 1. (A) Synthesis of exfoliated graphite. (B) Fabrication of an exfoliated graphite electrode using natural graphite. (C) Development of an exfoliated graphite electrode blended with semiconductor or nanomaterials for electrochemical analysis.
Characterization of Exfoliated Graphite
Typically, graphite usually exists in the form of flakes before exfoliation. After the exfoliation process, a great expansion occurs within the graphite interlayer as a result of the separation of the carbon layer. In most cases, the exfoliated graphite possesses a cellular structure with a fluffy morphology. Since large expansion happens along the c-axis, the exfoliated flake elongate in the direction corresponding to the c-axis (Chung, 2015). The cellular structure of exfoliated graphite depends on the intercalating compound. For instance, Chen and Chung et al. observed that when the intercalate is sulfuric acid, the exfoliated graphite could contain up to 60 carbon layers resulting in about 20 nm cell wall thickness (Chen and Chung, 2013).
The morphology of exfoliated graphite observed using the scanning electron microscope often revealed smooth and well-separated graphitic sheets. Such observation was reported by Zhao et al. (2014). Generally, natural graphite consists of compacted smooth interlayers of carbon sheets as shown in Figures 2A,C. As shown in Figures 2B,D, the intercalating compounds forced the compacted layers to be separated. After exfoliation due to heat treatment, a fluffy morphology of exfoliated graphite can be seen (Figures 2E,F). It can also be observed that the exfoliated graphite is composed of several openings which are well desired when a composite with other materials is to be formed.
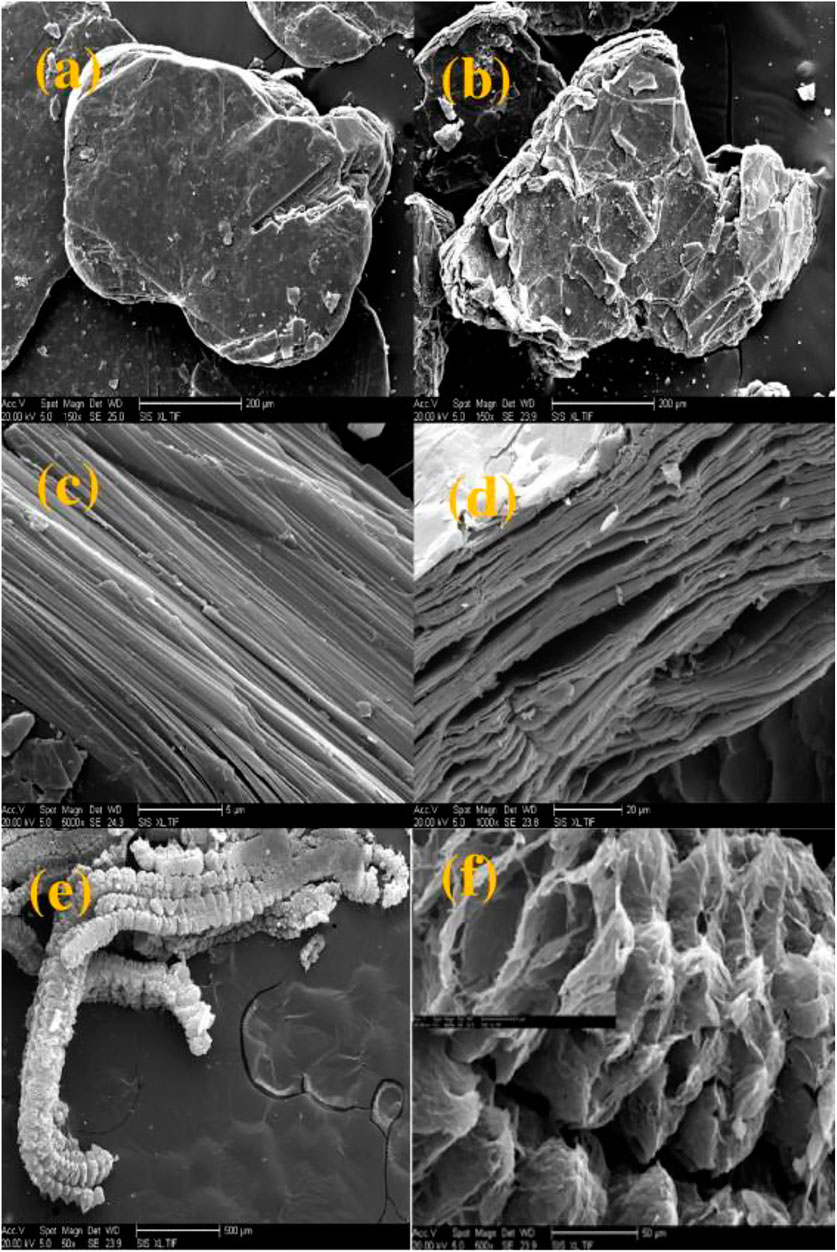
FIGURE 2. SEM images of natural graphite (A,C); intercalated graphite (B,D) and exfoliated graphite (E,D). Reproduced with permission from Elsevier [24].
Additionally, examination of exfoliated graphite using X-ray diffraction often reveals the presence of hexagonal phase of graphitic structure with a (200) diffraction plane, which is often confirmed by a characteristic peak at around 2θ ≈ 27 (Ntsendwana et al., 2016a). Furthermore, the chemical composition of exfoliated graphite has been explored using FTIR spectroscopy. It was similar to pristine graphite where n significant peaks were relating to any functional groups except that adsorbed water weak bands are often absent, and no bands of functional groups are anticipated in the FTIR spectrum of exfoliated graphite (Ţucureanu et al., 2016). However, the presence of additional peaks has been observed, which usually originates from the intercalation compounds (Shengtao et al., 2011; Umukoro et al., 2017a).
Applications of Exfoliated Graphite Electrode
As stated earlier, exfoliated graphite is a low-density material and possesses interesting analytical properties such as excellent thermal conductivity, high electrical and mechanical properties, ease of shaping, and functionalization (Mafa et al., 2016a). Several studies have documented outstanding results in the application of exfoliated graphite electrodes for electrochemical analysis. However, when inspected carefully this material has been under-utilized. The first electrochemical behavioral study of exfoliated graphite electrodes was reported by Frysz et al. in the year 1965. The author compared the electrochemical signature of this electrode with glassy carbon and the carbon paste electrode. The results obtained revealed that exfoliated graphite electrode offers lower capacitance, high electrochemical surface area (90 mm2), and better electron transfer than both electrodes (Winter and Woock, 1965). Hence, this electrode has attracted various researchers in employing exfoliated graphite electrodes for various electrochemical applications, including sensor development, water treatment, supercapacitors, and biosensors to name a few (Somashekarappa and Sampath, 2004; Ion et al., 2011; Mandal et al., 2015). The application of exfoliated graphite is discussed in the next section.
Sensing
The quest to quantify various toxic analytes using electrochemical techniques makes electrochemists develop various electrodes for the quantification of heavy metals and recalcitrant pollutants in water. For instance, Idris et al. electrodeposited bismuth film on the surface of an exfoliated graphite electrode for the voltammetry detection of As(III) in water (Ndlovu et al., 2014). The electrodeposition step was achieved by holding potential at -600 mV for 300 s in a solution containing 5 mM bismuth oxide (Bi2O3) prepared in 0.1 M HNO3. The fabricated electrode was electrochemically characterized using an Ru[(NH3)6]2+/3+ redox probe, and the result confirmed that the Bi-EGE modified electrode increases the electroactive surface area of the bare EGE from 13.1 to 16.3 mm2. The sensor was successfully used in the detection of As (III) in water with a detection limit of 5 ppb, the sensor was not susceptible to many interfering cations except Cu(II) ion. Based on these results, we recommend that the electrodeposition method should be used in the modification of the conducting substrate because it stabilizes modifiers on the electrode surface, which is far better than the drop drying and drop coating methods that make modifiers leach in the analyte solution, which affects the reproducibility and repeatability of most sensors.
An arsenic-based sensor was fabricated by electrodeposition of gold nanoparticle on the surface of exfoliated graphite electrode (Mafa et al., 2016a). The sensor was constructed by electrodeposition of 5 mM HAuCl4 on the EGE surface by cycling a potential from −0.2 to 1.2 V at a scan rate of 50 mV/s for 10 scans. Subsequently, the fabricated sensor (EG-AuNPs) was activated in 0.5 M sulfuric acid by cycling a potential from 0.6 to 1.4 V at a scan rate of 50 mV/s. Consequently, the deposition of gold nanoparticles on the EGE surface changed its color from gray to golden. Energy-dispersive X-ray spectroscopy (EDX) analysis was used to confirm the successful deposition of the gold nanoparticle on the exfoliated graphite electrode. Several parameters including optimization studies were used for the detection of As(III) in water at a deposition potential of −0.8 V, deposition time of 180 s, frequency of 5 Hz, pH 3, and pulse amplitude of 50 mV. The sensor was very selective and was not susceptible to interfering cations, including Cu(II) ion. The result obtained for this study was far better than our previous report in terms of selectivity and detection limit (Ndlovu et al., 2014).
