- 1Center for Evaluation of Environmental Impact on Human Health (TOXICAM), Experimental Research Unit (UNIPEX), Department of Pathology, Botucatu Medical School, São Paulo State University (UNESP), Botucatu, São Paulo, Brazil
- 2Center for Evaluation of Environmental Impact on Human Health (TOXICAM), Experimental Research Unit (UNIPEX), Department of Bioprocess and Biotechnology, Botucatu School of Agriculture, São Paulo State University (UNESP), Botucatu, São Paulo, Brazil
- 3Center for Evaluation of Environmental Impact on Human Health (TOXICAM), Experimental Research Unit (UNIPEX), Department of Biotechnology, Institute of Biosciences, Botucatu, São Paulo, Brazil
Introduction: Diuron (3-(3,4-dichlorophenyl)-1,1-dimethylurea) is a prominent herbicide in Brazilian agriculture, particularly in sugarcane farming. This herbicide persists in the environment and is transformed into potentially toxic metabolites, such as 3,4-dichloroaniline (DCA) and 3-(3,4-dichlorophenyl)-1-methylurea (DCPMU), raising concerns that encompass environmental contamination and One Health, which unites environmental and human health. Zebrafish are an appropriate experimental model to evaluate toxicity: they metabolically and genetically resemble humans and can be applied in ecotoxicology.
Methods: In this study, we have employed multiparameter evaluation to characterize the toxicity of environmentally relevant concentrations (0.5–100 μM) of Diuron, DCA, or DCPMU in Brazil, a developing country. In the early stages of zebrafish development, we performed the fish embryo toxicity test according to the guidelines established in OECD Protocol No. 236, extended to 144 h post fertilization (hpf). We also investigated the oxygen consumption rate in zebrafish embryos at 24 hpf, evaluated the acetylcholinesterase (AChE) enzymatic activity in zebrafish larvae at 96 hpf, and used the Comet Assay to assess DNA fragmentation in zebrafish larvae at 96 hpf. We subjected adult zebrafish to acute exposure for 96 h in accordance with OECD protocol no. 203 for the Acute Toxicity Test in Fish. Following this exposure, we analyzed mutagenicity by using the micronucleus test, which was complemented by quantification of nuclear anomalies (NA) in erythrocytes and further confirmed by histopathological investigations.
Results and discussion: Exposure to any of the tested Diuron concentrations damaged DNA, and NA became significant beyond 10 μM Diuron. At 10 μM, DCA altered AChE activity, suggesting neuromuscular toxicity. Between 0.5 and 5 μM, DCA was potentially genotoxic, and 5–10 μM DCA induced nuclear pleomorphism. At all the tested concentrations, DCPMU damaged DNA, and NA emerged between 1 and 10 μM DCPMU. Adult zebrafish exposed to any of the tested Diuron, DCA, or DCPMU concentrations for 96 h did not exhibit significant histopathological alterations. Erythrocyte morphology analysis did not reveal mutagenicity. Although we observed non-mutagenic genotoxicity, chromosomal instability is of concern. The findings of this study contribute to our understanding of how Diuron and its metabolites affect zebrafish and highlights the need for multiparametric assessment to elucidate how herbicides and their metabolites impact the environment and human health. This endeavor informs regulatory measures for safely using Diuron and similar herbicides and reveals substantial implications for developing nations grappling with escalating water contamination due to pesticide use.
1 Introduction
Pesticides are employed in agricultural production to manage, prevent, and eliminate pests, thereby increasing quality and productivity (Atwood and Paisley-Jones, 2017). Brazil, a developing country that relies heavily on agricultural exports, is a major consumer of pesticides, accounting for 20% of the global market (equivalent to approximately US$ 10 billion per year (Barizon et al., 2020).
Diuron, a systemic herbicide belonging to the phenylurea group, is commonly used in large sugarcane, soybean, corn, and eucalyptus plantations and various fruit crops such as bananas, pineapples, and grapes in both the pre-emergent and post-emergent phases. This herbicide is also applied in urban areas (EFSA, 2005). Diuron acts by inhibiting water photolysis, thereby preventing photosynthesis (Field et al., 2003) and regulating weed growth. However, its worldwide application has environmental consequences due to its moderate to high permanence in sediments, soils, and surface waters. Indeed, Diuron has been identified in numerous environmental compartments, particularly aquatic environments, which has raised concerns about potential human and animal exposure to this herbicide by several means, including water and food consumption and occupational activities (Hernández et al., 2013).
Diuron has consistently been detected in emerging countries. In South Korea, 0.1501–5.831 nM Diuron has been detected in marine areas and waters (Kim et al., 2014). In Australia, up to 0.3 nM Diuron has been detected in the surface waters of Queensland (Ensminger et al., 2013), and concentrations exceeding 184.48 nM Diuron have been verified in the Great Barrier Reef (Skerratt et al., 2023). Up to 1.6 nM Diuron has been found in the Beijing and Tianjin regions in China (Kong et al., 2016).
Contamination with Diuron has also been detected in several Brazilian regions. In 2019, Companhia Ambiental do Estado de São Paulo (CETESB) (Environmental Company of the state of São Paulo) detected Diuron in 91.9% of surface and groundwater samples in the state of São Paulo, with the highest concentrations reaching 0.32 μg/L (1.37 nM) (CETESB, 2021). In studies conducted in the state of Rio Grande do Sul, up to 3.39 nM Diuron has been detected in surface water, and a maximum of 6.2 nM Diuron has been measured in drinking water (Caldas et al., 2019). In São Luis, Maranhão, Diuron at concentrations ranging from <0.1288 to 2.87 nM have been observed in groundwater (Viana et al., 2019). Diuron at 0.00214 nM has been detected in the Aquidauana River, Mato Grosso do Sul (Finoto Viana et al., 2023), and up to 4.29 nM Diuron has been observed in the Piracicaba, Capivari, and Jundiai rivers, São Paulo (Madeira et al., 2023). Diuron at 30.53 μM has been found in groundwater in the region of Ribeirão Preto, São Paulo (Di Bernardo Dantas et al., 2011).
The Instituto Brasileiro do Meio Ambiente e dos Recursos Naturais Renováveis (IBAMA) (Brazilian Institute of the Environment and Renewable Natural Resources) classifies Diuron as a Class II product, indicating that it is highly dangerous for the environment (IBAMA, 2021). Furthermore, according to the Global Harmonized Classification System (GHS), Diuron is classified as a class III herbicide, which means it is dangerous to the environment (IBAMA, 2021). In the context of drinking water supply, the Brazilian Ministry of Health establishes maximum Diuron concentration of 90 μg/L (0.361 μM), in accordance with Regulation 2.914/2011 (Brasil Ministry of Health, 2011).
Nevertheless, Diuron concentrations detected in aquatic environments may not reflect the true problem of its environmental persistence. The half-life of Diuron in aquatic environments is generally around 2 weeks to 1 month (Huovinen et al., 2015). Diuron biodegradation yields metabolites, including 3,4-dichlorophenylmethylurea (DCPMU) and 3,4-dichloroaniline (DCA). DCPMU and DCA are considered environmental pollutants because they persist in soil and water (Field et al., 2003). It has been estimated that DCA and DCPMU remain in aquatic systems for more than 36 days (Gooddy et al., 2002; Carena et al., 2018). However, photodegradation is one of the processes that influences the decomposition of these metabolites and is often limited by factors such as turbidity and the presence of other organic materials that can shield the compound from light (Carena et al., 2018).
These metabolites have been found in the Piracicaba, Capivari, and Jundiai rivers at concentrations limited to 1.419 μM (Madeira et al., 2023). Its main degradation product is DCA, a xenobiotic that is the tested of decontamination studies (Quirantes et al., 2017). Additionally, DCA is toxic when it is ingested or inhaled or when it comes into contact with the skin (PubChem, 2022). In turn, DCPMU has rarely been studied in classical in vivo and in vitro models.
Toxicological characterization of Diuron in experimental models contributes with knowledge about this herbicide and its degradation products, such as DCA and DCPMU. In a more realistic context, the aquatic environment is the first destination of these compounds, so alternative models like fish are relevant for analyzing toxicity (Khabib et al., 2022). According to recent studies, exposure to 1.287–12.87 nM Diuron causes aneuploidy and alters hemocyte parameters, such as Reactive Oxygen Species in adult oysters (Crossostrea gigas) (Bouilly et al., 2007). Embryotoxic and genotoxic effects of this herbicide and its metabolites have been verified in oyster larvae at 6 h post fertilization (hpf) (Behrens et al., 2016). An experiment carried out at 96 hpf in fish (Clarias gariepinus) exposed to Diuron (0.077–0.025 M) showed mutagenicity, biochemical changes in the blood, and liver dysfunction (Michael, 2018). Exposure of Nile tilapia (Oreochromis niloticus) to Diuron and its metabolites DCA, DCPU, and DCPMU (acute semi-static exposure, 40–200 ng/L) has been shown to impair antioxidant activity and biotransformation as evidenced by oxidative stress and lipid peroxidation markers (Felício et al., 2018).
When it comes to assessing the toxicity of herbicides, their active ingredients, and their metabolites, the alternative model zebrafish (Danio rerio) stands out (Gonçalves et al., 2020). First, zebrafish develop fast and reproduce at a high rate, being an interesting approach for toxicological tests. Furthermore, given the 71% genomic similarity between zebrafish and humans, endpoints that are relevant for the human health can be evaluated (Tal et al., 2020). In accordance with the ethical principles of the “3 Rs”, recognized by regulators worldwide, and support by the Brazilian legislation, zebrafish are a valuable tool to assess the hazard of compounds released into the environment (Russel and Burch, 1959; Brasil, 2022). In addition, zebrafish and humans have similar and conserved metabolic, toxicokinetic, and toxicodynamic profiles (de Souza Anselmo et al., 2018). Besides that, zebrafish are easy to maintain, small, and can be used to evaluate toxicity through different exposure pathways, which makes them a highly effective and ethically sound in vivo model for toxicological research (Nagel, 2002). Using them in research provides results that help to understand how diverse substances impact the environment and human health (Zinsstag et al., 2011).
Here, we aimed to assess the general toxicity of Diuron and its metabolites DCA and DCPMU in different stages of zebrafish (Danio rerio) development. We want to provide further information for need for a multi-parametric evaluation to strengthen regulatory protocols for safely applying this herbicide not only in Brazil, but also in comparable developing countries that are experiencing increasing water contamination with Diuron.
2 Materials and methods
The study methodology focused on the toxicological characterization of Diuron, DCA, and DCPMU by using multiple endpoints at different stages of zebrafish development as shown in Figure 1.
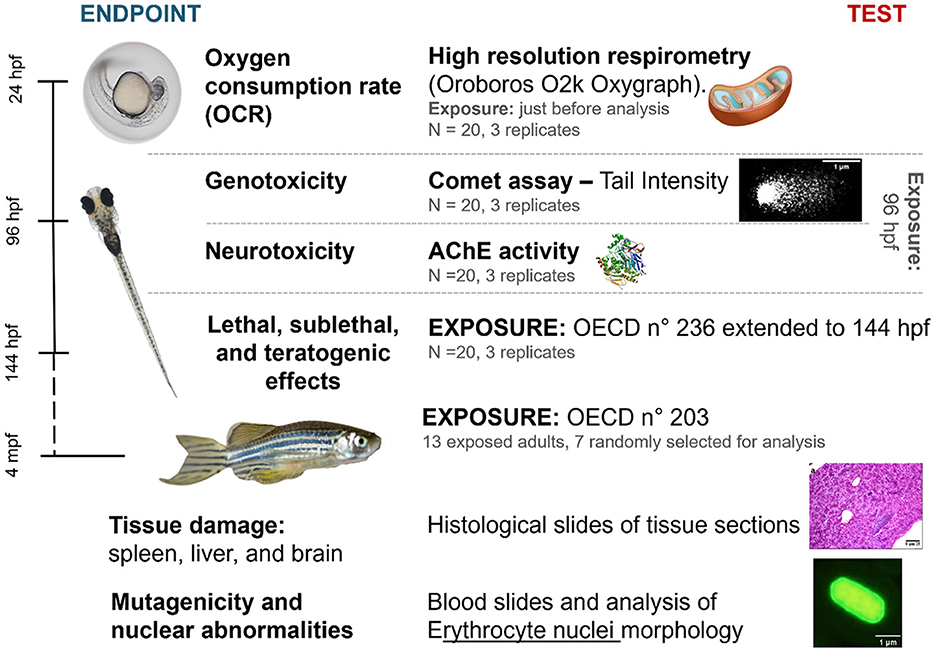
Figure 1. Diagram summarizing the methodology used in our study, indicating the performed analyses, stage of zebrafish development, time of exposure to one of the tested compounds, and number of samples. hpf, hours post-fertilization; mpf, months post-fertilization. Prepared by the authors.
