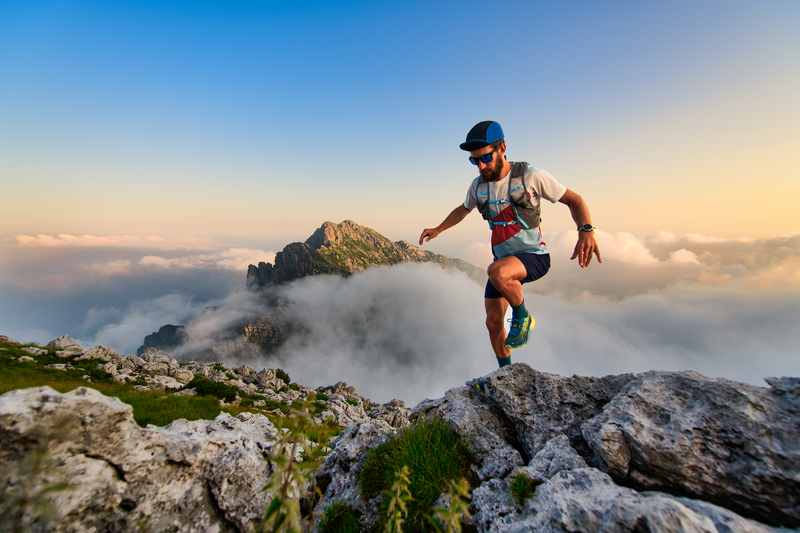
95% of researchers rate our articles as excellent or good
Learn more about the work of our research integrity team to safeguard the quality of each article we publish.
Find out more
ORIGINAL RESEARCH article
Front. Water , 19 February 2024
Sec. Water and Hydrocomplexity
Volume 6 - 2024 | https://doi.org/10.3389/frwa.2024.1286968
This article is part of the Research Topic Enhancing Coastal Resilience Through Nature-Based Solutions: Bridging Fluvial and Marine Ecosystems View all articles
Several studies on intermittent rivers and ephemeral streams (IRES) have focused on the ecology of populations and communities, and very few explored the biogeochemistry and bioavailability of key elements, such as phosphorus (P) and nitrogen (N). In this study, we aimed to explore the role of environmental seasonality on P bioavailability in an intermittent river in Northeastern Brazil and the implications for nutrient dynamics to downstream ecosystems, such as the estuaries. The study was performed at the Cruxati River, located in the Litoral Hydrographic Basin on the coast of Ceará State, Brazil. We sampled riverine sediments during three hydroperiods (i.e., dry, rewetting, and flow) according to the theoretical model for research in intermittent rivers. We performed a geochemical fractionation of different P forms and determined the physicochemical parameters, particle size distribution, mineralogy, and total organic carbon of the sediments. Mean values were compared using the Kruskal–Wallis non-parametric statistical test (p-value < 0.05) and by discriminant function analysis. We observed that the predominant fractions of P were associated with Fe-oxides, silicates, and refractory organic matter, but there were no statistical differences among these fractions for all hydroperiods. The exchangeable-P form was higher in the dry and flow periods than in the rewetting period. On the opposite side, P associated with humic acids and carbonates was lower in the dry and flow periods but higher in the rewetting period. We concluded that seasonality influences the bioavailability of P in intermittent rivers, being strongly influenced by the organic fraction, including the humic acid fraction and the refractory organic matter, which are important sources of P in the aquatic system. Furthermore, the IRES may act as a source or a sink of nutrients throughout the cycles of wetting and drying, which may release P from the sediments to the aquatic environment. Hence, IRES plays an important role in transporting nutrients to estuaries and maintaining their ecosystem services.