To improve sensor performance, various nanoparticles are blended and used to modify electrochemical sensors to improve their electrochemical signature. For this reason, Arotiba et al. employed cobalt nanoparticles (CoNPs) and reduced graphene oxide (RGO) to modify exfoliated graphite electrodes for the detection of As(III) in water (Jimana et al., 2018). The reduced graphene oxide was prepared by the reduction of graphene oxide via Hummer’s method, while the (Co–RGO) nanocomposite was synthesized by adding graphene oxide and cobalt dichloride (CoCl2) in ethylene glycol (20 ml), followed by the addition of 0.1 M NaOH, the resulting solution was refluxed at 110°C for 3 h under nitrogen. The solid material was washed copiously with water and ethanol to remove impurities. Thereafter, the material was dried in an oven for 24 h at 50°C to form (Co–RGO) nanocomposite. The fabrication of the sensor system involved dispersing 5 g Co–RGO nanocomposite in dimethylformamide (DMF). The dispersing solution (20 µl) was drop-coated on the surface of exfoliated graphite electrode and dried at room temperature for 3 h. The voltammetry results revealed that there was a 50% improvement in the capacitive current of the Co–RGO composite compared to an RGO modifier. This enhancement was attributed to the excellent electrocatalytic properties of the nanoparticles, which increases the sensitivity and the electroactive surface area of the electrode. The sensor was able to detect As(III) in water at a detection limit of 0.31 ppb. The detection limit obtained indicated that the combination of CoNPs and RGO exhibited better performance than the AuNPs and Bi film (Mafa et al., 2016a; Ndlovu et al., 2014). This was attributed to the synergistic effects of both modifiers (Co and RGO), which facilitated electron communication on the conducting substrate. Hence, we recommend the coupling of excellent modifiers on conducting substrates to improve their electrochemical activity.
Noteworthy, the excellent electrocatalytic activities of cobalt oxide nanoparticles for the detection of arsenic (Jimana et al., 2018), motivated us toward employing this nanomaterial for the detection of copper in water (Ndlovu et al., 2012). Herein, the nanoparticle was networked on the exfoliated graphite electrode surface by cycling a potential from 1200 to −1100 mV for 10 cycles at a scan rate of 50 mV/s in a solution containing CoCl2 (1 mM) prepared in 0.1 M phosphate buffer solution at pH 7. After the electrodeposition process, the electrochemical behavior of EG-CoO was interrogated in the Fe[(CN)6]3−/4− redox probe to confirm its application for electroanalysis. The result obtained revealed that EG-CoO gave approximately 20% enhancement in the peak current signal, and the sensor was used to detect copper in water to a limit of 94 ppb. Additionally, the sensor was employed to detect Cu2+ ions in real water samples by an addition method with a recovery rate of 99–101%.
The application of exfoliated graphite electrode was explored by electrodepositing a poly (propylene imine) dendrimer on the EGE surface for the detection and removal of Pb(II) in water (Ndlovu et al., 2011). In this study, the sensor was prepared by electrodeposition of 10 mM Generation 2 (G2) polypropylene imine (PPI) prepared in PBS (pH 7). The electrochemistry signatures of the sensor system were monitored with cyclic voltammetry by cycling potential from −400 to 1100 mV for 10 cycles. The evidence of the electrodeposition process was confirmed by characterization of the sensor platform using cyclic voltammetry (CV) and square wave voltammetry (SWV) on the bare EGE and the PPI-EGE. Although SWV is the most sensitive electrochemical technique, the results obtained from both CV and SWV techniques affirmed that there was a 40% enhancement in the peak current signal when PPI was used to modify EGE, the reason for this increment was attributed to the large electroactive surface area induced on the exfoliated graphite electrode by the PPI dendrimer. Furthermore, the opposing charge of the redox probe (anionic) and PPI (cationic) may facilitate the concentration of the redox probe on the EGE surface. Hence, this Pb(II)-based sensor system gave a limit of detection (LOD) value of 1 ppb and the dynamic linear response value of 2.5–40 ppb. Interestingly, the exfoliated graphite electrode was able to detect and remove Pb(II) ions in water. The removal of the analyte in water was carried out using an exfoliated graphite electrode with a diameter of 40 mm as the working electrode, Ag/AgCl (3M Cl−) and platinum wire as the reference and counter electrodes, respectively. The measurements for the analyte removal in water was monitored at a constant potential of −1.0 mV for 180 s in a solution containing 150 ml of 40 ppm Pb(II) ion. Notably, an aliquot (3 ml) from the analyte was taken every 30 min for atomic absorption spectroscopy measurements to determine the removal of Pb(II) ions. The results obtained revealed that 99% of the analyte were removed from the solution. It is important to highlight that the author did not modify the exfoliated graphite electrode used in removing Pb(II) in water because dendrimer is quite expensive. However, we employed the scientific community to use cheap smart nanomaterials to investigate the removal of other notorious analytes in water using this fantastic electrode. The cyclic voltammogram of the electrodeposition of PPI on the exfoliated graphite electrode is shown in Figure 3.
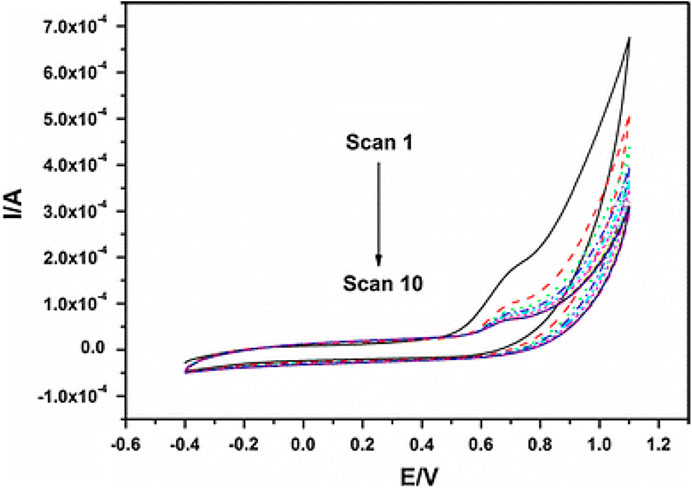
FIGURE 3. Electrodeposition of G2-PPI dendrimer on the surface of the exfoliated graphite electrode. Copyright (2011), with permission from Springer [43].
It is important to construct a smart sensor that can simultaneously detect multiple analytes because it saves cost, time, and energy. Hence, the most notable application of exfoliated graphite electrodes was the simultaneous detection of As(III), Pb(II), and Hg(II) in water using bismuth nanoparticles (BiNPs) (Mafa et al., 2016b). Here, we employed BiNPs to modify exfoliated graphite electrodes due to the ease of synthesis, low toxicity, excellent peak resolution, environmentally friendly, high hydrogen overpotential window, and insensitivity to dissolved oxygen (Mafa et al., 2016b). The bismuth film was electrodeposited on the exfoliated graphite electrode by holding potential at −1000 mV for 30 s in a solution containing 5 mM Bi2O3 prepared in 2 M nitric acid. The sensor was used to detect the analytes at different potential ranges from −200 to 300 mV (As), −700 to −200 mV (Pb), and 200–700 mV (Hg) for individual detection and −700–800 mV for the simultaneous detection of As(III), Hg(II), and Pb(II) (Figure 4). The metal ion that was not stripped off from the exfoliated graphite electrode surface during detection was stripped off by holding the potential at 600 mV for 100 s using square wave voltammetry. The sensor was used to detect As(III), Hg(II), and Pb(II) with the detection limits of 0.014, 0.081, and 0.053 ppb, respectively. Also, the sensor was used for individual detection of Pb(II) and Hg(II) in 0.1 M acetate buffer solution (pH 5), the detection limits of 0.83 and 0.46 ppb were obtained. The results obtained agree with the inductively coupled plasma-optical emission spectrometric technique.
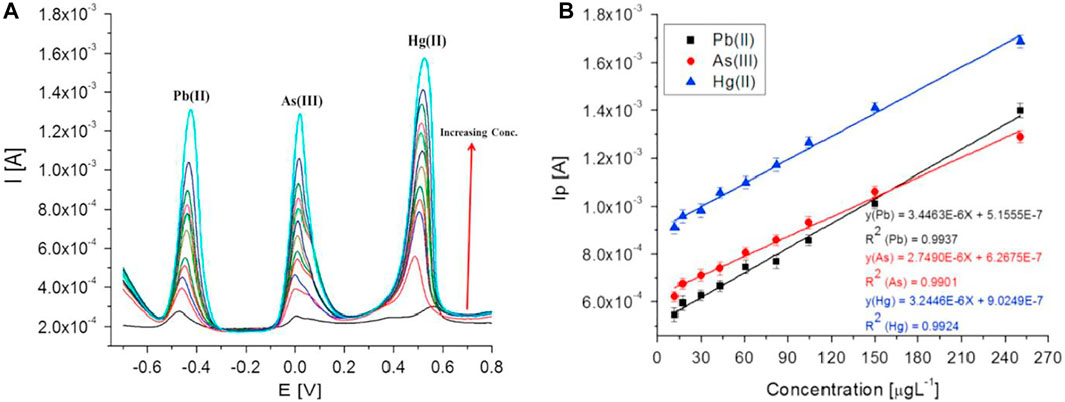
FIGURE 4. (A) SWASV of simultaneous detection of Pb(II), As(III), and Hg(II) using a bismuth film-modified exfoliated graphite electrode (B) Linear calibration graph showing current vs. concentration of the analytes. Copyright permission (2016), with permission from Elsevier.
The analytical properties of exfoliated graphite can be improved by functionalizing it with biological molecules, this helps to incorporate some functional groups such as carboxylic, carbonyl, and hydroxyl functional groups. These functional groups help to capture the analyte on the electrode surface. Due to this, Sampath et al. functionalized exfoliated graphite with dopamine (DA) for the simultaneous detection of ethanol and oxidation of nicotinamide adenine dinucleotide hydrogen (NADH) using the amperometry technique (Ramesh et al., 2002). The carboxylic acid on the EGE surface was covalently attached to the primary amine on dopamine (DA) to form amide bond linkage. The covalent attachment was carried out by adding 60 mg dicylohexylcarbodiimide (DCC) and 250 mg of exfoliated graphite into a solution containing 20 ml of trichloromethane (CHCl3) for 300 s. Thereafter, DA (50 mg) was added to the resulting solution and stirred for 4 days, the modified material was extracted with methanol using Soxhlet extraction. The fabricated sensor was used to detect ethanol in phosphate buffer (pH 7.2) using alcohol dehydrogenase enzyme and NAD+. The sensor detected ethanol within a linear range of 1–4 mM in a steady state mode at 0.15 V.