2.1 Chemicals
The herbicide Diuron (CAS no. 330-54-1, purity ≥ 98%, catalog no. D2425, Sigma-Aldrich Co, St Louis, MO) and its metabolites DCA (CAS no. 95-76-1, purity ≥ 98%, catalog no. 437778, Sigma-Aldrich Co., St Louis, MO) and DCPMU (CAS no. 3567-62-2, purity ≥ 98%, catalog no. S613053, Sigma-Aldrich Co., St Louis, MO) were used in standard solutions. Concentrations from 0.5 to 100 μM were analyzed because they correspond to environmental concentrations according to Field et al. (2003), including the concentrations of up to 30.53 μM found in groundwater in Brazil (Di Bernardo Dantas et al., 2011), and contribute to understanding the toxic effects of these compounds on the basis of concentrations previously employed by our study group (Da Rocha et al., 2013; Lima et al., 2022a,b). Dimethyl sulfoxide (DMSO) and Tween® 20 (Sigma-Aldrich Co., St Louis, MO) were used to prepare stock solutions of Diuron and its metabolites at nominal concentrations. The negative control was ISO-7346 water, and the solvent control was 0.01% DMSO and Tween® 20 in ISO-7346 water. Previous tests showed that 0.01% DMSO and Tween® 20 did not have any toxic effects on zebrafish. For the fish embryo toxicity (FET) test, a 2.0 μM hydrogen peroxide (H2O2) solution was used as positive control because it affects development, causing delayed hatching, edema, and suspected liver damage (Li et al., 2021). For the oxygen consumption rate (OCR) test, potassium cyanide (KCN) diluted in sodium hydroxide (NaOH) was employed as positive control because KCN inhibits cytochrome c oxidase, acting as a mitochondrial uncoupler and halting mitochondrial respiration (Cammer, 1982).
2.2 Fish embryo toxicity test
2.2.1 Fish maintenance
Wild-type adult zebrafish (Danio rerio) (strain AB), which belong to the class Actinopterygii, order Cypriniformes, family Cuprinidae, and subfamily Danioninae, were employed as model organism. Zebrafish size ranged from 1.8 to 4.0 cm, and the zebrafish were sourced from the vivarium at the Botucatu Medical School and raised under controlled conditions in a recirculating aquaculture system. Male and female zebrafish were kept in 6 L glass aquaria (15 fish in each aquarium) under controlled conditions [temperature = 27 ± 1°C, pH = 7.5 ± 0.5, dissolved oxygen at 95% saturation, conductivity = 750 ± 50 μs/cm, and 14/10 (light/dark) photoperiod] (Westerfield, 2000). The zebrafish were fed with Maramar® Tropical Granules, Alcon® Spirulina Feed, and Maramar® high hatching Artemia Eggs three times a day.
To obtain eggs, the male and female zebrafish at a 2:1 ratio were separated in the spawning tank until the following morning, when the separation was removed. Then, light stimulus promoted mating. Fertilized zebrafish eggs at ≤ 3 hpf were selected with the aid of an inverted microscope (AE2000, Motic®, Barcelona, Spain).
2.2.2 Experimental design
The study was based on OECD No. 236 (OECD, 2013) guidelines and was conducted at the Zebrafish Animal Laboratory located at UNIPEX—Experimental Research Unit of the Botucatu Medical School, São Paulo State University (UNESP), Brazil. The Ethics Committee on Animal Use approved the project (Certificate No. 1304/2019). Each Diuron, DCA, or DCPMU concentration was tested on 20 zebrafish embryos, which were distributed into sterile 24-well plates and exposed to autoclaved water obtained from a zebrafish flow-through system as internal control. The FET test was performed in independent triplicates. Zebrafish embryos or larvae exposed to one of the tested compounds were monitored and evaluated under an inverted microscope (Motic®, AE2000) at 8, 24, 48, 96, 120, and 144 hpf; OECD TG236 protocol was followed with an extension until 144 hpf. The exposed zebrafish embryos and larvae were compared to the description of typical zebrafish development by Kimmel et al. (1995), which includes endpoints such as mortality, sublethality, and teratogenicity. Images of embryos and larvae were captured at various times after fertilization by using a digital camera (Olympus MVX10) attached to a stereomicroscope (SMZ800, Nikon). The subsequent tests with embryos and larvae were conducted at concentrations ranging from 0.5 to 5 μM, following the analysis of mortality in the FET test.
2.2.3 Oxygen consumption rate test
At 24 hpf, the zebrafish embryos exposed to one of the tested compounds were subjected to an oxygen consumption rate (OCR) test. The Oroboros 02k Oxygraph (Oroboros Instruments, Austria) was used, and the protocol adapted from Gnaiger's (2020) research was followed. The test was conducted at 27°C and 500 rpm. After the oxygen consumption curve was stabilized and calibrated with 1 mL of MAS-1 mitochondrial respiration medium in the cuvettes, 20 embryos were inserted in each cuvette. After incubation for 3 min, substrates that activate the Krebs cycle and electron transport chain (ETC) were added including succinate (2 M), malate (2 M), pyruvate (2 M), and ADP (1 mM), followed by 35 mM digitonin (catalog No. 300410, Sigma-Aldrich Co.), to permeabilize the chorion and biological membranes. The negative control, the positive control [KCN (CAS No. 151-50-8, catalog No. 60178, Sigma-Aldrich Co.), 0.4 mol/L diluted in NaOH, pH > 10], Diuron, DCA, or DCPMU at 0.5, 1, or 5 μM was added. Assays were performed in triplicate for each experimental condition. Basal respiration was analyzed after 5 min. To inhibit ATP synthase and to enable respiration without phosphorylation, 45 mM oligomycin (catalog No. 495455, Sigma-Aldrich Co.) was added. This was followed by addition of 50 mM FCCP (carbonyl cyanide p-trifluoromethoxy phenylhydrazone, no TIP2k, catalog No. C2920, Sigma-Aldrich Co, purity ≥ 98%), a mitochondrial uncoupler that depolarizes mitochondrial membranes, increases the OCR, and allows uncoupled respiration to be analyzed. Finally, for residual consumption, 25 mM rotenone (catalog no. R8875, Sigma-Aldrich Co.) and antimycin A (catalog No. A8674, Sigma-Aldrich Co.) were added because they inhibit complexes I and II and stop respiration. The OCR was measured at stabilized 3-min intervals. The residual consumption after addition of rotenone and antimycin A was subtracted to obtain a specific value for mitochondrial respiration.
2.2.4 Acetylcholinesterase activity assay
At the end of each exposure assay, a pool of 30 larvae at 96 hpf per experimental condition was used to measure AChE activity. The enzyme was quantified using the Bradford method (Bradford, 1976), adapted for a 96-well plate. The AChE activity was determined by using the method of Ellman et al. (1961), adapted to a 96-well plate by Guilhermino et al. (1998), with acetylthiocholine as substrate. Conjugation of 5,5′-ditiobis (2-nitrobenzoic acid) (DTNB) (catalog no. 22582, Sigma-Aldrich Co) with 2-(dimethylamino) ethanethiol hydrochloride (thiocholine) (catalog no. D2425, Sigma-Aldrich Co) was monitored by measuring the increase in absorbance at 414 nm for 5 min. AChE activity is expressed as nmol of hydrolyzed substrate per minute per mg of protein (ε = 13.6 × 103 M −1 cm−1).
2.2.5 Comet assay
The protocol of Abe et al. (2024) was used to accomplish the comet assay at 96 hpf with zebrafish larvae exposed to one of the tested compounds. Twenty selected embryos were exposed to each experimental condition using six-well culture plates. The experimental conditions included three concentrations of each tested compound (0.5, 1, or 5 μM), a positive control [0.001% H2O2 (v/v), CAS no. 7722-84-1, catalog no. 1.08600, Sigma-Aldrich Co., St Louis, MO, 29-32%], and a negative control (ISO-7346 water and 0.01% v/v DMSO and Tween 20). Three independent replicates were conducted. After static exposure for 96 h, the larvae were euthanized and resuspended in 100 μL of PBS buffer. The next step involved maceration to isolate cells. Cell viability was assessed by using the Trypan Blue method after filtration in a cell strainer and centrifugation. Samples with a viability equal to or >80% were accepted. Then, 50 μL of each cell suspension was added to 150 μL of a 1% LMP agarose (Low Melting Point, catalog no. 16520050, Invitrogen, USA) solution at 27°C. The mixture was homogenized and distributed on pre-gelatinized slides containing 1.5% NMP agarose (Normal Melting Point, UltraPure™ Agarose, catalog no. 18300012, Invitrogen, USA). The process involved gelatinization followed by lysis in a solution containing 2.5 M NaCl, 10 mM Tris, 100 mM EDTA, 1%Triton X-100 (v/v), and 10% DMSO (v/v) at pH = 10. Electrophoresis was carried out in a buffer containing 1 mM EDTA and 300 mM NaOH at 4°C and pH = 13 for 20 min. Slides were neutralized in 0.1 M Tris-HCl buffer. Cells were stained with 0.1% SYBR™ Gold Nucleic Acid Gel Stain (1:10,000, catalog no. S11494, Invitrogen, USA) (v/v) and analyzed under an epifluorescence microscope (Eclipse E200, Nikon, Japan). The intensity of the comet tail (Tail Intensity) was quantified by using the Comet IV software version 4.3 provided by Perceptive Instruments Ltd.
2.3 Fish acute toxicity test
2.3.1 Fish maintenance
Sexually mature zebrafish (~3 months old) were provided by the Laboratory of Applied Toxinology of the Butantan Institute (São Paulo/SP) and were maintained under controlled conditions in 9 L aquaria with reconstituted water standardized according to ISO 7346-1 and 7346-2 (2 mM CaCl2, 5 mM MgSO4, 0.75 mM NaHCO3, and 0.07 mM KCl) (ISO, 2007), at 27 ± 1°C and under a 14/10 (light/dark) photoperiod (Westerfield, 2000). Throughout the experiment, parameters such as pH, ammonia, and oxygen were also monitored and controlled. The zebrafish were fed with Maramar® Tropical Granules, Alcon® Spirulina Feed, and Maramar® high hatching Artemia Eggs three times a day. The experimental group consisted of adult zebrafish with average weight of 0.34 ± 0.04 g and average length of 3.01 ± 0.3 cm, acclimated for 1 month before the experiment started. The mortality rate during maintenance was <2%.
2.3.2 Experimental design
Thirteen adult zebrafish were used for each concentration of the tested compounds (Diuron, DCA, or DCPMU) or the solvent control (DMSO 0.01%). The fish were kept in 8 L glass aquaria. Exposure was semi-static, with 50% of water and the tested compound being replaced after exposure for 48 h. The protocol was recommended by OECD No. 203 (OECD, 2019), which required only one acute exposure for 96 h. The parameters temperature (°C), pH, dissolved oxygen (mg/L), and total hardness (mg/L) were monitored daily during exposure to assess water quality. After 96 h, seven adult zebrafish were randomly selected for analysis, in accordance with the minimum number of animals established by OECD No. 203 (OECD, 2019). The fish were euthanized with benzocaine hydrochloride (0.1%, 3 mg/L, catalog no. E1501, Sigma-Aldrich Co., St Louis, MO) previously dissolved in ethanol was added. The other six adult zebrafish were used in another experiment. The concentrations analyzed for histopathology and nuclear anomalies (NA) ranged from 0.5 to 100 μM, whereas DCA and DCPMU could be analyzed up to 50 μM due to the mortality observed.