Intermittent rivers and ephemeral streams (IRES) are characterized by drying up their streamflow over the year. These lentic habitats are fragmented during the dry season, forming isolated pools on the river bed. Depending on the rainfall regime and frequency and duration of streamflow, the isolated pools may last from days to months (Acuña et al., 2014; Datry et al., 2014; Bonada et al., 2020; Perez et al., 2020). These environments represent more than 30% of rivers in the world and occur mostly in arid and semi-arid regions (Larned et al., 2010; Datry et al., 2014). Despite their intermittent regime, the IRES provide several ecosystem services, such as habitat for a variety of aquatic and terrestrial species, structuring populations and communities of plants and animals, nutrient cycling, water supply, and fishing (Skoulikidis and Amaxidis, 2009; Larned et al., 2010; Acuña et al., 2014; Datry et al., 2014; Leigh et al., 2015; Acuña et al., 2017).
However, most studies on IRES have focused on the ecology of populations and communities (Farias et al., 2012; Queiroz and Terra, 2019; Rodrigues Filho et al., 2020; Gonçalves-Silva et al., 2022), and very few explored the biogeochemical cycling of nutrients within the IRES (Acuña et al., 2017). Studies that explore biogeochemical processes in IRES were only leveraged at the beginning of the 21st century (Leigh et al., 2015), and few studies focused on quantification and bioavailability of limiting nutrients for primary production, such as phosphorus (P) and nitrogen (N). For these biogeochemical cycling studies, the works of von Schiller deserve special mention e.g., (see von Schiller et al., 2007, 2008, 2009, 2010, 2011, 2015). With work focused on the ecology and biogeochemistry of intermittent rivers (i.e., Sediment respiration pulses in intermittent rivers and ephemeral streams; Nutrient and organic matter dynamics in intermittent rivers and ephemeral streams; Regulation causes nitrogen cycling discontinuities in Mediterranean rivers; A comparison of two empirical approaches to estimate in-stream net nutrient uptake; A round-trip ticket: the importance of release processes for in-stream nutrient spiraling; and Inter-annual, annual, and seasonal variation of P and N retention in a perennial and an intermittent stream), this author has been attempting to elucidate the dynamics of nutrients, primarily N and P, and organic matter in IRES.
The streamflow dynamics across the watershed may impact the biogeochemistry and the living organisms locally and downstream. One of the nutrients that are important for sustaining life interconnected between aquatic and terrestrial is phosphorus (P) (Martins et al., 2007; Attygalla et al., 2016; O’Connell et al., 2018; Horppila, 2019). For instance, when IRES are in a flowing regime, significant amounts of P are carried out across the river and reach the estuarine ecosystems (e.g., mangrove, salt marshes, and seagrass meadows) (Lacerda et al., 2008). Additionally, seasonal effects on fluvial discharge have significant impacts on estuarine ecosystems (Lacerda et al., 2008; Nóbrega et al., 2013). Lower freshwater inputs during drier periods may decrease the concentration of P in estuaries and change primary productivity (e.g., causing a decrease in phytoplankton and affecting upper levels of the food chain) (Barroso et al., 2016).
Moreover, in soils and sediments, the dynamics of P are conditioned by the interaction with the soil/sediments matrix (i.e., clay minerals and organic matter) (Cornell and Schwertmann, 2003; Cavalcante et al., 2018). In the mineral fraction, Fe oxyhydroxides are important for the retention of P because of the different degrees of crystallinity, surface area, and hydroxyl (OH) groups (Cornell and Schwertmann, 2003; Otero et al., 2008; Fink et al., 2016; Queiroz et al., 2021). These interactions are among the most important in regulating the quantity and forms of P within terrestrial and aquatic ecosystems (Fink et al., 2016; Barcellos et al., 2019; Queiroz et al., 2021). Additionally, parameters such as pH, Eh, microbial activity, salinity, and organic matter have an influence on the dynamics of P (Nóbrega et al., 2014; Parsons et al., 2017; Barcellos et al., 2019). Consequently, depending upon the biogeochemical conditions, soils and sediments may act as sinks or sources of P.