Sampath et al. employed an unmodified exfoliated graphite electrode for the selective quantification of dopamine (DA) in the presence of ascorbic acid (AA) (Ramesh et al., 2004). The exfoliation temperature and the edge plane played significant roles in the effective separation of the analytes and the activation of the conducting substrate. The effective separation of both analytes was due to the repulsive interactions between the functional groups on the EGE surface and the ascorbate anion. This was confirmed by comparing the pKa of the AA with the point of zero charge (pHpzc) of the EGE surface, using pH titration. The chronoamperometry technique was used to detect DA (50 nM) in the presence of 100 µM AA. The result revealed that the fabricated sensor selectively and successfully detected DA in the presence of AA.
Another research group decorated bismuth oxide nanotiles on the surface of exfoliated graphite for the quantification of imipramine (antidepression drug) (Yamuna et al., 2020). Bi2O3-EG was synthesized by the sono-hydrolysis-assisted exfoliation method. Briefly, a mixture of graphite (50 mg) and Bi(NO3)3 (150 mg) was dispersed in 1 M NaOH (40 ml) solution. The resulting mixture was sonicated for (1, 2, and 3 h) on a sonication bath. During sonication, Bi(NO3)3 was hydrolyzed to α-Bi2O3, and the graphite was exfoliated to graphene sheets. The equations for the reaction are shown in Eqs 1, 2. Although the author did not write this equation, we encouraged researchers to use equation(s) to show the reaction that takes place during synthesis. Hence, we proposed the chemical reactions that took place during the synthesis of bismuth oxide in Eqs 1, 2.
The Bi2O3-EG nanocomposite obtained was used to modify a screen-printed electrode for the detection of imipramine (IMPR). The electrochemical studies affirmed that the electrode fabricated from the modifier sonicated for 3 h gave the best electrochemical performance in terms of low peak potential separation, electrochemical active surface area, and high peak current signal. The author reported that long time (3 h) sonication assisted in exfoliating the graphite, incorporating more functional groups, and increased the electrode active sites. Hence, the efficient heterogeneous rate constant (koeff) was calculated using the Nicholson method in Eqs 3, 4.
where Ψ is the kinetic parameter, which is a function of ∆Ep and α = 0.5. F is the Faraday constant, R is the rate constant, n is the number of moles, and D is the diffusion coefficient.
Bi2O3-EG sonicated for 3 h gave the highest koeff value of 5.0 × 10−3, followed by 1.9 × 10−3, 2.0 × 10−3, and 1.2 × 10−4 cm s−1 for Bi2O3-EG (2 h), Bi2O3-EG (1 h), and bare SPCE, respectively. Hence, Bi2O3-EG (3 h sonication) was used to detect IMPR with a detection limit and sensitivity of 6 nM and 0.414 μA μM−1cm−2. The proposed scheme for the electrochemical pathway of imipramine is depicted in Scheme 1.
Balau et al. employed gum guar polymer to stabilize copper oxide on exfoliated graphite for the electrochemical determination of hydrogen peroxide in milk and pharmaceutical samples (Balu et al., 2019). The nanocomposite was prepared by a two-step process. First, double-distilled (DD) water was added into 5 mg of guar powder, and the resulting solution was sonicated until a clear solution appeared. Thereafter, natural graphite (5 mg) was added into guar solution, and the mixture was sonicated for 45 min at ambient temperature. Furthermore, 0.15 g (urea) and 0.2323 g Cu(NO3)2 were added into the GR-guar composite solution, and the mixture was sonicated for half an hour. The mixture was adjusted using ammonia solution. The resulting mixture was transferred into an autoclave and heated at 140 C for 3 h. A black precipitate of GR-guar/CuO composite was obtained after washing with DD and ethanol. It is important to highlight the role of each material in the nanocomposite. First, the exfoliated graphite was used to enhance the electrochemical activity of the sensor; gum guar was employed as a green dispersing agent to prevent the re-stacking of the exfoliated graphite and to stabilize the CuO nanoparticles. The analytical reports showed that the sensor is highly selective and sensitive toward the detection of hydrogen peroxide. The fabricated sensor successfully detected hydrogen peroxide in milk and commercial lens cleaning solution. The author reported that the excellent properties of GR-guar (high conductivity, large surface area, and more edge plane defects), and high porosity of the CuO nanoparticles are responsible for the fast diffusion and sensitive electro-reduction of the analyte. The proposed mechanisms for the electrochemical reduction of H2O2 by the modifiers are shown in Eqs 5, 6. The possible electrochemical mechanism of the reduction of hydrogen peroxide is shown in Scheme 2.
A notable study was reported in the literature on the application of exfoliated graphite electrodes for the simultaneous detection of ascorbic acid (AA), dopamine (DA), and uric acid (UA) (Cai et al., 2014). An exfoliated graphite with rough edges displayed excellent electrocatalytic activity toward the detection of the analytes with well-separated voltammetric peaks. The peak separation occurred at -100, 110, and 230 mV for AA, DA, and UA, respectively. The pH studies revealed that the overall process for the determination of the analytes is proton dependent and involves two electrons and two protons in the oxidation of the analytes as shown in Scheme 3. The optimized oxidation of the three analytes was found to be achieved at pH 7. Remarkably, the oxidation peak currents of these analytes increase linearly with their concentrations; this showed that the platform is stable and displayed excellent electrocatalytic activity toward the oxidation of AA, DA, and UA. The excellent electrocatalytic activity of the EGE was attributed to a large amount of edge plane-like defects, which effectively decreased the overpotential and increased the oxidation currents of the analyte. This platform was used to detect DA and UA in human urine samples.
To date, we are the first to report the application of exfoliated graphite electrodes for the detection of carcinoembryonic antigen-a cancer biomarker (Idris et al., 2019). In this report, we immobilized a nanocomposite containing carbon nanodot and poly (propylene imine) dendrimer on the exfoliated graphite surface due to the electrostatic attraction between the modifiers, dual-biocompatibility, and supramolecular chemistry of the dendrimer. It is important to highlight that supramolecular interactions maintain the natural conformity of the biological molecules; we recommend that nanomaterials with excellent supramolecular properties should be used in biosensor development because it offers a closer representation of nature (biomimicry), and thus an impressive bio-recognition for biomolecules.
In a recent study by Plekhanova et al. (2022), a biosensor based on thermally modified exfoliated graphite was developed. The modification of the exfoliated graphite was achieved using an additional oxidation process with thermal treatment by microwave irradiation which increased the orderliness of the graphite layers. The additional expansion of exfoliated graphite increased the distance between the individual graphite layers resulting in the formation of more cavities into which other desired nanoparticles or bacteria can be immobilized. The modified exfoliated graphite was used to develop a biosensor for glucose within a detection range of 0.75–10 mM. It was observed that the modification of exfoliated graphite led to an increase in the sensitivity of biosensors to glucose, specifically from 3.57 to 8.28 µA x mM−1 x cm−2. The findings from the study revealed the prospect of using modified exfoliated graphite to develop second and third generation biosensors and microbial fuel cells. The summary of recent studies on exfoliated graphite-based sensors is presented in Table 1.
Application of the Exfoliated Graphite Electrode for Wastewater Treatment
The problem of water scarcity and the access to portable clean water has necessitated the development of various water treatment methods that can effectively remove various water pollutants in water (Alkhouzaam and Qiblawey, 2021; Li et al., 2021; Mwafy et al., 2021). Different conventional methods such as absorption, decomposition, biological, and advanced oxidation processes (AOPs) have been effectively used for water treatment (Azbar et al., 2004; Qi et al., 2011; Wang et al., 2019; Zhai et al., 2020). However, reports have shown that both biological methods and decomposition methods of water treatments have failed to produce clean water, especially for wastewater obtained from the textile industry (Kavitha et al., 2014). Also, the application of AOPs for water treatment has become unappealing owing to the high voltage needed for the electrochemical degradation process and the generation of chlorine as a reaction by-product (Coha et al., 2021).
Recently, the promising environmentally friendly technique for the efficient and effective removal of organic pollutants in water is the photoelectrocatalytic process (PEC). The PEC is the synergy of heterogeneous photocatalysis and electrochemical oxidation process; both techniques are used for the removal of recalcitrant organic pollutants in water. The PEC is a form of the advanced oxidation process that involves the in situ generation of active oxidizing species, including superoxide radicals, hydroxyl radicals, and holes, which oxidizes organic pollutants to carbon dioxide and water (Figure 5). In PEC, both electric and light energy is used to produce these radicals, and it involves electrolysis and photocatalysis techniques. Solar energy is used to minimize the cost in PEC, and the phenomenon is known as solar photocatalysis (SPEC) (Zhao et al., 2021). The photoanodes employed for PEC are fabricated by the immobilization of photoactive substance materials on a conducting substrate such as conducting quartz glass, anodized titanium sheet, polished stainless steel, fluorine-doped tin oxide (FTO) glass, and exfoliated graphite. Among the conducting substrates, exfoliated graphite possesses the following important analytical properties such as high mechanical, thermal, electrical conductivities, high-temperature resistance, high specific surface area, flexibility, resilience, corrosion resistance, and compressibility (Mafa et al., 2016a). Hence, due to the excellent conductivity of exfoliated graphite, it has been used in the fabrication of various photoanodes for the degradation of stubborn organic pollutants in water. For instance, Yong et al. reported the application of unmodified exfoliated graphite for the degradation of methyl orange (MO) in artificial wastewater (Kong et al., 2011). The reactor (Figure 4) was assembled by placing the exfoliated graphite electrode (anode) and copper cathode vertically and parallelly to each other with a gap of 1.5 cm in 40 ml (MO) solution. The analyte was prepared in 0.1 M NaCl as a supporting electrolyte because it enhanced the degradation efficiency and reduced the reaction time (Kong et al., 2011). The MO was indirectly oxidized because of the reduction of NaCl to chlorite ions during electrolysis. The equations for the indirect oxidation are shown in Eqs 7–9.