2.3.3 Histological evaluation
The liver, brain, and spleen were removed, weighed, and immersed in 10% buffered formalin until histological processing. Histological sections with a thickness of 4 μm were stained with H&E and analyzed for alterations in the liver, brain, and spleen under a conventional optical microscope (Olympus Optical Co., Japan). The organs were qualitatively analyzed by histological analysis (Camargo and Martinez, 2007; Lakshmaiah, 2017). Alterations were quantified on a scale of 0 (normal) to 3 (severe). The alteration index was calculated by summing the intensity of the observed damages for each established parameter, multiplied by the level of importance of each parameter (Gibson-Corley et al., 2013).
2.3.4 Micronucleus and nuclear abnormality test
The caudal fins were removed for blood to be collected. Three blood smears per adult zebrafish were fixed in absolute methanol, placed on glass histological slides, and stained with acridine orange (5 mg/mL acridine orange, catalog no. A8097, Sigma-Aldrich Co., St Louis, MO) before being analyzed under a fluorescence microscope (Olympus BX53) (Çavaş, 2008). Observations were recorded in microphotographs by using the cellSens software (Olympus, Center Valley, PA, USA) and analyzed with Fiji-ImageJ (National Institutes of Health, Bethesda, Maryland, USA). Micronuclei in erythrocytes were identified by following specific criteria, including the presence of extranuclear bodies with spherical or ovoid shapes in the cytoplasm. Erythrocytes with NA were evaluated by the protocol described by Canedo et al. (2021). The frequency of NA, such as micronuclei, was determined by using the equation FAN = AN/N, where FAN represents the frequency of anomalies, and AN/N represents the ratio between the number of anomalies and the erythrocyte nuclei counted for each examined zebrafish (1,000 nuclei).
2.4 Statistical analyses
The data obtained from the tests, which included more than two unpaired groups, were submitted to a normality test (Shapiro-Wilks). For the non-parametric data, analysis of variance (Kruskal-Wallis), followed by Dunn's multiple comparison post-test was applied. If the data was parametric and normal, analysis of variance (One-way ANOVA), followed by Dunnet's post-test was used to determine the significance of the exposures compared to the negative control. Statistical significance of the data was set at p ≤ 0.05. The analyses were performed by using the GraphPad Prism 9.00 software (San Diego, CA 92108, USA, GraphPad Software, Inc).
3 Results
3.1 Embryo analysis
Mortality occurred from 8 hpf in zebrafish embryos exposed to 50 or 100 μM Diuron, DCA, or DCPMU (Figure 2). Significant mortality (p < 0.05) also occurred from 48 hpf at 10 μM Diuron, from 8 hpf at 5 or 10 μM DCA, and from 8 hpf at 10 μM DCPMU (Figure 2). Malformations consistent with pericardial edema, yolk sac edema, and skeletal deformities were observed in zebrafish larvae exposed to 10 μM DCA or DCPMU (Figure 3). Figure 4 shows microphotographs taken at 120 hpf of control zebrafish larvae and larvae exposed to Diuron, DCA, or DCPMU.
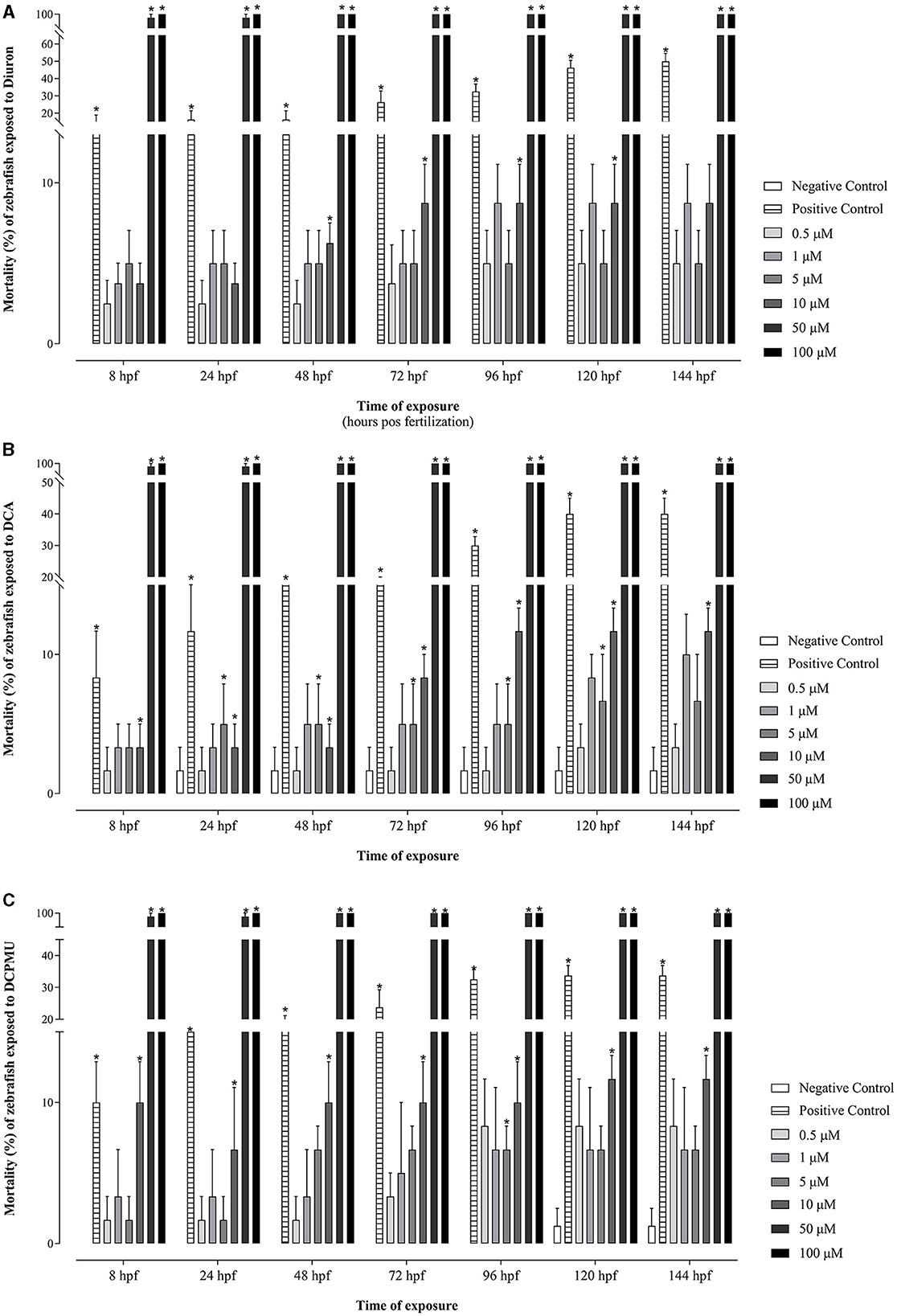
Figure 2. Mortality of zebrafish embryos and larvae, expressed by the percentage of clotted eggs and absence of heartbeats due to the concentration of and time of exposure to Diuron, 3,4-dichloroaniline (DCA), or 3-(3,4-dichlorophenyl)-1-methylurea (DCPMU). (A) Diuron, (B) DCA, and (C) DCPMU. Mortality was statistically significant (p < 0.05) compared to the negative control when represented by (*). The bar graphs represent the mean and standard deviation (n = 20 embryos per condition/three replications). Plotted with the GraphPad Prism 5.01 software.
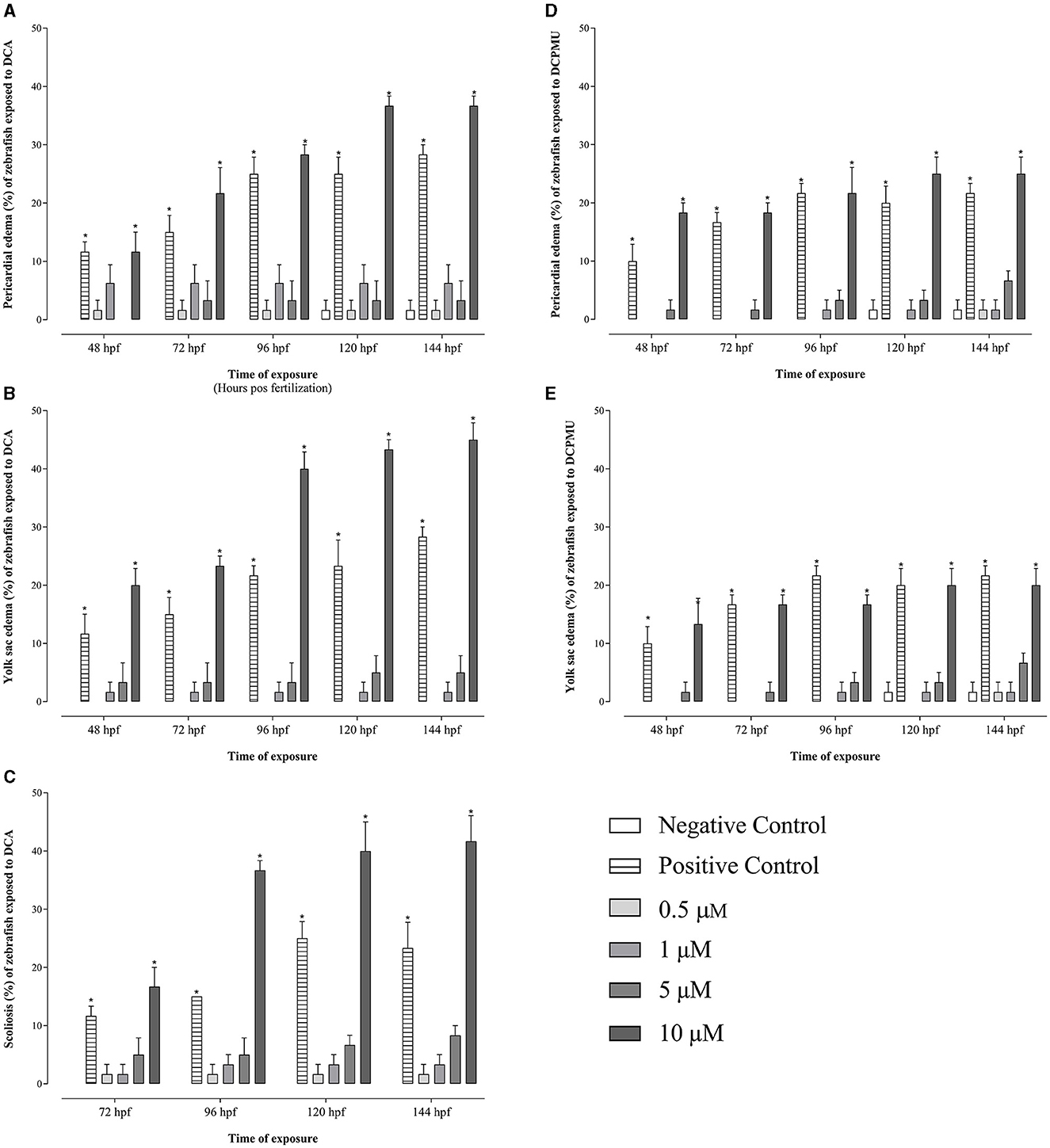
Figure 3. Malformations in zebrafish embryos and larvae according to the concentration and time exposure to 3,4-dichloroaniline (DCA), or 3-(3,4-dichlorophenyl)-1-methylurea (DCPMU). Malformations due to exposure to DCA included (A) pericardial edema, (B) yolk sac edema, and (C) scoliosis, while malformations due to exposure to DCPMU included (D) pericardial edema and (E) yolk sac edema. Malformations were statistically significant (p < 0.05) compared to the negative control when represented by (*). The bar graphs represent the mean and standard deviation (n = 20 embryos per condition/3 replications). Plotted with the GraphPad Prism 5.01 software.