In semi-arid regions where most rivers are intermittent, P availability may be limited by the low rainfall and the low river discharge, which results in low P concentrations in the water (Lacerda et al., 2006, 2008; Barroso et al., 2016). In the ecoregion Caatinga Phytogeographic Domain (Moro et al., 2016), in the northeast of Brazil, there is a large semi-arid coast that is occupied mostly by mangrove ecosystems (accounting for 45% of the Brazilian mangrove area) (Brasil, Ministério do Meio Ambiente, 2018), where the upstream P inputs impact the productivity of coastal ecosystems. Although several studies indicated that mangroves in northeastern Brazil receive high loads of P from anthropogenic activities (Nóbrega et al., 2014; Godoy and Lacerda, 2015), there are no studies exploring the natural sources of P, such as the IRES systems impacting P dynamics in estuaries.
Laboratory and field experiments exploring the release of P over “wet” and “dry” cycles are still discrepant. Schönbrunner et al. (2012) studied sediments drying and re-inundation in laboratory settings and indicated that the degree of drying is a determining factor in controlling the release of P to the aqueous phase. According to these authors, upon reconnection of water flow, phosphorus is released in large amounts. In experimental laboratory studies, Attygalla et al. (2016) observed an increase in exchangeable phosphorus content after the drying process and subsequent wetting of sediments. However, other forms of non-available phosphorus associated with minerals did not exhibit significant variations (Attygalla et al., 2016). O’Connell et al. (2018) reported that drying events decreased the amount of organic phosphorus, thus influencing the organic phosphorus mineralization process. Furthermore, the results remain poorly understood due to the influence of other variables such as Eh, pH, amounts of organic matter (Kim et al., 2016), and biological factors (Kong et al., 2021).
In environments with intense seasonality, such as intermittent rivers, studies carried out “in loco” involving nutrient cycling are still scarce. Thus, understanding the cycling of nutrients (especially P) in these rivers is essential for understanding the ecological and biogeochemical processes that structure these ecosystems (Lillebo et al., 2007; Tzoraki et al., 2007; Skoulikidis and Amaxidis, 2009; Larned et al., 2010; Steward et al., 2012; Datry et al., 2014; Skoulikidis et al., 2017). Therefore, this study aimed to investigate the role of hydrological dynamics on P bioavailability in an intermittent river in Northeast Brazil and the implications for nutrient cycling to adjacent and downstream ecosystems.
The Cruxati River is located within the Cruxati basin, containing an area of approximately 77 km2 in the State of Ceará, NE-Brazil (Figure 1), flowing into another significant river in the region, the Mundaú River. In this region, the vegetation is diverse, characterized by a complex vegetation typical of the local coastal zone, as well as sub-deciduous tropical rainforest (dry forest), tropical sub-evergreen rain-cloud forest (humid forest), dense shrub caatinga, and open shrub caatinga. The predominant local geology is composed of metamorphic and sedimentary rocks, with the presence of Luxisols, Luvisols, Fluvisols, Planosols, and Regosols (Funceme – Fundação Cearense de Meteorologia e Recursos Hídricos, 2018).
Figure 1. Study area in the state of Ceará, Brazil, with details of elevation, the Cruxatí river basin area, drainage, monthly rainfall, and evapotranspiration (ETo) distribution along the year and hydroperiods (A). Illustration of sampled site details during the rewetting (B), flow (C), and dry hydroperiods (D).
The region is characterized by a semi-arid climate with a mean annual precipitation of 1,100 mm, an evapotranspiration rate above 1,400 mm, and annual temperatures ranging between 26°C and 28°C (Figure 1; Funceme – Fundação Cearense de Meteorologia e Recursos Hídricos, 2018; Duarte et al., 2021). The highest precipitation regime occurs during the rainy season (February to April), while during the dry season (August to December), precipitation is nearly zero (Porto et al., 2004; Funceme – Fundação Cearense de Meteorologia e Recursos Hídricos, 2018; Duarte et al., 2021). Thus, the Cruxati River dries up for most of the year, with pools of water in some reaches. However, during the rainy season in December, the streamflow gradually increases, pools begin to connect, and a small flow arises that is fed by heavy rainfall in January and February (Figure 1). However, beyond the rainy season (which concludes in June), the high evaporation rates cause the river to flow again, leading it to quickly dry up.