It is important to highlight that the decolourization of the MO was independent of electrolysis time, current density, and the concentration of both NaCl and MO. Hence, it was deduced that the electrochemical oxidation of the analyte had little influence on its color removal but a great impact on the chemical oxygen demand of the analyte. The UV–vis analysis revealed that intermediate products are formed during the degradation of MO, and FTIR studies confirmed that the presence of the –OH functional group in exfoliated graphite was responsible for the chemical adsorption of MO molecules.
The SEM images of the exfoliated graphite and the exfoliated graphite electrode are depicted in Figure 6.
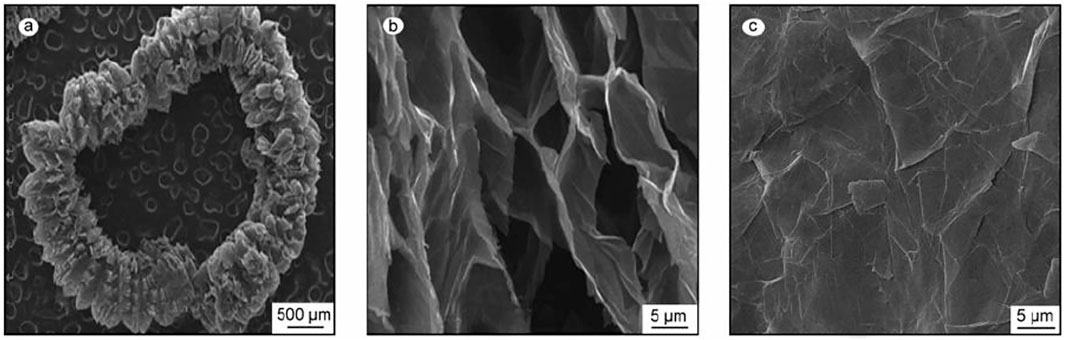
FIGURE 6. SEM image of EG (A) before and (B,C) after it was compressed into an electrode. Copyright permission from Elsevier (2011).
Similarly, unmodified exfoliated graphite was used to adsorb Congo red (CR) dye in aqueous solutions (Van Pham et al., 2019). The EG was prepared using Hummer`s method via intercalation with sulfuric acid and hydrogen peroxide followed by exfoliation in a microwave system (Figure 7). It is important to highlight that the intercalation process played a significant role in enhancing the recyclability and absorbability of the dye. Different optimization parameters such as initial concentration of the dye (20–60 mg/L), contact time (0–210 min), EG dosage (0.3–0.7 g/L), and pH (4–10) were interrogated. The pH study revealed that the adsorption process of the dye decreases as pH values increases because of the electrostatic repulsion between the anionic CR molecules and the negative charge on the EG surface. Also, the high porosity and great adsorption capacity of EG aided the removal of CR in water during the first 10 min and attained equilibrium after 150 min. The isotherm and adsorption study affirmed that the adsorption of CR onto exfoliated graphite obeyed the multilayer mechanism and chemisorption.
In another study, exfoliated graphite was prepared and blended with titanium oxide for the photoelectrochemical degradation of methylene blue under visible light radiation (Ama and Arotiba, 2017a). The titanium oxide (TiO2) was prepared by adding polyethylene glycol (4 ml) to 200 ml isopropanol, and a magnetic stirrer was used to stir the solution for 45 min, followed by the addition of titanium (IV) isopropoxide (40 ml), and the resulting mixture was stirred for 1 h. Thereafter, 20 ml of deionized water was added to initiate the formation of a precipitate, the solution was magnetically stirred for 1 h and the precipitate was dried at 100°C for 12 h, and calcined at 500°C for 3 h. The photoanode was prepared by adding deionized water to a mixture of exfoliated graphite and the prepared TiO2 in a ratio of 1:1. The resulting solution was stirred for half a day, sonicated, and dried at 50°C. EG/TiO2 was compressed to a pellet and used to fabricate an electrode or a photoanode as shown in Scheme 1. The photoanode degraded the organic dye for 240 min at a degradation efficiency of 85%. It is important to highlight that exfoliated graphite played the following roles: 1) it was used as the substrate for the immobilisation of the TiO2, 2) it provided surplus electrons in the conduction band for the semi-conductor, 3) it shuttled and captured electrons, thereby lengthening the life-span of the photogenerated charge carriers, 4) it aided in the adsorption of the organic dye, and 5) EG promoted the production of hydroxyl radicals, which played a pivotal role in the oxidation of the analyte in water.
Similarly, the photoanode (EG/TiO2) was employed for the degradation of pharmaceuticals in water (Peleyeju et al., 2017a). The photoanode was used in the degradation of sulfamethazole (SMZ), and the intermediate products were investigated. The photoanode degraded the analyte after 6 h and approximately 100% of the SMZ was removed from water and 90% chemical oxygen demand (COD) was obtained. The UV–vis spectrophotometer was used to monitor the reduction in the concentrated SMZ (antibiotic drug) during degradation, and liquid chromatography–mass spectrophotometry (LCMS) was used to identify the intermediate products. The results obtained from LCMS analysis affirmed that the PEC degradation of SMZ involved β-,γ-,δ-,ε-cleavages, hydroxylation, and ring openings. The proposed PEC degradation pathway for the analyte is shown in Figure 8.
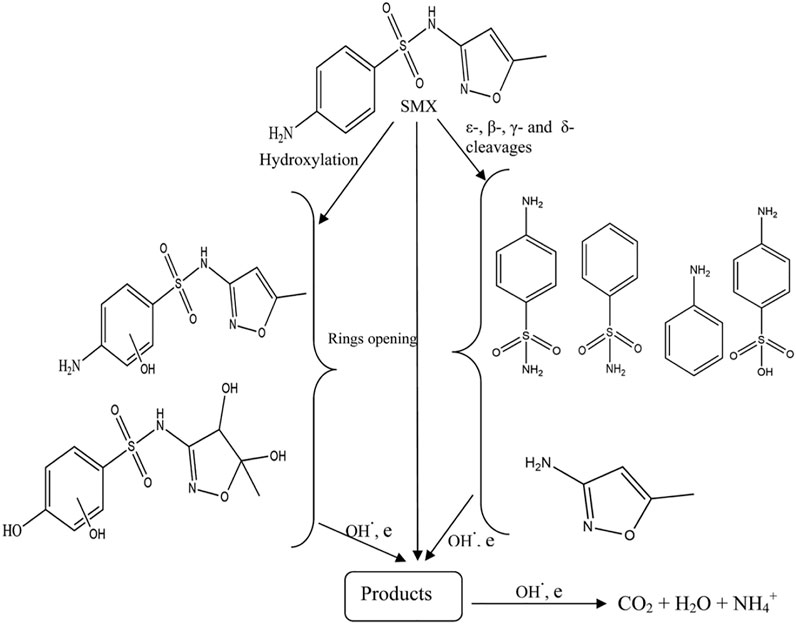
FIGURE 8. Pathway for the mineralization of SMZ using LCMS. Copyright permission from RSC Advance (2017).
The quest to utilize solar energy from the Sun activated our interest in using exfoliated graphite-ZnO nanocomposite for the photodegradation of eosin yellow under visible light irradiation (Ntsendwana et al., 2016b). We employed ZnO for this study because it possesses the following analytical properties, which include high electron mobility, relatively cheap, large exciton binding energy (60 meV), and close bandgap energy with TiO2. The advantage of ZnO over TiO2 is that the former absorbs a larger fraction of the solar spectrum and has more light quanta than the latter. Also, it displays higher reaction and mineralization rates than TiO2 due to the production of efficient hydroxyl ions. Before the degradation of eosin yellow, the pH studies were investigated because wastewater containing dyes are usually discharged at various pH. In addition to this, most semiconductors are amphoteric; therefore, their surface charge is affected by pH, which influences the surface reaction of the photocatalysts. The pH studies were carried out from 1.5 to 12, the result revealed that the degradation efficiency of eosin yellow decreases as the pH value increases. The lowest degradation efficiency was observed at pH 12 because the zero-point charge of ZnO is 9, which means that at a higher pH value the surface charge of the semiconductor is negatively charged. Thus, the anionic dye is repelled by the negative charge on the semiconductor surface leading to lower degradation efficiency at higher pH. On the contrary, acidic pH (1.5) enhanced the degradation of the dye because of electrostatic attraction between the positive surface charge of ZnO and the negative charge of eosin yellow. The degradation efficiency and kinetic rate of 93% and 11.0 × 10−3 min−1 were obtained for the degradation of the analyte using the prepared photoanode. We recommend that researchers should probe the interaction that takes place between the semiconductor(s) and the pollutant to be degraded.