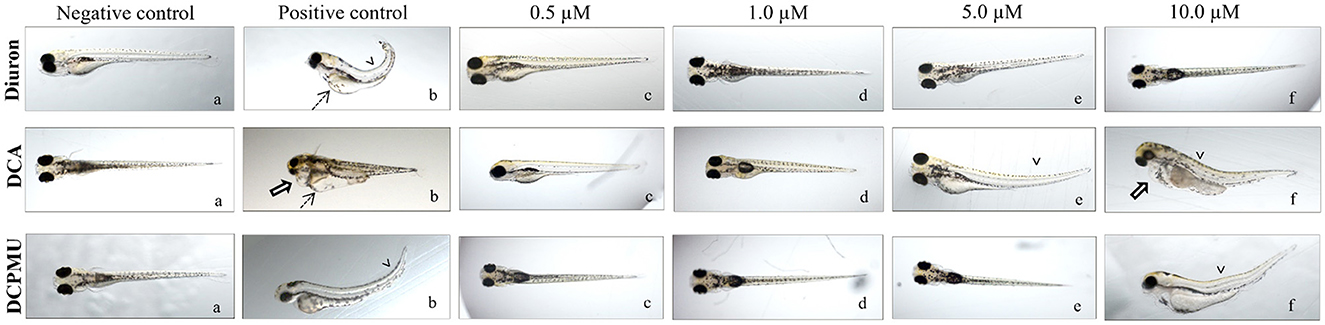
Figure 4. Representative micrographs of zebrafish larvae taken at 120 hpf after exposure to (a) negative control, (b) positive control, (c) 0.5 μM, (d) 1 μM, (e) 5 μM, and (f)10 μM Diuron, 3,4-dichloroaniline (DCA), or 3-(3,4-dichlorophenyl)-1-methylurea (DCPMU) (1.6x magnification for larvae under an Olympus MVX10 microscope). Legend for the verified changes: dashed arrows, yolk sac edema; open arrow, pericardial edema; and arrowheads, skeletal deformity. Micrographs are representative of three independent experiments.
Figure 5 displays the OCR at 24 hpf obtained for each phase of mitochondrial respiration. Compared to the negative control, the addition of different Diuron, DCA, or DCPMU did not significantly alter the OCR during basal respiration of zebrafish embryos; for the same tested compound, differences between the concentrations were not significant, either. However, the OCR during basal respiration tended to increase at 1 μM Diuron and all the DCA or DCPMU concentrations (0.5–5 μM). When we evaluated non-phosphorylating respiration with oligomycin, which inhibits ATP synthase (Rahn et al., 2013), there were no significant changes at the different Diuron, DCA, or DCPMU concentrations (0.5–5 μM) compared to the control. When FCCP is used for uncoupled respiration, it uncouples oxidative phosphorylation, causing the ETC to reach its maximum capacity (Obeidat et al., 2018). Exposure to the negative control, to the tested compounds, or to the various concentrations of the same tested compound did not elicit significantly different results.
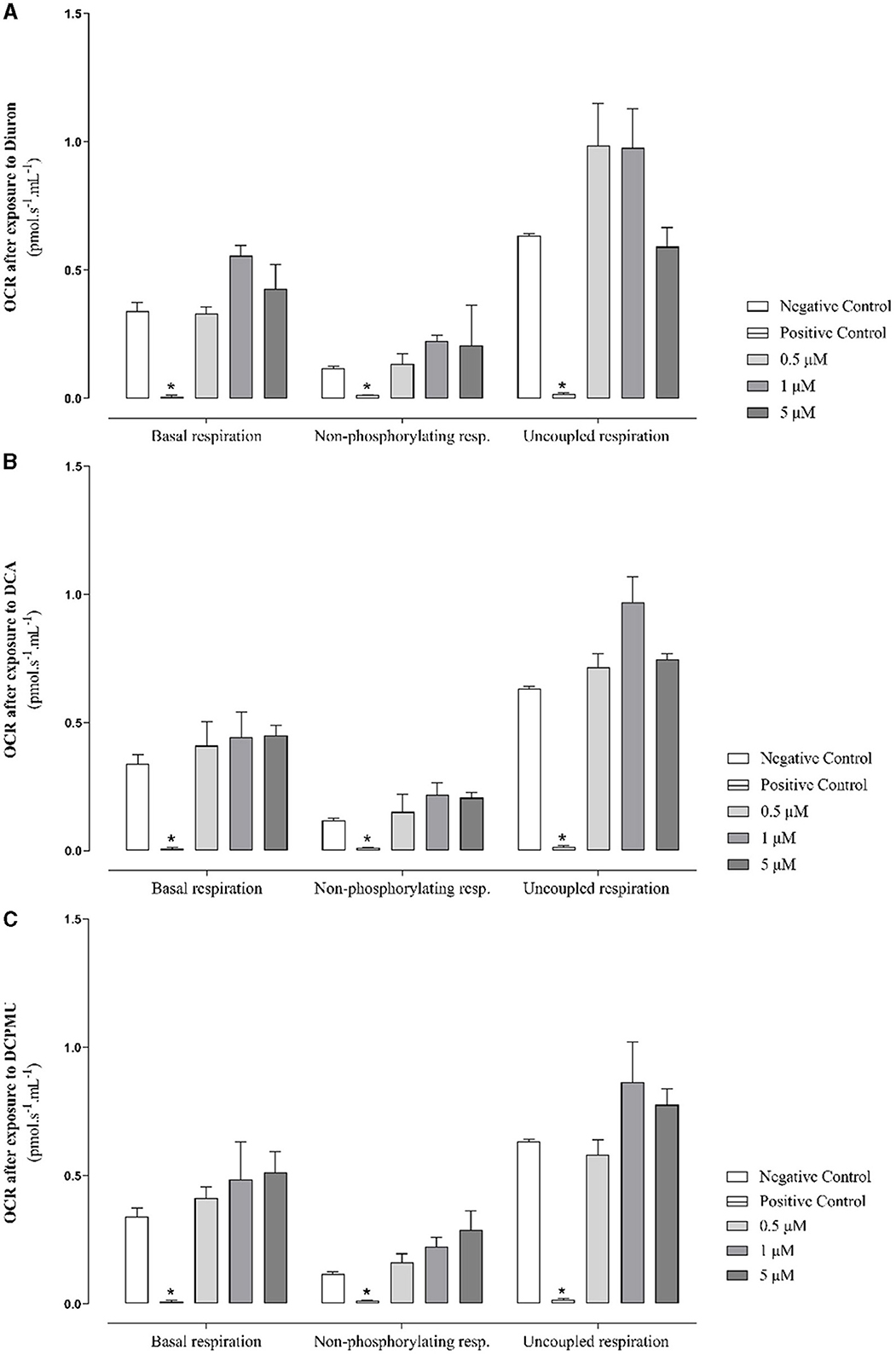
Figure 5. Representative graphs of the oxygen consumption rate (OCR) during mitochondrial respiration were generated at 24 h post-fertilization by adding mitochondrial modulators to zebrafish embryos exposed to different Diuron, 3,4-dichloroaniline (DCA), or 3-(3,4-dichlorophenyl)-1-methylurea (DCPMU) concentrations. The figures depict the OCR (pmol s−1 mL−1) obtained for each concentration and tested compound during respiration monitoring and include the negative and positive controls (10 mM KCN in NaOH pH > 10) (with mean and standard deviation values) for each phase. Basal respiration was measured by adding Diuron, DCA, or DCPMU (0.5–5 μM) followed by addition of 7.5 μM oligomycin (45 mM) to establish non-phosphorylating respiration. Next, 10 μM FCCP (50 mM) was added to measure uncoupled respiration. OCR results obtained at 24 hf for embryos exposed to (A) Diuron, (B) DCA, or (C) DCPMU are shown. The OCR was statistically significant (p ≤ 0.005) compared to the negative control when represented by (*). The bar graphs represent the mean and standard deviation (n = 20 embryos per condition/three replicates). Plotted with the GraphPad Prism 5.01 software.
Figure 6 presents the results of AChE enzyme activity analysis at 96 hpf after exposure to Diuron, DCA, or DCPMU. The AChE activity decreased in larvae exposed to 10 μM DCA compared to the control group.
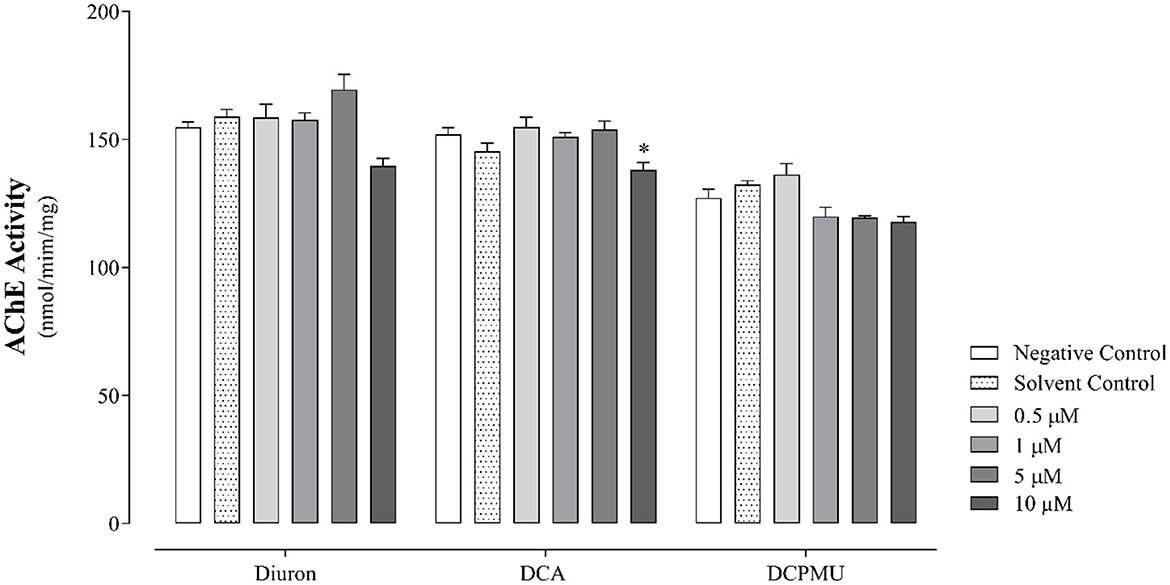
Figure 6. Acetylcholinesterase (AChE) enzyme activity at 96 hpf in cell pools obtained from larvae after exposure to different Diuron, 3,4-dichloroaniline (DCA), or 3-(3,4-dichlorophenyl)-1-methylurea (DCPMU) concentrations. The AChE activity was statistically significant (p < 0.05) compared to the negative control when represented by (*). The bar graphs represent the mean and standard deviation (n = 20 embryos per condition/three replicates). Plotted with the GraphPad Prism 5.01 software.
Regarding the genotoxicity analyses conducted during the larval stage (96 hpf), the cell suspensions obtained from maceration of the larvae had high cell viability (93 ± 6%). The results also indicated that exposure to the tested compounds damaged DNA. Indeed, the comet assay showed significantly increased comet tail intensity (p < 0.0001), indicating higher levels of damaged DNA at all the tested Diuron, DCA, or DCPMU concentrations (0.5–5 μM) compared to the negative control (Figure 7).