Sediment and water samples were sampled during three campaigns, one in each of the hydroperiods as follows: Dry (October/November 2018), Flow (July 2019), and Rewetting (January 2020; Figure 1). These hydroperiods were previously proposed in studies involving nutrients dynamic in IRES (von Schiller et al., 2007, 2011; Datry et al., 2016, 2017). Accordingly, the dry period can be characterized when the aboveground water dries up completely and becomes disconnected from the groundwater; the rewetting period represents an intermediate phase where the river has no flow and fragments, with a few pools along the river bed; and the flow is established after highly concentrated rainfall periods, and watercourse quickly settles down and the river has a continuous flow of runoff.
Sediment sampling was carried out along 200 m transects distributed at strategic points along the river as follows: upstream, middle, and downstream. Each transect was divided into three sections (beginning: 0 m; middle: 100 m; and ending: 200 m in length). Subsequently, in each section, sediments were randomly collected at a depth of 0–9 cm from the left bank, right bank, and center of the river channel, totaling three samples per section and nine samples per transect. The collection was performed using polycarbonate tubes (0.05 m internal diameter and 0.5 m length) attached to a sediments sampler (Trado Josefina: Soil sampler specially designed for waterlogged soils and sediments). In total, 27 sediment samples were collected for each hydroperiod. Water samples were collected using amber glass bottles previously washed with HCl 10% (v/v) and stored at a low temperature (~ 4°C) to determine total phosphorus and orthophosphate.
Along the sampling, sediments’ pH and redox potential (Eh) values were measured in situ using a glass electrode calibrated with pH standards of 4.0 and 7.0 and a calomel electrode for Eh, while water’s pH and Eh values were obtained directly in situ using a multimeter probe (YSI 7000).
The particle size distribution (sand, silt, and clay) was obtained using the method proposed by Gee and Bauder (1986). For mineralogical characterization, due to the geological and soil homogeneity along the transects, only one representative sample from each transect was chosen and pre-treated with a sodium hypochlorite solution to oxidize the organic matter and then crushed and sieved (<150 mesh) (Siregar et al., 2005). After separating the granulometric fractions, unoriented slides (powder) were mounted with the clay fraction, and analysis was carried out by X-ray diffraction (XRD) using a Miniflex II Desktop X-ray diffractometer with Cu-Kα radiation at the step of 0.02° 2θ s−1 in the range of 3–60° 2θ (Chen, 1977). The fraction of free or pedogenic iron of high crystallinity was obtained by the dithionite-citrate-bicarbonate method (Mehra and Jackson, 1960), and the fraction of low-crystallinity iron oxyhydroxides was acquired by the acid ammonium oxalate method (Mckeague, 1978). For this analysis, we homogenized the samples and considered a depth of 0–9 cm.