One-pot synthesis of Pd–ZnO exfoliated graphite nanocomposite was used for the removal of acid orange 7 dye in water (Umukoro et al., 2017b). The exfoliated graphite in the nanocomposite helped to shuttle and capture electrons, thus, acting as an electron sink, and helped to minimize recombination of the photogenerated electron–hole pair. While Pd was used as a dopant to reduce the electron–hole pair recombination and scavenge photogenerated electrons. The holes and electrons generated during irradiation migrate to the photoanode surface to facilitate the reduction–oxidation environment suitable for the degradation of the pollutant. The mechanism of the mineralization of the organic pollutant involves the following: the holes react with the water molecules to form hydroxyl radicals (*OH) and protons, while the electrons react with the oxygen molecule from the wastewater to form superoxide (*O2). The radicals react with the protons to form hydroperoxide radicals (*HO2), and the radicals react continuously with the analyte to degrade it to CO2 and H2O. The proposed mechanism for the photocatalytic activity of the photoanode is shown in Scheme 4.
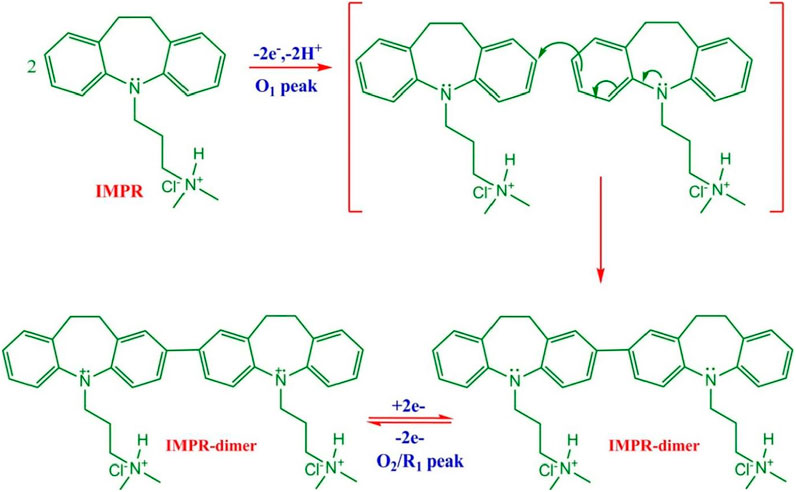
SCHEME 1. Pathway for the electrochemical oxidation of IMPR using Bi2O3-EG. Copyright permission from Elsevier (Yamuna et al., 2020).
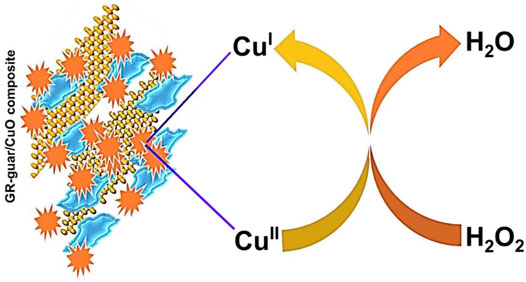
SCHEME 2. Proposed electrochemical mechanism for the reduction of hydrogen peroxide on the fabricated sensor. Copyright permission from Elsevier, 2019 (Yamuna et al., 2020).
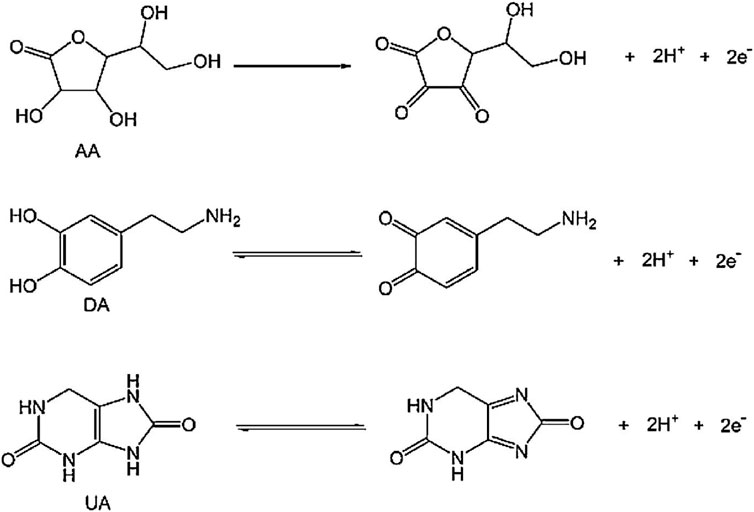
SCHEME 3. Electrocatalytic oxidation of AA, DA, and UA on EGE. Copyright permission (2014) from Elsevier (Yamuna et al., 2020).
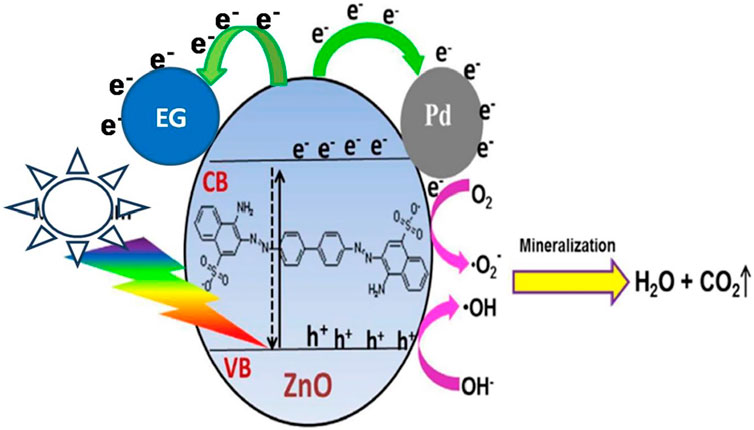
SCHEME 4. Mechanism for the photocatalytic activity of Pd-ZnO-EG toward the degradation of acid orange 7 dye. Copyright permission from Elsevier (2017).
We employed EG-BiVO4@ZnO nanocomposite for the degradation of rhodamine B in water. A heterojunction was formed between BiVO4 and ZnO to overcome the problem of rapid recombination of photogenerated electron–hole pairs and to improve the photocatalytic ability of the semiconductors (Orimolade et al., 2019a). A control experiment was performed by fabricating different types of electrodes (bare EG, EG-ZnO, EG-BiVO4, and EG-BiVO4@ZnO) for the degradation of the analyte. The degradation efficiency of 27, 52, 61, and 91% were obtained for EG, EG-ZnO, EG-BiVO4, and EG-BiVO4@ZnO, respectively. EG-BiVO4 (2.4 eV) gave better degradation efficiency than that of EG-ZnO (3.4 eV) because it has lower bandgap energy in the visible light region. While the nanocomposite gave the best photocatalytic activities owing to the formation of heterojunction, which enhanced better charge separation and reduces the recombination process of electron–hole pairs, resulting in better light harvesting.
Similarly, we prepared exfoliated graphite bismuth vanadate for the photoelectrochemical degradation of acid orange 7 dye using hydrothermal synthesis (Orimolade and Arotiba, 2019). EG-BiVO4 was used to degrade the analyte using the electrochemical (without light) and photoelectrochemical (PEC) techniques. The result obtained revealed that the degradation efficiency of the former was 66%, while the latter gave 81%; the improved degradation efficiency of the PEC was attributed to the photocatalytic activity of the semiconductor and the combined synergy of electrical energy and solar energy, which were beneficial for the generation of hydroxyl radicals required for the degradation of the dye molecules. In this study, we employed a bare exfoliated graphite electrode without immobilization of the BiVO4 for the degradation of the analyte, a degradation efficiency of 55% was obtained, which was attributed to the electrooxidation of the exfoliated graphite surface with minimal generation of hydroxyl radical due to the absence of a photocatalyst. The proposed equation for the hydrothermal synthesis of BiVO4 is shown in Eq (10). We encouraged researchers to write the equation that takes place during synthesis.
A novel photoanode was constructed from expanded graphite p–n MoS2–SnO2 heterojunction nanocomposite for the photoelectrocatalytic degradation of ciprofloxacin, a pharmaceutical pollutant (Umukoro et al., 2018). The PEC study on the degradation was performed using photoanode (EG-p–n MoS2–SnO2) in a solution of 20 ppm ciprofloxacin (100 ml) prepared in 0.1 M sodium sulfate as a supporting electrolyte. Various parameters such as current density, pH, and mass of MoS2 in the nanocomposite were optimized. Thereafter, an aliquot of 3 ml of the analyte was taken every 30 min from the photoreactor using a disposable syringe over a time range of 180 min. The aliquot was filtered, and a UV–visible spectrophotometer was used to determine the removal efficiency of the ciprofloxacin at an absorption band of ʎ = 271 nm. The photoanode was able to degrade the analyte by 86%, which validated its potential for the removal of organic pollutants in wastewaters. Recent studies on the application of exfoliated graphite electrodes for the removal of pollutants through photoelectrochemical oxidation are summarized in Table 2.
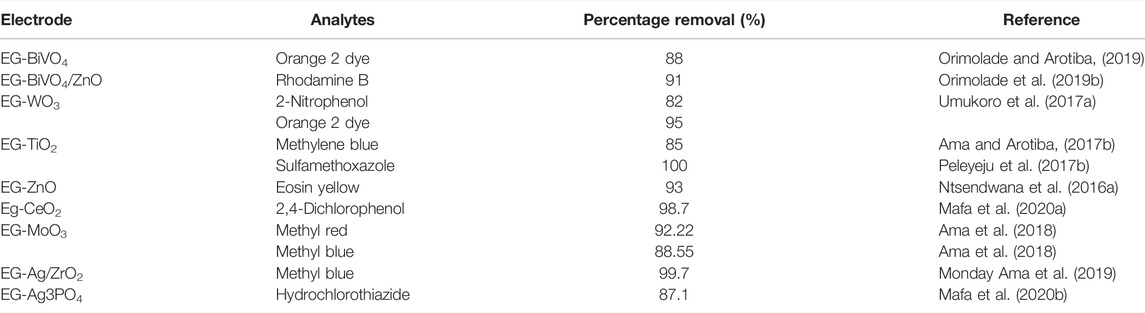
TABLE 2. Summary of reports on exfoliated graphite electrodes for photoelectrochemical wastewater treatment.