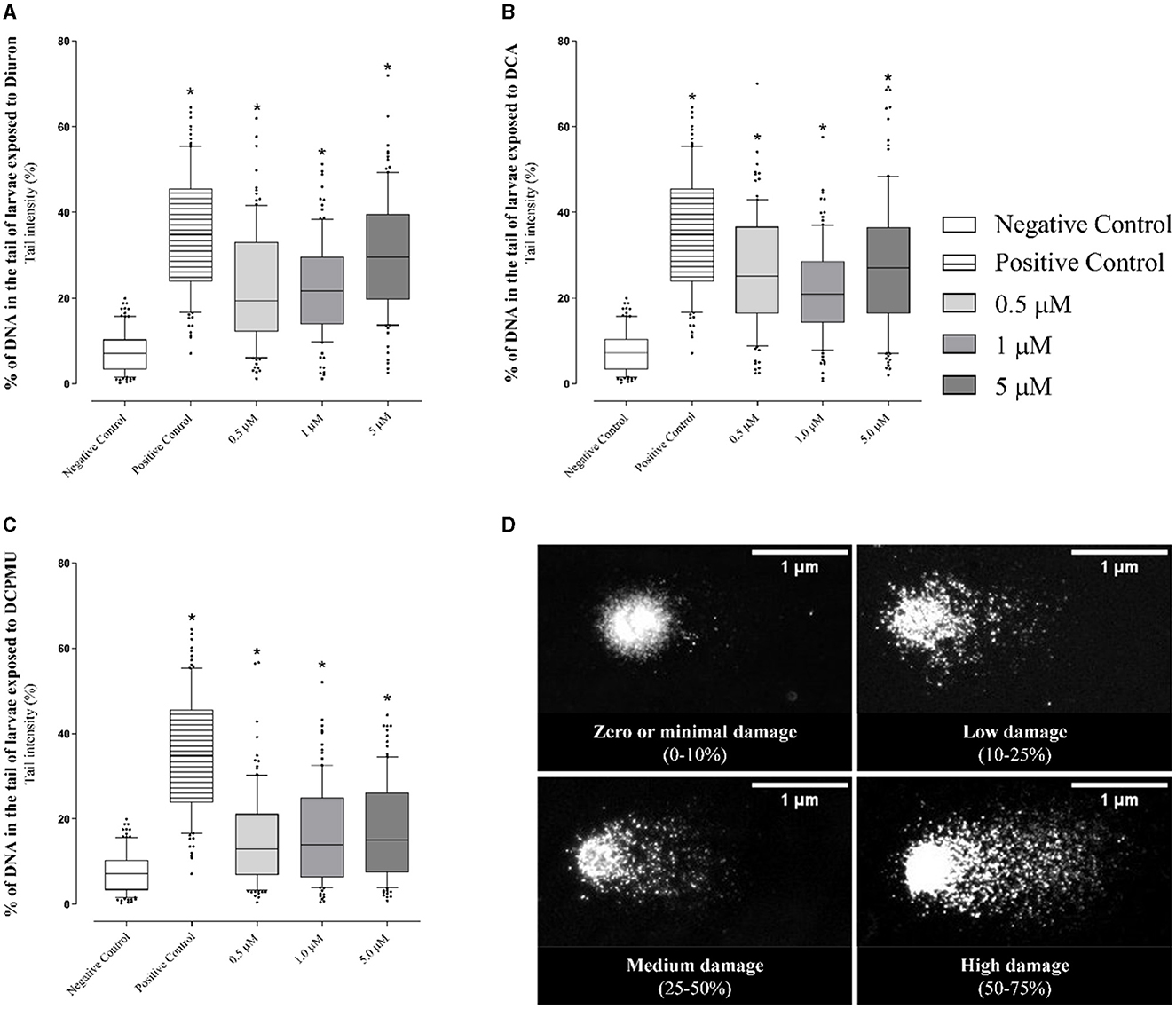
Figure 7. Representative graphs of comet tail intensity (%) in the analyses of cell pools obtained from larvae (96 hpf) after exposure to different concentrations of (A) Diuron, (B) DCA 3,4-dichloroaniline (DCA), or (C) 3-(3,4-dichlorophenyl)-1-methylurea (DCPMU). The graphs express the tail intensity (%) obtained at each concentration for each tested compound (boxplot with median and quartiles are indicated, percentile intervals 10–90). The intensity was significant (p ≤ 0.005) compared to the negative control when indicated by (*) (n = 20 larvae per condition/three replicates) (100 nucleoids per slide per condition). The Comet IV software (version 4.3, Perceptive Instruments Ltd) was employed to capture the microphotographs, which accurately reflect the intensity of the damage observed during exposure. Prepared with the GraphPad Prism 5.01 software. (D) Representative micrographs of the various levels of DNA damage observed in a pool of larvae (96 hpf) after exposure to Diuron, DCA, or DCPMU. The images indicate the DNA damage levels recorded during the experiment and were obtained by using the Comet IV software.
We classified the nucleoid tail intensities according to DNA fragmentation levels (Figure 7D), which ranged from little or no damage (0–10%) to severe damage (>75%). Supplementary Table 1 lists the results obtained for each concentration. The data indicated that all the exposures decreased the number of nucleotides with minimal or no damage (0–10%), suggesting that Diuron, DCA, and DCPMU fragmented DNA. At 1 μM Diuron or DCA, the percentage of damaged cells with low index was significant. Most cells exposed to 1 or 5 μM Diuron or 0.5–5 μM DCA exhibited medium damage (25–50%). High damage (50–75%) occurred at 0.5 or 5 μM Diuron or DCA.
3.2 Adult zebrafish analysis
Regarding acute exposure, all the adult zebrafish exposed to different Diuron concentrations survived, but the adult zebrafish exposed to 100 μM Diuron became apathetic. All the adult zebrafish exposed to the highest DCA or DCMPU concentrations (50 or 100 μM) died. Most of the adult zebrafish exposed to the lowest DCA or DCPMU concentrations (0.5, 1, 5, or 10 μM) survived (data not shown). As for the histological analyses at 96 hpf of the liver, spleen, and brain tissues removed from adult zebrafish exposed to Diuron, DCA, or DCPMU, they generally revealed normal morphology: tissues and cells had normal distribution, prominent nuclei, and outlined, intact, clear, dense, and well-preserved cytoplasm. However, the adult zebrafish exposed to 10 μM DCA or DCPMU had slightly structurally altered hepatocytes, specifically cytoplasmic vacuolation and hyaline droplets (Figure 8). Supplementary Table 2 summarizes the semi-qualitative score of the liver, spleen, and brain histology of adult zebrafish exposed to different Diuron, DCA, or DCPMU concentrations.
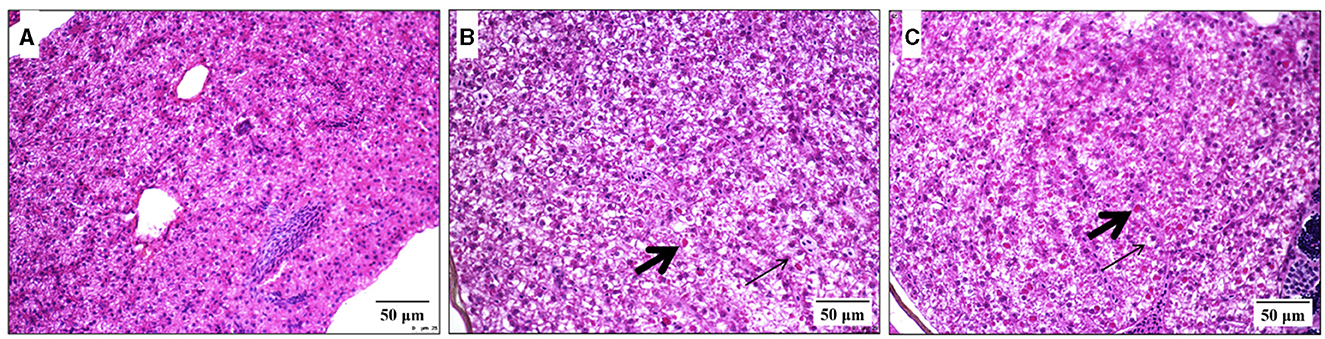
Figure 8. Histological micrographs showing the effects of exposure to 3,4-dichloroaniline (DCA), or 3-(3,4-dichlorophenyl)-1-methylurea (DCPMU) for 96 h on the liver of adult zebrafish (H&E, 25 μm). Liver sections exposed to (A) the negative control, (B) 10 μM DCA and (C) 10 μM DCPMU (40x on Olympus MVX10 microscope). Legend of the verified changes: thin arrows, vacuolization; thick arrows, hyaline droplets.
The frequency of micronuclei in adult zebrafish erythrocytes did not differ significantly across the exposures. Additionally, the frequency of micronuclei in exposed adult zebrafish erythrocytes did not exceed the naturally observed negative control levels of 0.5% (Canedo et al., 2021) (data not shown).
Compared to the control, we observed significant differences in the shapes of erythrocyte nuclei, which included lobulated, bilobed, blistered, notched, and kidney-shaped nuclei and nuclear constrictions (Figure 9) (refer to Supplementary Table 3). Diuron (5–100 μM), DCA (1 or 10 μM), or DCPMU (1–10 μM) exposure resulted in a significantly higher proportion of erythrocytes with blistered nuclei compared to the negative control, surpassing the baseline limit of 1.6% reported by Canedo et al. (2021). These authors found notable concentrations of specific compounds, including 50 μM Diuron, 10 μM DCA, and 1 μM DCPMU, all of which led to higher proportion of erythrocytes with blistered nuclei than the threshold of 1.1% (Canedo et al., 2021). In addition, 10 μM Diuron, 10 μM DCA, or 1–5 μM DCPMU showed a significantly higher proportion of erythrocytes with blisters or sprouts compared to the negative control, surpassing the threshold proportion of 0.7% of zebrafish erythrocytes (Canedo et al., 2021). The proportion of erythrocytes with bilobulated nuclei was only significant at 50 μM DCA or 1 μM DCPMU. Nuclear abnormalities (NA), such as nuclear constriction and kidney-shaped nuclei, emerged at 100 μM Diuron and 5–10 μM DCPMU, respectively. Figure 9A shows significantly increased percentage of nuclei exhibiting NA at 10–100 μM Diuron, indicating that Diuron at high concentrations impacted nuclear morphology. DCA at 10 μM or 1–10 μM DCPMU also provided noteworthy NA percentages.
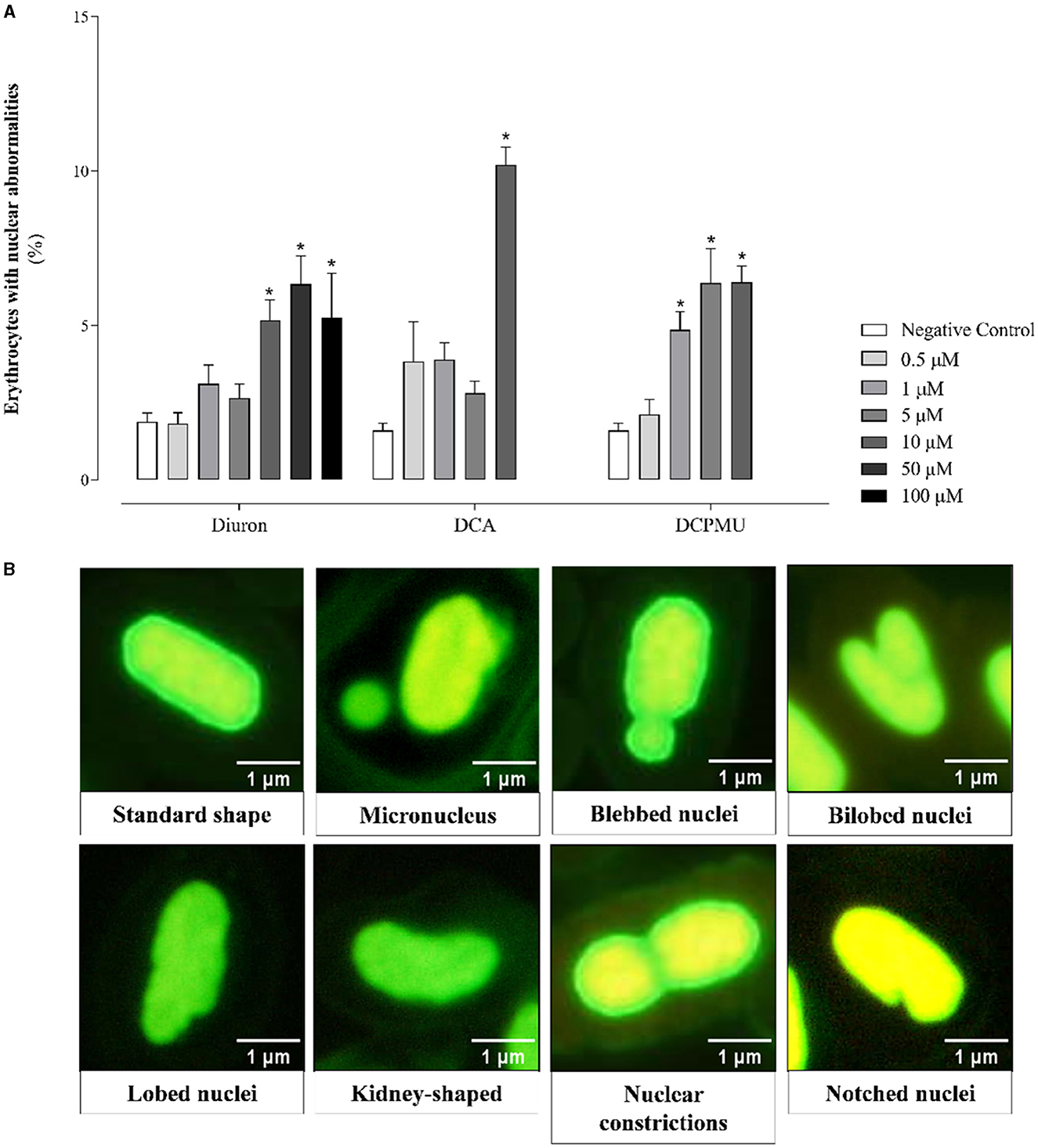
Figure 9. (A) Graph depicting the cumulative count of nuclear irregularities observed in red blood cells of adult zebrafish exposed to Diuron, 3,4-dichloroaniline (DCA), or 3-(3,4-dichlorophenyl)-1-methylurea (DCPMU) in a semi-static environment for 96 h. The graph displays the frequency (percentage) of nuclear abnormalities (NA) per 1,000 cells at each tested compound concentration. The frequency was notable (p ≤ 0.005) compared to the negative control when marked by (*). The bar graphs represent the mean and standard deviation (n = 7 fish per condition, 1,000 cells per fish). (B) Representative microphotographs describing the NA found in the erythrocytes of adult zebrafish exposed to Diuron, DCA, or DCPMU for 96 h. Prepared with the GraphPad Prism 5.01 software. (B) The micrographs are representative of the NA found after the exposures, 1,000 X magnification.