Conclusion
The incorporation of exfoliated graphite into nanomaterials and semiconductors has opened greater doors of opportunities for the construction of sensors, biosensors, and photoanodes. In this review, we discussed the preparation of exfoliated graphite electrode and how it was enveloped with various nanocomposites for the construction of various sensors in the detection of heavy metals and biological molecules. This novel exfoliated graphite electrode can be upscaled to boost its surface area, which will help in the mineralization of stubborn organic pollutants in real wastewater.
We recommend the following: 1) the electrodeposition method should be used to immobilize modifiers on conducting substrates because it is better than drop drying and drop coating methods that make modifiers leach in analytical solution during electrochemical analysis, 2) during degradation studies, researchers should probe the intermediate products formed during the degradation of analyte because some products are more toxic than parent compounds, 3) researchers should use chemical equations to validate the reaction that occurs during synthesis, 4) the interaction that takes place between the semiconductors and the analyte of interest should be studied, 5) the chemistry and interactions that take place when more than one modifier is used for the construction of a photoreactor should be investigated, and 6) the precursors used for the preparation of modifiers should be environmentally friendly and affordable.
Unfortunately, despite various fantastic results reported in the literature in the application of exfoliated graphite electrodes, very scanty reports have been documented. We recommend this novel electrode to scientific communities and industrial researchers for real-life applications because of its potential to be upscaled and employed for various applications.
Author Contributions
Conceptualization, writing, formal analysis, investigation, data curation, and original draft preparation, AI, BO and MP. Methodology, visualization, supervision, software, review and editing, critical revision, acquisition, analysis or interpretation of data for the work project administration, and funding acquisition, UF, TN and BM.
Conflict of Interest
The authors declare that the research was conducted in the absence of any commercial or financial relationships that could be construed as a potential conflict of interest.
Publisher’s Note
All claims expressed in this article are solely those of the authors and do not necessarily represent those of their affiliated organizations, or those of the publisher, the editors, and the reviewers. Any product that may be evaluated in this article, or claim that may be made by its manufacturer, is not guaranteed or endorsed by the publisher.
References
Al-Sulami, A. F., Mohammed, G. I., Alwael, H., Abduljabbar, T. N., Ismail, I. M. I., Bahaidarah, E. A., et al. (2021). Dual Wave β-correction Spectrophotometry for Trace Determination and Chemical Speciation of As(III)/As(V) in Water. Microchemical J. 162, 105856–105859. doi:10.1016/j.microc.2020.105856
Alampanos, V., and Samanidou, V. (2021). An Overview of Sample Preparation Approaches Prior to Liquid Chromatography Methods for the Determination of Parabens in Biological Matrices. Microchemical J. 164, 105995. doi:10.1016/j.microc.2021.105995
Alkhouzaam, A., and Qiblawey, H. (2021). Functional GO-Based Membranes for Water Treatment and Desalination: Fabrication Methods, Performance and Advantages. A Review. Chemosphere 274, 129853. doi:10.1016/j.chemosphere.2021.129853
Ama, O. M., and Arotiba, O. a. (2017). Exfoliated Graphite/titanium Dioxide for Enhanced Photoelectrochemical Degradation of Methylene Blue Dye under Simulated Visible Light Irradiation. J. Electroanalytical Chem. 803, 157–164. doi:10.1016/j.jelechem.2017.09.015
Ama, O. M., and Arotiba, O. A. (2017). Exfoliated Graphite/titanium Dioxide for Enhanced Photoelectrochemical Degradation of Methylene Blue Dye under Simulated Visible Light Irradiation. J. Electroanalytical Chem. 803, 157–164. doi:10.1016/j.jelechem.2017.09.015
Ama, O. M., Kumar, N., Adams, F. V., and Ray, S. S. (2018). Efficient and Cost-Effective Photoelectrochemical Degradation of Dyes in Wastewater over an Exfoliated Graphite-MoO3 Nanocomposite Electrode. Electrocatalysis 9, 623–631. doi:10.1007/s12678-018-0471-5
Anderson, S. H., and Chung, D. D. L. (1984). Exfoliation of Intercalated Graphite. Carbon 22, 253–263. doi:10.1016/0008-6223(84)90169-6
Asif, A., Heiskanen, A., Emnéus, J., and Keller, S. S. (2021). Pyrolytic Carbon Nanograss Electrodes for Electrochemical Detection of Dopamine. Electrochimica Acta 379, 138122. doi:10.1016/j.electacta.2021.138122
Azbar, N., Yonar, T., and Kestioglu, K. (2004). Comparison of Various Advanced Oxidation Processes and Chemical Treatment Methods for COD and Color Removal from a Polyester and Acetate Fiber Dyeing Effluent. Chemosphere 55, 35–43. doi:10.1016/j.chemosphere.2003.10.046
Bagherinasab, Z., Beitollahi, H., Yousefi, M., Bagherzadeh, M., and Hekmati, M. (2020). Rapid Sol Gel Synthesis of BaFe12O19 Nanoparticles: An Excellent Catalytic Application in the Electrochemical Detection of Tramadol in the Presence of Acetaminophen. Microchemical J. 156, 104803–104809. doi:10.1016/j.microc.2020.104803
Balu, S., Palanisamy, S., Velusamy, V., and Yang, T. C. K. (2019). Sonochemical Synthesis of Gum Guar Biopolymer Stabilized Copper Oxide on Exfoliated Graphite: Application for Enhanced Electrochemical Detection of H2O2 in Milk and Pharmaceutical Samples. Ultrason. Sonochem. 56, 254–263. doi:10.1016/j.ultsonch.2019.04.023
Basavaiah, K., and Nagegowda, P. (2004). Determination of Ranitidine Hydrochloride in Pharmaceutical Preparations by Titrimetry and Visible Spectrophotometry Using Bromate and Acid Dyes. Il Farmaco 59, 147–153. doi:10.1016/j.farmac.2003.11.012
Bassi, M. d. J., Wouk, L., Renzi, W., Oliveira, C. K., Duarte, J. L., Heisler, I. A., et al. (2021). Non-radiative Energy Transfer in Aqueously Dispersed Polymeric Nanoparticles for Photovoltaic Applications. Synth. Met. 275, 116740–116747. doi:10.1016/j.synthmet.2021.116740
Brocklehurst, J. E. (1962). Thermal Expansion Measurements on Graphite-Bromine Compounds. Nature 194, 247–249. doi:10.1038/194247a0
Cai, W., Lai, T., Du, H., and Ye, J. (2014). Electrochemical Determination of Ascorbic Acid, Dopamine and Uric Acid Based on an Exfoliated Graphite Paper Electrode: A High Performance Flexible Sensor. Sensors Actuators B: Chem. 193, 492–500. doi:10.1016/j.snb.2013.12.004
Chen, P.-H., and Chung, D. D. L. (2013). Viscoelastic Behavior of the Cell wall of Exfoliated Graphite. Carbon 61, 305–312. doi:10.1016/j.carbon.2013.05.009
Chen, Z., Tong, K., Xu, F., Xue, M., Chen, H., Chen, Q., et al. (2021). Development of Supercritical Water Oxidation Technology for Application to Hazardous Waste Treatment: An Extreme Case Study. J. Environ. Chem. Eng. 9, 105296. doi:10.1016/j.jece.2021.105296
Chung, D. D. L. (2015). A Review of Exfoliated Graphite. J. Mater. Sci. 51, 554–568. doi:10.1007/s10853-015-9284-6
Chung, D. D. L. (1987). Exfoliation of Graphite. J. Mater. Sci. 22, 4190–4198. doi:10.1007/BF01132008
Coha, M., Farinelli, G., Tiraferri, A., Minella, M., and Vione, D. (2021). Advanced Oxidation Processes in the Removal of Organic Substances from Produced Water: Potential, Configurations, and Research Needs. Chem. Eng. J. 414, 128668. doi:10.1016/j.cej.2021.128668
Dong, Y., Zhou, M., and Zhang, L. (2020). Designed 3D Porous Core-Shell Vanadium Oxide Microspheres for the Simultaneous Electrochemical Sensing of Toxic Metal Ions. J. Alloys Comp. 827, 154357. doi:10.1016/j.jallcom.2020.154357
Idris, A., Mabuba, N., and Arotiba, O. (2019). An Exfoliated Graphite-Based Electrochemical Immunosensor on a Dendrimer/carbon Nanodot Platform for the Detection of Carcinoembryonic Antigen Cancer Biomarker. Biosensors 9, 39. doi:10.3390/bios9010039
Idris, A. O., Mamba, B., and Feleni, U. (2020). Poly (Propylene Imine) Dendrimer: A Potential Nanomaterial for Electrochemical Application. Mater. Chem. Phys. 244, 122641–122649. doi:10.1016/j.matchemphys.2020.122641
Ion, I., Ion, A. C., and Culetu, A. (2011). Application of an Exfoliated Graphite Nanoplatelet-Modified Electrode for the Determination of Quintozen. Mater. Sci. Eng. C 31, 1553–1557. doi:10.1016/j.msec.2011.07.004