4 Discussion
We carried out toxicological characterization of the herbicide Diuron and its metabolites (DCA and DCPMU) by using zebrafish at different developmental stages (embryonic, larval, and adult) and identified some toxic effects of these compounds on the experimental model. We found that Diuron is less embryotoxic than its metabolites, that DCA affects AChE activity, and that high Diuron, DCA, or DCPMU concentrations cause mortality, DNA damage, and NA. Therefore, attention is required because these compounds are present in aquatic environments.
Here, all the zebrafish embryos exposed to 50 or 100 μM Diuron, DCA, or DCPMU died. Zaluski et al. (2022) exposed zebrafish embryos to 1 or 1.5 mg/L (4.29 or 6.43 μM) Diuron and verified malformations such as increased ocular distance at seven days post-fertilization (dpf). We did not observe alterations in embryos or larvae after exposure to 10 μM (2.33 mg/L) Diuron, the highest concentration at which zebrafish survived. However, different concentrations of Diuron residues have been found in the aquatic environment, which raises environmental concerns (Masiá et al., 2015).
Scheil et al. (2009) demonstrated edemas in zebrafish embryos and larvae resulting from exposure to 1 mg/L DCA for 96 h, which was close to the DCA concentrations studied herein. Zhu et al. (2013) evaluated DCA effects on the aquatic experimental model rare minnow (Gobiocypris rarus) in the embryonic and larval phase and detected malformations at 72 hpf at low DCA concentrations (1.426 mg/L or 8.8 μM and 0.187 mg/L or 1.15 μM, respectively). On the basis of these results, both models are sensitive to low concentrations of DCA. In the specific case of the zebrafish and DCA, published data vary. Voelker et al. (2007) reported LOEC of 2.01 mg/L DCA for survival and sublethal effects on 48 h zebrafish embryos. In turn, von Hellfeld et al. (2020) reported acute and sublethal toxicity data for DCA obtained from embryo tests in zebrafish as follows—EC10: 1,200 mg/L (0.007 M), EC50: 1,900 mg/L (0.012 M), LC10: 1,600 mg/L (0.01 M), and LC50: 2,400 mg/L (0.015 M). At 96 hpf, Schiwy et al. (2020) found LC50 of 2.7 mg/L (16.66 μM) for DCA. Such results highlight that it is important to study the real DCA toxicity in this experimental model given that here we identified malformations at 48 hpf after exposure to 10 μM (1.62 mg/L) DCA, while Scheil et al. (2009) detected malformations in zebrafish larvae exposed to 1 mg/L (6.17 μM).
After exposing zebrafish embryos to DCPMU, we verified malformations consistent with edema. Data on embryotoxicity in zebrafish exposed to DCPMU do not exist, and EC and LC values have not been established for this metabolite. However, DCPMU has been shown to be toxic to adult fish. For example, exposure to DCPMU has been shown to alter reproduction in Nile tilapia (Oreochromis niloticus) with estrogenic effects being potentially mediated through enhanced estradiol biosynthesis (Pereira et al., 2015). Altered biochemical parameters have also been demonstrated in tissues of Nile tilapia exposed to DCPMU, including decreased Glutathione S-transferase (GST) activity and oxidative stress and increased lipid peroxidation (LP), and an integrated analysis of biomarkers has pointed DCPMU as the compound underlying these changes (Felício et al., 2018).
Here, edemas were one of the main alterations in embryos and larvae exposed to 10 μM DCA or DCPMU. Edema has been suggested to be caused by kidney failure, circulatory failure, ionic imbalance, or permeability defects.
The OCR assay is fundamental for real-time analysis of the mitochondrial function. This assay allows basal respiration to be measured, the respiration phases to be evaluated by addition of modulators, and the impact of chemical compounds on mitochondrial homeostasis and the electron transport chain (ETC) to be assessed (Jönsson et al., 2007; Obeidat et al., 2018; Gnaiger, 2020). Diuron has been linked to mitochondrial dysfunction in various experimental models, including human cancer cells (Mohammed et al., 2020). On the basis of metabolomic analysis of mitochondria isolated from urothelial cells of male Wistar rats exposed to 10–100 μM Diuron and metabolites, it has suggested that mitochondrial dysfunction is a potential molecular initiating event (MIE) of Diuron toxicity (Lima et al., 2022a). Nevertheless, we did not observe significantly altered OCR in zebrafish after exposure to the tested Diuron, DCA, or DCPMU concentrations.
We also evaluated whether exposure to Diuron, DCA, or DCPMU affects the enzyme AChE. Some authors have shown that Diuron causes neurological alterations in experimental models (Lima et al., 2022a). We verified inhibited AChE activity in zebrafish larvae exposed to 10 μM DCA, which suggested that DCA is neurotoxic to zebrafish in early life stages. Evaluating the neurotoxicity of contaminants is relevant. Lima et al. (2022b) also observed neurotoxicity in larvae of the model organism Caenorhabditis elegans after exposure to Diuron, DCA, or DCPMU.
Adult zebrafish exposed to 4.29 nM Diuron for 14 and 21 days presented damaged DNA in liver and sperm cells, as detected by the comet assay, indicating that Diuron genotoxicity depends on the exposure time (Bony et al., 2010). Furthermore, according to research on trochophore oyster larvae, Diuron genotoxicity (0.00858–2.145 nM) has been suggested to underlie embryotoxicity in these oysters. Behrens et al. (2016) found that the comet tail intensity increases with the DCA (0.01234–3.0863 nM, 6 hpf) or DCPMU (0.00912–2.282 nM, 6 hpf) exposure in oyster larvae. These findings suggested that Diuron and its metabolites can damage DNA by causing single or double breaks in phosphodiester bonds, alkaline-labile sites, or cross-links (Collins, 2004). Thus, the results of the present study support the findings of previous studies by suggesting that Diuron and its metabolites have genotoxic potential and significantly damage DNA at the tested concentrations. However, the fragments are reparable as evidenced by the absence of micronuclei, which are chromosomal fragments that indicate mutagenic effects.
As indicated in the present study, Diuron, classified as non-mutagenic by the U.S. EPA and EFSA regulatory agencies (US EPA, 2003; EFSA, 2005), does not cause the percentage of micronuclei to increase in adult zebrafish erythrocytes. Nevertheless, the Diuron concentration and duration of exposure may affect micronucleus formation, as observed in adult zebrafish exposed to Diuron (Bony et al., 2010). Diuron has been theorized to be aneugenic and may impact mitotic chromosome segregation (Bony et al., 2010), and the mutagenic nature of Diuron in several organisms such as oysters and fish has been confirmed (Bouilly et al., 2007; Michael, 2018).
The cause of NA varies and determines the shape of the nuclei after exposure. Bubbles or buds indicate genotoxic damage, while notched nuclei suggest cytotoxicity (Bolognesi and Cirillo, 2014). Diuron, DCA, and DCPMU have been found to exhibit cytotoxic effects in numerous contexts (Huovinen et al., 2015; Mohammed et al., 2020). Lobed nuclei, extensions that indicate cytotoxicity, differ morphologically from bubbles and buds. Nuclear constriction and kidney-shaped nuclei, classified as nuclear pleomorphisms, may result from alterations in mitotic spindle fibers. NA in erythrocytes have been associated with genotoxic, cytotoxic, and epigenetic mechanisms (Bolognesi and Cirillo, 2014; Canedo et al., 2021). Diuron, DCA, and DCPMU have been shown to affect epigenetic factors, to disrupt the microtubule of the metaphase spindle, to cause cytogenetic effects, and to alter DNA methylation and histone proteins (Ren et al., 2017). In this context, DCA disrupts the cell cycle by interfering in the anaphase complex and expression of mitosis-specific genes (Sawle et al., 2010).
Herein, we histologically evaluated the toxicity of Diuron, DCA, and DCPMU in the liver, spleen, and brain of adult zebrafish tissues because histological alterations serve as useful biomarkers of environmental contamination (Shah and Parveen, 2022). Although Diuron is biotransformed to DCA and DCPMU, we did not find any significant effects when we assessed the acute effects of exposure to these metabolites for 96 h on adult zebrafish liver, spleen, or brain. The potential effects of these compounds on adult zebrafish liver, spleen, or brain during this exposure time have not been described. On the other hand, we verified mild structural changes, vacuolization, and hyaline droplets inside hepatocytes of adult zebrafish at higher DCA or DCPMU concentrations (10 μM). Diuron has been shown to affects rat livers (da Silva Simões et al., 2017). Kamarudin et al. (2019) demonstrated histopathological changes in the liver of adult medaka fish (Oryzias javanicus) exposed to 500 or 1,000 μg/L (2.14 or 4.3 μM) Diuron. Recently, it has been shown that DCA promotes fatty liver in zebrafish larvae exposed to 1–10 μM DCA (Park et al., 2020).
On the basis of our multi-parametric analysis of Diuron, DCA, and DCPMU, the metabolites induce more toxic effects than Diuron at different stages of zebrafish development. Specifically, DCA exerts heightened toxic effects during the early stages of zebrafish development, as indicated by mortality and morphological alterations, followed by DCPMU, which displays less severe toxic effects or toxic effects at higher concentrations. However, regarding adult zebrafish exposure to these compounds, DCPMU impacts genomic or nuclear instability more markedly, as evidenced by NA, which suggests that this metabolite is more cytotoxic at this developmental stage. This study contributes to a growing body of research conducted by our TOXICAM group, which has identified the higher toxicity of Diuron metabolites in other model organisms. For instance, Da Rocha et al. (2014) identified DCA as a potential carcinogen for the bladder epithelium of Wistar rats, while Lima et al. (2022b) demonstrated the essential role played by DCA in the global toxicity of Diuron in C. elegans.
With respect to genetic toxicology parameters, specifically DNA damage, the degree of DNA damage elicited by the tested compounds is not significantly different as evidenced by the comet tail intensity, which corroborates the study of Behrens et al. (2016). Therefore, this DNA repair mechanism should be explored in future studies by accomplishing the comet assay on the erythrocytes of the same adults in which the micronuclei will be quantified. This suggestion is based on the fact that we did not observe changes in these parameters, so conducting these studies could indicate whether the adult stage of zebrafish development is more affected by the genotoxic effects of these compounds.
Bearing the continuous use of Diuron, its biotransformation to DCA and DCPMU, and the discharge of these compounds into the environment, the possible damage that these products may cause to the aquatic ecosystem and human health is of concern. The results of this study highlight the importance of analyzing tested and non-tested organisms to build the weight of evidence for environmental and human health. Practically, the results underscore that it is important to assess the toxicity of pesticide metabolites by considering their persistence in the environment. Furthermore, these findings are relevant in the regulatory field and may contribute to re-evaluation and regulation of Diuron by the competent authorities. Finally, this study highlights the growing importance of zebrafish as model organism in the field of environmental toxicology.
Data availability statement
The original contributions presented in the study are included in the article/Supplementary material, further inquiries can be directed to the corresponding author.
Ethics statement
The animal study was approved by Committee on Ethics in the Use of Animals of Botucatu Medical School, São Paulo State University. The study was conducted in accordance with the local legislation and institutional requirements.
Author contributions
PP: Conceptualization, Data curation, Formal analysis, Investigation, Methodology, Project administration, Software, Supervision, Validation, Visualization, Writing – original draft, Writing – review & editing. BS: Conceptualization, Data curation, Formal analysis, Investigation, Methodology, Project administration, Software, Supervision, Validation, Visualization, Writing – original draft, Writing – review & editing. LA: Formal analysis, Investigation, Methodology, Software, Supervision, Visualization, Writing – review & editing. CV: Conceptualization, Investigation, Methodology, Validation, Visualization, Writing – review & editing. LP: Conceptualization, Data curation, Funding acquisition, Investigation, Project administration, Resources, Supervision, Validation, Visualization, Writing – original draft, Writing – review & editing.
Funding
The author(s) declare financial support was received for the research, authorship, and/or publication of this article. This work was supported by The National Council for Scientific and Technological Development (CNPq) of Brazil under Grant (13687/2021-2) and São Paulo Research Foundation (FAPESP) under Grant (22/03045-4).
Acknowledgments
We thank Alicia Juliana Kowaltowski, PhD, from the Energy Metabolism Laboratory at São Paulo University, São Paulo/SP, and Rafael Henrique Nobrega, PhD, from the Reproductive and Molecular Biology Group at São Paulo State University, Botucatu/SP, for their technical support.
Conflict of interest
The authors declare that the research was conducted in the absence of any commercial or financial relationships that could be construed as a potential conflict of interest.
Publisher's note
All claims expressed in this article are solely those of the authors and do not necessarily represent those of their affiliated organizations, or those of the publisher, the editors and the reviewers. Any product that may be evaluated in this article, or claim that may be made by its manufacturer, is not guaranteed or endorsed by the publisher.
Supplementary material
The Supplementary Material for this article can be found online at: https://www.frontiersin.org/articles/10.3389/frwa.2024.1401712/full#supplementary-material
References
Abe, F. R., Dorta, D. J., Gravato, C., and de Oliveira, D. P. (2024). Elucidating the effects of pure glyphosate and a commercial formulation on early life stages of zebrafish using a complete biomarker approach: All-or-nothing! Sci. Total Environ. 916, 170012. doi: 10.1016/j.scitotenv.2024.170012
Atwood, D., and Paisley-Jones, C. (2017). Pesticides Industry Sales and Usage: 2008-2012 Market Estimates.
Barizon, R. R. M., de Figueiredo, R., de Souza Dutra, D. R. C., Regitano, J. B., and Ferracini, V. L. (2020). Pesticides in the surface waters of the Camanducaia River watershed, Brazil. J. Environ. Sci. Health B 55, 283–292. doi: 10.1080/03601234.2019.1693835
Behrens, D., Rouxel, J., Burgeot, T., and Akcha, F. (2016). Comparative embryotoxicity and genotoxicity of the herbicide diuron and its metabolites in early life stages of Crassostrea gigas: implication of reactive oxygen species production. Aquat. Toxicol. 175, 249–259. doi: 10.1016/j.aquatox.2016.04.003
Bolognesi, C., and Cirillo, S. (2014). Genotoxicity biomarkers in aquatic bioindicators. Curr. Zool. 60, 273–284. doi: 10.1093/czoolo/60.2.273
Bony, S., Gaillard, I., and Devaux, A. (2010). Genotoxicity assessment of two vineyard pesticides in zebrafish. Int. J. Environ. Anal. Chem. 90, 421–428. doi: 10.1080/03067310903033659
Bouilly, K., Bonnard, M., Gagnaire, B., Renault, T., and Lapègue, S. (2007). Impact of diuron on aneuploidy and hemocyte parameters in Pacific Oyster, Crassostrea gigas. Arch. Environ. Contam. Toxicol. 52, 58–63. doi: 10.1007/s00244-005-0256-8
Bradford, M. M. (1976). A rapid and sensitive method for the quantitation of microgram quantities of protein utilizing the principle of protein-dye binding. Anal. Biochem. 72, 248–254. doi: 10.1016/0003-2697(76)90527-3
Brasil (2022). Resolution No. 56 of 5 October 2022. Brasília: Brasil, Ministry of Science Technology, and Innovation and National Council for the Control of Animal Experimentation. Available at: https://www.in.gov.br/web/dou/-/resolucao-n-56-de-5-de-outubro-de-2022-434544861 (accessed July 5, 2023).
Brasil and Ministry of Health (2011). Ordinance No 2.914. Brasília: Gabinete do Ministro. Available at: https://bvsms.saude.gov.br/bvs/saudelegis/gm/2011/prt2914_12_12_2011.html (accessed July 4, 2023).
Caldas, S. S., Arias, J. L. O., Rombaldi, C., Mello, L. L., Cerqueira, M. B. R., Martins, A. F., et al. (2019). Occurrence of pesticides and PPCPs in surface and drinking water in southern Brazil: Data on 4-year monitoring. J. Braz. Chem. Soc. 30, 71–80. doi: 10.21577/0103-5053.20180154
Camargo, M. M. P., and Martinez, C. B. R. (2007). Histopathology of gills, kidney and liver of a Neotropical fish caged in an urban stream. Neotrop. Ichthyol. 5, 327–336. doi: 10.1590/S1679-62252007000300013
Cammer, W. (1982). Release of mitochondrial respiratory control by cyanate salts. Biochim. Biophys. Acta 679, 343–346. doi: 10.1016/0005-2728(82)90305-X
Canedo, A., de Jesus, L. W. O., Bailão, E. F. L. C., and Rocha, T. L. (2021). Micronucleus test and nuclear abnormality assay in zebrafish (Danio rerio): Past, present, and future trends. Environ. Pollut. 290:118019. doi: 10.1016/j.envpol.2021.118019
Carena, L., Proto, M., Minella, M., Ghigo, G., Giovannoli, C., Brigante, M., et al. (2018). Evidence of an important role of photochemistry in the attenuation of the secondary contaminant 3,4-dichloroaniline in paddy water. Environ. Sci. Technol. 52, 6334–6342. doi: 10.1021/acs.est.8b00710
Çavaş, T. (2008). In vivo genotoxicity of mercury chloride and lead acetate: micronucleus test on acridine orange stained fish cells. Food Chem. Toxicol. 46, 352–358. doi: 10.1016/j.fct.2007.08.015
CETESB (2021). Diagnosis of surface water, groundwater and sediment contamination by pesticides. São Paulo. Available at: http://cetesb.sp.gov.br/publicacoes-relatorios/ (accessed June 29, 2023).
Collins, A. R. (2004). The comet assay for DNA damage and repair: principles, applications, and limitations. Appl. Biochem. Biotechnol. 26, 249–261. doi: 10.1385/MB:26:3:249
Da Rocha, M. S., Arnold, L. L., De Oliveira, M. L. C. S., Catalano, S. M. I., Cardoso, A. P. F., Pontes, M. G. N., et al. (2014). Diuron-induced rat urinary bladder carcinogenesis: Mode of action and human relevance evaluations using the international programme on chemical safety framework. Crit. Rev. Toxicol. 44, 393–406. doi: 10.3109/10408444.2013.877870
Da Rocha, M. S., Arnold, L. L., Dodmane, P. R., Pennington, K. L., Qiu, F., De Camargo, J. L. V., et al. (2013). Diuron metabolites and urothelial cytotoxicity: in vivo, in vitro and molecular approaches. Toxicology 314, 238–246. doi: 10.1016/j.tox.2013.10.005
da Silva Simões, M., Bracht, L., Parizotto, A. V., Comar, J. F., Peralta, R. M., and Bracht, A. (2017). The metabolic effects of diuron in the rat liver. Environ. Toxicol. Pharmacol. 54, 53–61. doi: 10.1016/j.etap.2017.06.024
de Souza Anselmo, C., Sardela, V. F., de Sousa, V. P., and Pereira, H. M. G. (2018). Zebrafish (Danio rerio): a valuable tool for predicting the metabolism of xenobiotics in humans? Comp. Biochem. Physiol. Part C 212, 34–46. doi: 10.1016/j.cbpc.2018.06.005
Di Bernardo Dantas, A., Paschoalato, C. F. R., Martinez, M. S., Ballejo, R. R., and Di Bernardo, L. (2011). Removal of diuron and hexazinone from guarany aquifer groundwater. Braz. J. Chem. Eng. 28, 415–424. doi: 10.1590/S0104-66322011000300007
EFSA (2005). Conclusion regarding the peer review of the pesticide risk assessment of the active substance Diuron. EFSA J. 3, 1–58. doi: 10.2903/j.efsa.2005.25r
Ellman, G. L., Courtney, K. D., Andres, V., and Featherstone, R. M. (1961). A new and rapid colorimetric determination of acetylcholinesterase activity. Biochem. Pharmacol. 7, 88–95. doi: 10.1016/0006-2952(61)90145-9
Ensminger, M. P., Budd, R., Kelley, K. C., and Goh, K. S. (2013). Pesticide occurrence and aquatic benchmark exceedances in urban surface waters and sediments in three urban areas of California, USA, 2008-2011. Environ. Monit. Assess. 185, 3697–3710. doi: 10.1007/s10661-012-2821-8
Felício, A. A., Freitas, J. S., Scarin, J. B., de Souza Ondei, L., Teresa, F. B., Schlenk, D., et al. (2018). Isolated and mixed effects of diuron and its metabolites on biotransformation enzymes and oxidative stress response of Nile tilapia (Oreochromis niloticus). Ecotoxicol. Environ. Saf. 149, 248–256. doi: 10.1016/j.ecoenv.2017.12.009
Field, J. A., Reed, R. L., Sawyer, T. E., Griffith, S. M., and Wigington, P. J. (2003). Diuron occurrence and distribution in soil and surface and ground water associated with grass seed production. J. Environ. Qual. 32, 171–179. doi: 10.2134/jeq2003.171
Finoto Viana, L., do Amaral Crispim, B., Kummrow, F., Alice de Lima, N., Amaral Dias, M., Carolina Montagner, C., et al. (2023). Occurrence of contaminants of emerging concern and their risks to the Pantanal Sul-Mato-Grossense aquatic biota, Brazil. Chemosphere 337:139429. doi: 10.1016/j.chemosphere.2023.139429
Gibson-Corley, K. N., Olivier, A. K., and Meyerholz, D. K. (2013). Principles for valid histopathologic scoring in research. Vet. Pathol. 50, 1007–1015. doi: 10.1177/0300985813485099
Gnaiger, E. (2020). Mitochondrial Pathways and Respiratory Control. An Introduction to OXPHOS Analisys.
Gonçalves, Í. F. S., Souza, T. M., Vieira, L. R., Marchi, F. C., Nascimento, A. P., and Farias, D. F. (2020). Toxicity testing of pesticides in zebrafish—a systematic review on chemicals and associated toxicological endpoints. Environ. Sci. Pollut. Res. 27, 10185–10204. doi: 10.1007/s11356-020-07902-5
Gooddy, D. C., Chilton, P. J., and Harrison, I. (2002). A field study to assess the degradation and transport of diuron and its metabolites in a calcareous soil. Sci. Total Environ. 297, 67–83. doi: 10.1016/S0048-9697(02)00079-7
Guilhermino, L., Soares, A. M., Carvalho, A. P., and Lopes, M. C. (1998). Acute effects of 3,4-dichloroaniline on blood of male wistar rats. Chemosphere 37, 619–632. doi: 10.1016/S0045-6535(98)00087-3
Hernández, A. F., Parrón, T., Tsatsakis, A. M., Requena, M., Alarcón, R., and López-Guarnido, O. (2013). Toxic effects of pesticide mixtures at a molecular level: their relevance to human health. Toxicology 307, 136–145. doi: 10.1016/j.tox.2012.06.009
Huovinen, M., Loikkanen, J., Naarala, J., and Vähäkangas, K. (2015). Toxicity of diuron in human cancer cells. Toxicology in Vitro 29, 1577–1586. doi: 10.1016/j.tiv.2015.06.013
IBAMA (2021). Environmental Assessment for the Registration of Pesticides, Their Components and Related Products for Agricultural Use. Ministry of the Environment, 1. Available at: http://www.ibama.gov.br/agrotoxicos/avaliacao-ambiental/avaliacao-ambiental-para-registro-de-agrotoxicos-seus-componentes-e-afins-de-uso-agricola#:~:text=A obrigatoriedade de uma avaliação,exigências relativas ao meio ambiente (accessed July 3, 2022).