Jimana, A., Peleyeju, M. G., Tshwenya, L., Pillay, K., and Arotiba, O. A. (2018). Voltammetric Analysis of As(III) at a Cobalt Nanoparticles/reduced Graphene Oxide Modified Exfoliated Graphite Electrode. Int. J. Electrochem. Sci. 13, 10127–10140. doi:10.20964/2018.11.09
Karuppiah, C., Palanisamy, S., Chen, S.-M., Ramaraj, S. K., and Periakaruppan, P. (2014). A Novel and Sensitive Amperometric Hydrazine Sensor Based on Gold Nanoparticles Decorated Graphite Nanosheets Modified Screen Printed Carbon Electrode. Electrochimica Acta 139, 157–164. doi:10.1016/j.electacta.2014.06.158
Karuppiah, C., Velmurugan, M., Chen, S.-M., Devasenathipathy, R., Karthik, R., and Wang, S.-F. (2016). Electrochemical Activation of Graphite Nanosheets Decorated with Palladium Nanoparticles for High Performance Amperometric Hydrazine Sensor. Electroanalysis 28, 808–816. doi:10.1002/elan.201500453
Kavitha, S. R., Umadevi, M., Janani, S. R., Balakrishnan, T., and Ramanibai, R. (2014). Fluorescence Quenching and Photocatalytic Degradation of Textile Dyeing Waste Water by Silver Nanoparticles. Spectrochimica Acta A: Mol. Biomol. Spectrosc. 127, 115–121. doi:10.1016/j.saa.2014.02.076
Kong, Y., Wang, Z.-l., Wang, Y., Yuan, J., and Chen, Z.-d. (2011). Degradation of Methyl orange in Artificial Wastewater through Electrochemical Oxidation Using Exfoliated Graphite Electrode. New Carbon Mater. 26, 459–464. doi:10.1016/S1872-5805(11)60092-9
Li, D., Zhuge, Y., Liu, Y., Pham, P. N., Zhang, C., Duan, W., et al. (2021). Reuse of Drinking Water Treatment Sludge in Mortar as Substitutions of Both Fly Ash and Sand Based on Two Treatment Methods. Construction Building Mater. 277, 122330. doi:10.1016/j.conbuildmat.2021.122330
Liang, W., Liu, L., Li, Y., Ren, H., Zhu, T., Xu, Y., et al. (2019). Nitrogen-rich Porous Carbon Modified Electrochemical Sensor for the Detection of Acetaminophen. J. Electroanalytical Chem. 855, 113496. doi:10.1016/j.jelechem.2019.113496
Liu, H., Xu, T., Liu, K., Zhang, M., Liu, W., Li, H., et al. (2021). Lignin-based Electrodes for Energy Storage Application. Ind. Crops Prod. 165, 113425. doi:10.1016/j.indcrop.2021.113425
Mafa, J. P., Mabuba, N., and Arotiba, O. a. (2016). An Exfoliated Graphite Based Electrochemical Sensor for As(III) in Water. Electroanalysis 28, 1462–1469. doi:10.1002/elan.201501107
Mafa, P. J., Idris, A. O., Mabuba, N., and Arotiba, O. A. (2016). Electrochemical Co-detection of As(III), Hg(II) and Pb(II) on a Bismuth Modified Exfoliated Graphite Electrode. Talanta 153, 99–106. doi:10.1016/j.talanta.2016.03.003
Mafa, P. J., Mamba, B. B., and Kuvarega, A. T. (2020). Photoelectrocatalytic Evaluation of EG-CeO2 Photoanode on Degradation of 2,4-dichlorophenol. Solar Energ. Mater. Solar Cell 208, 110416. doi:10.1016/j.solmat.2020.110416
Mafa, P. J., Patala, R., Mamba, B. B., Liu, D., Gui, J., and Kuvarega, A. T. (2020). Plasmonic Ag3PO4/EG Photoanode for Visible Light-Driven Photoelectrocatalytic Degradation of Diuretic Drug. Chem. Eng. J. 393, 124804. doi:10.1016/j.cej.2020.124804
Mandal, S., Pal, A., Arun, R. K., and Chanda, N. (2015). Gold Nanoparticle Embedded Paper with Mechanically Exfoliated Graphite as Flexible Supercapacitor Electrodes. J. Electroanalytical Chem. 755, 22–26. doi:10.1016/j.jelechem.2015.07.025
Masemola, D. P., Mafa, P. J., Nyoni, H., Mamba, B. B., and Msagati, T. A. M. (2020). Gold Nanoparticles Modified Exfoliated Graphite Electrode as Electrochemical Sensor in the Determination of Psychoactive Drug. J. Environ. Sci. Health B 55, 455–461. doi:10.1080/03601234.2020.1713670
Mitra, S., Lokesh, K. S., and Sampath, S. (2008). Exfoliated Graphite-Ruthenium Oxide Composite Electrodes for Electrochemical Supercapacitors. J. Power Sourc. 185, 1544–1549. doi:10.1016/j.jpowsour.2008.09.014
Monday Ama, O., Wilson, A. W., and Ray, S. S. (2019). Photoelectrochemical Degradation of Methylene Blue Dye under Visible Light Irradiation Using EG/Ag-ZrO2 Nanocomposite Electrodes. Int. J. Electrochem. Sci. 14, 9982–10001. doi:10.20964/2019.10.41
Mwafy, E. a., Gaafar, M. S., Mostafa, A. M., Marzouk, S. Y., and Mahmoud, I. S. (2021). Novel Laser-Assisted Method for Synthesis of SnO2/MWCNTs Nanocomposite for Water Treatment from Cu (II). Diamond Relat. Mater. 113, 108287–108288. doi:10.1016/j.diamond.2021.108287
Ndlovu, T., Arotiba, O. a., Sampath, S., Krause, R. W., and Mamba, B. B. (2012). Electroanalysis of Copper as a Heavy Metal Pollutant in Water Using Cobalt Oxide Modified Exfoliated Graphite Electrode. Phys. Chem. Earth, Parts A/B/C 50-52, 127–131. doi:10.1016/j.pce.2012.08.007
Ndlovu, T., Arotiba, O. a., Sampath, S., Krause, R. W., and Mamba, B. B. (2011). Electrochemical Detection and Removal of lead in Water Using Poly(propylene Imine) Modified Re-compressed Exfoliated Graphite Electrodes. J. Appl. Electrochem. 41, 1389–1396. doi:10.1007/s10800-011-0360-6
Ndlovu, T., Mamba, B. B., Sampath, S., Krause, R. W., and Arotiba, O. a. (2014). Voltammetric Detection of Arsenic on a Bismuth Modified Exfoliated Graphite Electrode. Electrochimica Acta 128, 48–53. doi:10.1016/j.electacta.2013.08.084
Ntsendwana, B., Sampath, S., Mamba, B. B., Oluwafemi, O. S., and Arotiba, O. A. (2016). Photoelectrochemical Degradation of Eosin Yellowish Dye on Exfoliated Graphite-ZnO Nanocomposite Electrode. J. Mater. Sci. Mater. Electron. 27, 592–598. doi:10.1007/s10854-015-3793-6
Ntsendwana, B., Sampath, S., Mamba, B. B., Oluwafemi, O. S., and Arotiba, O. a. (2016). Photoelectrochemical Degradation of Eosin Yellowish Dye on Exfoliated Graphite-ZnO Nanocomposite Electrode. J. Mater. Sci. Mater. Electron. 27, 592–598. doi:10.1007/s10854-015-3793-6
Orimolade, B. O., and Arotiba, O. A. (2019). An Exfoliated Graphite-Bismuth Vanadate Composite Photoanode for the Photoelectrochemical Degradation of Acid Orange 7 Dye. Electrocatalysis 10, 429–435. doi:10.1007/s12678-019-00534-5
Orimolade, B. O., Koiki, B. a., Zwane, B. N., Peleyeju, G. M., Mabuba, N., and Arotiba, O. a. (2019). Interrogating Solar Photoelectrocatalysis on an Exfoliated Graphite-BiVO4/ZnO Composite Electrode towards Water Treatment. RSC Adv. 9, 16586–16595. doi:10.1039/c9ra02366f
Orimolade, B. O., Koiki, B. A., Zwane, B. N., Peleyeju, G. M., Mabuba, N., and Arotiba, O. A. (2019). Interrogating Solar Photoelectrocatalysis on an Exfoliated Graphite-BiVO4/ZnO Composite Electrode towards Water Treatment. RSC Adv. 9, 16586–16595. doi:10.1039/c9ra02366f
Palanisamy, S., Karuppiah, C., Chen, S.-M., and Periakaruppan, P. (2014). Highly Sensitive and Selective Amperometric Nitrite Sensor Based on Electrochemically Activated Graphite Modified Screen Printed Carbon Electrode. J. Electroanalytical Chem. 727, 34–38. doi:10.1016/j.jelechem.2014.05.025
Peleyeju, M. G., Umukoro, E. H., Tshwenya, L., Moutloali, R., Babalola, J. O., and Arotiba, O. a. (2017). Photoelectrocatalytic Water Treatment Systems: Degradation, Kinetics and Intermediate Products Studies of Sulfamethoxazole on a TiO2-Exfoliated Graphite Electrode. RSC Adv. 7, 40571–40580. doi:10.1039/c7ra07399b
Peleyeju, M. G., Umukoro, E. H., Tshwenya, L., Moutloali, R., Babalola, J. O., and Arotiba, O. A. (2017). Photoelectrocatalytic Water Treatment Systems: Degradation, Kinetics and Intermediate Products Studies of Sulfamethoxazole on a TiO2-Exfoliated Graphite Electrode. RSC Adv. 7, 40571–40580. doi:10.1039/c7ra07399b
Plekhanova, Y., Tarasov, S., Kitova, A., Kolesov, V., Kashin, V., Sundramoorthy, A. K., et al. (2022). Modification of Thermally Expanded Graphite and its Effect on the Properties of the Amperometric Biosensor. 3 Biotech. 12, 1–9. doi:10.1007/s13205-021-03107-w
Qi, L., Wang, X., and Xu, Q. (2011). Coupling of Biological Methods with Membrane Filtration Using Ozone as Pre-treatment for Water Reuse. Desalination 270, 264–268. doi:10.1016/j.desal.2010.11.054
Ramesh, P., Sivakumar, P., and Sampath, S. (2002). Renewable Surface Electrodes Based on Dopamine Functionalized Exfoliated Graphite:. J. Electroanalytical Chem. 528, 82–92. doi:10.1016/S0022-0728(02)00888-4
Ramesh, P., Suresh, G. S., and Sampath, S. (2004). Selective Determination of Dopamine Using Unmodified, Exfoliated Graphite Electrodes. J. Electroanalytical Chem. 561, 173–180. doi:10.1016/j.jelechem.2003.08.002
Shengtao, Z., Anyan, G., Huanfang, G., and Xiangqian, C. (2011). Characterization of Exfoliated Graphite Prepared with the Method of Secondary Intervening. Int. J. Ind. Chem. 2, 123–130.
Silva, T. R., Smaniotto, A., and Vieira, I. C. (2018). Exfoliated Graphite Nanoplatelets and Gold Nanoparticles Based Electrochemical Sensor for Determination of Levodopa. J. Solid State. Electrochem. 22, 1277–1287. doi:10.1007/s10008-017-3677-1
Somashekarappa, M. P., and Sampath, S. (2004). Sol-gel Derived, Silicate-Phthalocyanine Functionalized Exfoliated Graphite Based Composite Electrodes. Analytica Chim. Acta 503, 195–201. doi:10.1016/j.aca.2003.10.031
Sun, Y., Gao, P., Han, R., Luo, C., and Wei, Q. (2021). A Target-Triggered Signal Chemiluminescence Sensor for Prostate Specific Antigen Detection Based on Hollow Porous Silica Encapsulated Luminol by Aptamers. Sensors Actuators B: Chem. 333, 129543. doi:10.1016/j.snb.2021.129543
Sun, Z., Cai, X., Feng, D.-Y., Huang, Z.-H., Song, Y., and Liu, X.-X. (2018). Hybrid Iron Oxide on Three-Dimensional Exfoliated Graphite Electrode with Ultrahigh Capacitance for Energy Storage Applications. ChemElectroChem 5, 1501–1508. doi:10.1002/celc.201800143
Ţucureanu, V., Matei, A., Avram, A. M., Ţucureanu, V., Matei, A., Marius, A., et al. (2016). FTIR Spectroscopy for Carbon Family Study. Crit. Rev. Anal. Chem. 46, 502–520. doi:10.1080/10408347.2016.1157013
Umukoro, E. H., Kumar, N., Ngila, J. C., and Arotiba, O. A. (2018). Expanded Graphite Supported P-N MoS2-SnO2 Heterojunction Nanocomposite Electrode for Enhanced Photo-Electrocatalytic Degradation of a Pharmaceutical Pollutant. J. Electroanalytical Chem. 827, 193–203. doi:10.1016/j.jelechem.2018.09.027
Umukoro, E. H., Madyibi, S. S., Peleyeju, M. G., Tshwenya, L., Viljoen, E. H., Ngila, J. C., et al. (2017). Photocatalytic Application of Pd-ZnO-Exfoliated Graphite Nanocomposite for the Enhanced Removal of Acid orange 7 Dye in Water. Solid State. Sci. 74, 118–124. doi:10.1016/j.solidstatesciences.2017.11.001
Umukoro, E. H., Peleyeju, M. G., Ngila, J. C., and Arotiba, O. A. (2017). Towards Wastewater Treatment: Photo-Assisted Electrochemical Degradation of 2-nitrophenol and orange II Dye at a Tungsten Trioxide-Exfoliated Graphite Composite Electrode. Chem. Eng. J. 317, 290–301. doi:10.1016/j.cej.2017.02.084
Van Pham, T., Van Tran, T., Duy Nguyen, T., Thi Hong Tham, N., Thanh Tri Quang, P., Thi To Uyen, D., et al. (2019). Adsorption Behavior of Congo Red Dye from Aqueous Solutions onto Exfoliated Graphite as an Adsorbent: Kinetic and Isotherm Studies. Mater. Today Proc. 18, 4449–4457. doi:10.1016/j.matpr.2019.07.414
Velmurugan, M., Sakthinathan, S., Chen, S. M., and Karuppiah, C. (2015). Direct Electron Transfer of Glucose Oxidase and Electrocatalysis of Glucose Based on Gold Nanoparticles/electroactivated Graphite Nanocomposite. Int. J. Electrochem. Sci. 10, 6663–6671.
Wang, C., Hao, Z., Wei, Z., Bai, L., Yao, Z., Xu, H., et al. (2019). A Simple Method to Improve the Adsorption Properties of Drinking Water Treatment Residue by Lanthanum Modification. Chemosphere 221, 750–757. doi:10.1016/j.chemosphere.2019.01.099
Wei, D., Grande, L., Chundi, V., White, R., Bower, C., Andrew, P., et al. (2012). Graphene from Electrochemical Exfoliation and its Direct Applications in Enhanced Energy Storage Devices. Chem. Commun. 48, 1239–1241. doi:10.1039/c2cc16859f
Winter, S. S., and Woock, R. R. (1965). At State University of New York at Buffalo. J. Res. Sci. Teach. 3, 130–135. doi:10.1002/tea.3660030210
Wu, Y., Wu, Y., Lv, X., Lei, W., Ding, Y., Chen, C., et al. (2020). A Sensitive Sensing Platform for Acetaminophen Based on Palladium and Multi-Walled Carbon Nanotube Composites and Electrochemical Detection Mechanism. Mater. Chem. Phys. 239, 121977–121978. doi:10.1016/j.matchemphys.2019.121977
Xia, Y.-G., Li, X., Yu, L.-S., Liang, J., Sun, H.-M., and Kuang, H.-X. (2020). Structural-fingerprinting of Polysaccharides to Discern Panax Species by Means of Gas-Liquid Chromatography and Mass Spectrometry. Int. J. Biol. Macromolecules 151, 932–943. doi:10.1016/j.ijbiomac.2020.02.194
Yamuna, A., Sundaresan, P., and Chen, S.-M. (2020). Sonochemical Preparation of Bismuth Oxide Nanotiles Decorated Exfoliated Graphite for the Electrochemical Detection of Imipramine. Ultrason. Sonochem. 64, 105014–105018. doi:10.1016/j.ultsonch.2020.105014
Yang, Y.-j., Liu, E.-H., Li, L.-m., Huang, Z.-z., Shen, H.-j., and Xiang, X.-x. (2009). Nanostructured MnO2/exfoliated Graphite Composite Electrode as Supercapacitors. J. Alloys Comp. 487, 564–567. doi:10.1016/j.jallcom.2009.08.008
Zgagacz, W., Zakrzewski, R., Urbaniak, K., Chwatko, G., and Nowicki, A. (2020). The Use of High-Performance Liquid Chromatography with Diode Array Detector for the Determination of Sulfide Ions in Human Urine Samples Using Pyrylium Salts. J. Chromatogr. B 1157, 122309. doi:10.1016/j.jchromb.2020.122309
Zhai, J., Jiumu, L., Cong, L., Wu, Y., Dai, L., Zhang, Z., et al. (2020). Reed Decomposition under Bacillus Subtilis Addition Conditions and the Influence on Water Quality. Ecohydrology & Hydrobiology 20, 504–512. doi:10.1016/j.ecohyd.2019.11.003
Zhao, Q., Cheng, X., Wu, J., and Yu, X. (2014). Sulfur-free Exfoliated Graphite with Large Exfoliated Volume: Preparation, Characterization and its Adsorption Performance. J. Ind. Eng. Chem. 20, 4028–4032. doi:10.1016/j.jiec.2014.01.002
Keywords: biomolecules, biosensor, electrode, exfoliated graphite, sensor
Citation: Idris AO, Orimolade BO, Potlako M, Feleni U, Nkambule TTI and Mamba BB (2022) Exfoliated Graphite: A Surface Renewed Electrode for Environmental Applications. Front. Sens. 3:861965. doi: 10.3389/fsens.2022.861965
Received: 25 January 2022; Accepted: 21 March 2022;
Published: 19 April 2022.
Edited by:
Nomso Charmaine Hintsho-Mbita, University of Limpopo, South AfricaReviewed by:
Annamalai Senthil Kumar, VIT University, IndiaChelladurai Karuppiah, Ming Chi University of Technology, Taiwan
Anatoly Nikolaevich Reshetilov, Institute of Biochemistry and Physiology of Microorganisms (RAS), Russia
Copyright © 2022 Idris, Orimolade, Potlako, Feleni, Nkambule and Mamba. This is an open-access article distributed under the terms of the Creative Commons Attribution License (CC BY). The use, distribution or reproduction in other forums is permitted, provided the original author(s) and the copyright owner(s) are credited and that the original publication in this journal is cited, in accordance with accepted academic practice. No use, distribution or reproduction is permitted which does not comply with these terms.
*Correspondence: Azeez Olayiwola Idris, aWRyaXNhbG9uZTRyZWFsQGdtYWlsLmNvbQ==