ISO (2007). Water quality—Determination of the acute toxicity of waste water to zebrafish eggs (Danio rerio). International Organization for Standardization.
Jönsson, M. E., Orrego, R., Woodin, B. R., Goldstone, J. V., and Stegeman, J. J. (2007). Basal and 3,3′,4,4′,5-pentachlorobiphenyl-induced expression of cytochrome P450 1A, 1B and 1C genes in zebrafish. Toxicol. Appl. Pharmacol. 221, 29–41. doi: 10.1016/j.taap.2007.02.017
Kamarudin, N. A., Zulkifli, S. Z., Zahidah, F., Aziz, A., and Ismail, A. (2019). Histological alterations in liver and kidney of Javanese Medaka (Oryzias javanicus, Bleeker 1854) exposed to sublethal concentration of herbicide diuron. Pertanika J. Sci. Technol. 27, 1041–1050.
Khabib, M. N. H., Sivasanku, Y., Lee, H. B., Kumar, S., and Kue, C. S. (2022). Alternative animal models in predictive toxicology. Toxicology 465:153053. doi: 10.1016/j.tox.2021.153053
Kim, N. S., Shim, W. J., Yim, U. H., Hong, S. H., Ha, S. Y., Han, G. M., et al. (2014). Assessment of TBT and organic booster biocide contamination in seawater from coastal areas of South Korea. Mar. Pollut. Bull. 78, 201–208. doi: 10.1016/j.marpolbul.2013.10.043
Kimmel, C. B., Ballard, W. W., Kimmel, S. R., Ullmann, B., and Schilling, T. F. (1995). Stages of embryonic development of the zebrafish. Dev. Dyn. 203, 253–310. doi: 10.1002/aja.1002030302
Kong, L., Kadokami, K., Duong, H. T., and Chau, H. T. C. (2016). Screening of 1300 organic micro-pollutants in groundwater from Beijing and Tianjin, North China. Chemosphere 165, 221–230. doi: 10.1016/j.chemosphere.2016.08.084
Lakshmaiah, G. (2017). Brain histopathology of the fish Cyprinus carpio exposed to lethal concentrations of an organophosphate insecticide phorate. Int. J. Adv. Res. Dev. 2, 668–672. doi: 10.7439/ijasr.v2i4.3233
Li, E., Wang, Y., Li, Q., Li, L., and Wei, L. (2021). Protective effects of sal B on oxidative stress-induced aging by regulating the Keap1/Nrf2 signaling pathway in Zebrafish. Molecules 26:5239. doi: 10.3390/molecules26175239
Lima, T. R. R., de Oliveira Lima, E., Delafiori, J., Ramos Catharino, R., Viana de Camargo, J. L., and Pereira, L. C. (2022a). Molecular signatures associated with diuron exposure on rat urothelial mitochondria. Toxicol. Mech. Methods. 32, 628–635. doi: 10.1080/15376516.2022.2062271
Lima, T. R. R., Martins, A. C., Pereira, L. C., and Aschner, M. (2022b). Toxic effects induced by diuron and its metabolites in Caenorhabditis elegans. Neurotox. Res. 40, 1812–1823. doi: 10.1007/s12640-022-00596-2
Madeira, C. L., Acayaba, R. D. A., Santos, V. S., Villa, J. E. L., Jacinto-Hernández, C., Azevedo, J. A. T., et al. (2023). Uncovering the impact of agricultural activities and urbanization on rivers from the Piracicaba, Capivari, and Jundiaí basin in São Paulo, Brazil: a survey of pesticides, hormones, pharmaceuticals, industrial chemicals, and PFAS. Chemosphere 341:139954. doi: 10.1016/j.chemosphere.2023.139954
Masiá, A., Campo, J., Navarro-Ortega, A., Barceló, D., and Picó, Y. (2015). Pesticide monitoring in the basin of Llobregat River (Catalonia, Spain) and comparison with historical data. Sci. Total Environ. 503–504, 58–68. doi: 10.1016/j.scitotenv.2014.06.095
Michael, P. O. (2018). Sublethal effect of diuron on DNA status, hematological and biochemical indices of fresh water catfish, Clarias gariepinus. Juveniles 6, 621–627.
Mohammed, A. M., Huovinen, M., and Vähäkangas, K. H. (2020). Toxicity of diuron metabolites in human cells. Environ. Toxicol. Pharmacol. 78:103409. doi: 10.1016/j.etap.2020.103409
Nagel, R. (2002). DarT: the embryo test with the Zebrafish Danio rerio–a general model in ecotoxicology and toxicology. Altern. Tierexperimenten 19(Suppl. 1), 38–48.
Obeidat, Y. M., Evans, A. J., Tedjo, W., Chicco, A. J., Carnevale, E., and Chen, T. W. (2018). Monitoring oocyte/embryo respiration using electrochemical-based oxygen sensors. Sens. Actuat. B Chem. 276, 72–81. doi: 10.1016/j.snb.2018.07.157
OECD (2013). Test No. 236: Fish Embryo Acute Toxicity (FET) Test. OECD. doi: 10.1787/9789264203709-en
OECD (2019). Test Guideline 203: Fish, Acute Toxicity Test. OECD Guideline For The Testing of Chemicals, 1–23. doi: 10.1787/9789264069961-en
Park, J.-S., Song, J., Park, J.-S., Lee, S., Lee, J., Park, H.-J., et al. (2020). 3,4-Dichloroaniline promotes fatty liver in zebrafish larvae. Mol. Cell Toxicol. 16, 159–165. doi: 10.1007/s13273-019-00066-5
Pereira, L. C., de Souza, A. O., Bernardes, M. F. F., Pazin, M., Tasso, M. J., Pereira, P. H., et al. (2015). A perspective on the potential risks of emerging contaminants to human and environmental health. Environ. Sci. Pollut. Res. 22, 13800–13823. doi: 10.1007/s11356-015-4896-6
PubChem (2022). 3,4-dicloroanilina, 50. Available at: https://pubchem.ncbi.nlm.nih.gov/compound/7257 (accessed July 18, 2023).
Quirantes, M., Nogales, R., and Romero, E. (2017). Sorption potential of different biomass fly ashes for the removal of diuron and 3,4-dichloroaniline from water. J. Hazard. Mater. 331, 300–308. doi: 10.1016/j.jhazmat.2017.02.047
Rahn, J. J., Stackley, K. D., and Chan, S. S. L. (2013). Opa1 is required for proper mitochondrial metabolism in early development. PLoS ONE 8:59218. doi: 10.1371/journal.pone.0059218
Ren, N., Atyah, M., Chen, W.-Y., and Zhou, C.-H. (2017). The various aspects of genetic and epigenetic toxicology: testing methods and clinical applications. J. Transl. Med. 15:110. doi: 10.1186/s12967-017-1218-4
Russel, W. M. S., and Burch, R. L. (1959). Recovering the principles of humane experimental technique: the 3Rs and the human essence of animal research. Principl. Hum. Exp. Tech. 43, 622–648. doi: 10.1177/0162243917726579
Sawle, A. D., Wit, E., Whale, G., and Cossins, A. R. (2010). An information-rich alternative, chemicals testing strategy using a high definition toxicogenomics and zebrafish (Danio rerio) embryos. Toxicol. Sci. 118, 128–139. doi: 10.1093/toxsci/kfq237
Scheil, V., Kienle, C., Osterauer, R., Gerhardt, A., and Köhler, H.-R. (2009). Effects of 3,4-dichloroaniline and diazinon on different biological organisation levels of zebrafish (Danio rerio) embryos and larvae. Ecotoxicology 18, 355–363. doi: 10.1007/s10646-008-0291-0
Schiwy, S., Herber, A.-K., Hollert, H., and Brinkmann, M. (2020). New insights into the toxicokinetics of 3,4-dichloroaniline in early life stages of zebrafish (Danio rerio). Toxics 8:16. doi: 10.3390/toxics8010016
Shah, Z. U., and Parveen, S. (2022). Oxidative, biochemical and histopathological alterations in fishes from pesticide contaminated river Ganga, India. Sci. Rep. 12:3628. doi: 10.1038/s41598-022-07506-8
Skerratt, J., Baird, M. E., Mongin, M., Ellis, R., Smith, R. A., Shaw, M., et al. (2023). Dispersal of the pesticide diuron in the Great Barrier Reef. Sci. Total Environ. 879:163041. doi: 10.1016/j.scitotenv.2023.163041
Tal, T., Yaghoobi, B., and Lein, P. J. (2020). Translational toxicology in zebrafish. Curr. Opin. Toxicol. 23–24, 56–66. doi: 10.1016/j.cotox.2020.05.004
US EPA (2003). Reregistration Eligibility Decision (RED) for Diuron. US EPA Archive Document, United States Enviromental Protection Agency, 211. Available at: http://library1.nida.ac.th/termpaper6/sd/2554/19755.pdf
Viana, J. L. M., dos Santos, S. R. V., dos Santos Franco, T. C. R., and Almeida, M. A. P. (2019). Occurrence and partitioning of antifouling booster biocides in sediments and porewaters from Brazilian Northeast. Environ. Pollut. 255:112988. doi: 10.1016/j.envpol.2019.112988
Voelker, D., Vess, C., Tillmann, M., Nagel, R., Otto, G. W., Geisler, R., et al. (2007). Differential gene expression as a toxicant-sensitive endpoint in zebrafish embryos and larvae. Aquat. Toxicol. 81, 355–364. doi: 10.1016/j.aquatox.2006.12.013
von Hellfeld, R., Brotzmann, K., Baumann, L., Strecker, R., and Braunbeck, T. (2020). Adverse effects in the fish embryo acute toxicity (FET) test: a catalogue of unspecific morphological changes versus more specific effects in zebrafish (Danio rerio) embryos. Environ. Sci. Eur. 32:122. doi: 10.1186/s12302-020-00398-3
Westerfield, M. (2000). The Zebrafish Book. A Guide for the Laboratory Use of Zebrafish (Danio rerio), 4th Edn. Eugene: University of Oregon. Available at: https://zfin.org/zf_info/zfbook/zfbk.html (accessed June 20, 2023).
Zaluski, A. B., Wiprich, M. T., de Almeida, L. F., de Azevedo, A. P., Bonan, C. D., and Vianna, M. R. M. (2022). Atrazine and Diuron effects on survival, embryo development, and behavior in larvae and adult zebrafish. Front. Pharmacol. 13:841826. doi: 10.3389/fphar.2022.841826
Zhu, B., Liu, T., Hu, X., and Wang, G. (2013). Developmental toxicity of 3,4-dichloroaniline on rare minnow (Gobiocypris rarus) embryos and larvae. Chemosphere 90, 1132–1139. doi: 10.1016/j.chemosphere.2012.09.021
Keywords: embryotoxicity, genotoxicity, mutagenicity, acetylcholinesterase activity, histopathology
Citation: Peixoto PVL, Sales BCP, Aggio L, Viriato C and Pereira LC (2024) Multiparameter analysis of Diuron and its metabolites (DCA and DCPMU) in different stages of zebrafish (Danio rerio) development. Front. Water 6:1401712. doi: 10.3389/frwa.2024.1401712
Received: 15 March 2024; Accepted: 30 August 2024;
Published: 24 September 2024.
Edited by:
Geonildo Rodrigo Disner, Butantan Institute, BrazilReviewed by:
Temitope O. Sogbanmu, University of Lagos, NigeriaIzonete Guiloski, Pelé Pequeno Príncipe Research Institute, Brazil
Copyright © 2024 Peixoto, Sales, Aggio, Viriato and Pereira. This is an open-access article distributed under the terms of the Creative Commons Attribution License (CC BY). The use, distribution or reproduction in other forums is permitted, provided the original author(s) and the copyright owner(s) are credited and that the original publication in this journal is cited, in accordance with accepted academic practice. No use, distribution or reproduction is permitted which does not comply with these terms.
*Correspondence: Lilian Cristina Pereira, bGlsaWFuLnBlcmVpcmEmI3gwMDA0MDt1bmVzcC5icg==
†These authors have contributed equally to this work