Different phases of phosphorus were obtained by an operational geochemical fractionation according to Paludan and Jensen (1995), Paludan and Morris (1999), and Nóbrega et al. (2014) as follows: Exchangeable-P (EX-P), P adsorbed to iron oxyhydroxides (Oxide-P), P associated to phyllosilicates and aluminum hydroxides (Silicate-P), P associated to humic acids (HA-P), P in carbonates/apatite (Ca-P), and P associated with refractory organic matter (Res-P) (Paludan and Jensen, 1995; Paludan and Morris, 1999; Nóbrega et al., 2014). Phosphorus concentrations were determined by the colorimetric method ascorbic acid/molybdenum blue, and absorbance was obtained using a spectrophotometer with a wavelength of 885 nm (Murphy and Riley, 1962). The different P fractions were analyzed in a sequential extraction as follows:
1 (EX-P): extracted with 20 mL of 1 M MgCl2. Samples were shaken for 1 h and centrifuged at 3,500 rpm for 15 min. 2 (Oxide-P): extracted with 20 mL of 0.11 M sodium bicarbonate and sodium dithionite (NaHCO3 + Na2S2O4) solution. Samples were shaken for 1 h and centrifuged at 3,500 rpm for 15 min. 3 (Silicate-P): extracted with 20 mL of 0.1 M NaOH. Samples were shaken for 18 h and centrifuged at 3,500 rpm for 15 min. After centrifugation, the solution was acidified (pH ~1) and filtered. 4 (HA-P): The material isolated from the previous step was submitted at combustion (550°C), and the ashes were boiled in HCl 1.0 M. 5 (Ca-P): The material obtained from centrifugation in step 3 (Silicate-P) was extracted with 20 mL of solution HCl (37%) 0.5 M. Samples were shaken for 1 h and centrifuged at 3,500 rpm for 15 min. 6 (Res-P): extracted with 20 mL of HCl 1 M (in hot), after combustion at 550°C (2 h). (For more details see Nóbrega et al., 2014).
By using the Past 4.03 (Hammer et al., 2001) and R software (R Core Team, 2019), we performed the non-parametric Kruskal–Wallis test (p-value < 0.05) to assess the differences among the hydroperiods for the different parameters. Additionally, we perform a discriminant analysis to explore associations among variables and hydroperiods (Reimann et al., 2008; Gotelli and Ellison, 2013; Manly and Alberto, 2016).
The mineralogical composition of the collected sediments, analyzed by XRD, revealed that all transects contained similar mineralogical assemblage, composed mainly of clay minerals–kaolinite (d-spacing: 7.14, 4.36, 3.58, 2.49, and 2.34 nm; Figure 2).
Figure 2. XRD patterns from the three sampled transects (i.e., upstream, middle, and downstream) through the Cruxati River. Kaolinite (K) and d-spacing (d).
The particle size distribution was predominantly sandy, and no significant differences were observed among the samples. The average contents of sand, clay, and silt were the following: 93 ± 7.18%, 4.36 ± 4.69%, and 2.64 ± 2.93, respectively.
The sequential chemical extraction of Fe oxides indicates a predominance of crystalline phases (i.e., Fe DCB) compared to low crystalline phases (i.e., Fe OA). Regarding the contents of Fe DCB (Figure 3A), we observed a significantly higher content in the flow hydroperiod (6.7 ± 3.7 mg kg−1) compared to the rewetting (5.0 ± 2.7 g kg−1) and dry periods (3.4 ± 1.3 g kg−1, Figure 3A). On average, the Fe DCB in the flow hydroperiod increased by 34 and 97% compared to rewetting and dry hydroperiods, respectively. On the other hand, although the values of Fe AO were approximately 1.4-fold and 1.2-fold higher during the flow periods compared to the dry and rewetting periods, no significant differences (p-value > 0.05) were observed in Fe AO for all three hydroperiods. The mean values were 0.05 ± 0.04 g kg−1, 0.06 ± 0.04 g kg−1, and 0.07 ± 0.07 g kg−1 for the dry, rewetting, and flow periods, respectively (Figure 3B).
Figure 3. Fe contents of the three hydroperiods investigated. (A) Crystalline Fe extracted by DCB (Dithionite-citrate-bicarbonate), and (B) Low-crystallinity Fe extracted by AO (Ammonium oxalate). Different lowercase letters above the bars indicate the mean (± standard deviation) for either Fe DCB or Fe AO contents that differ statistically between studied hydroperiods (dry, rewetting, and flow) at the 0.05 level of the Kruskal–Wallis test.
We chose to present the geochemical extraction of P in two ways: showing the most predominant fractions among the hydroperiods and percentages (Figure 4A) and averages at the depth of 0–9 cm, with the statistical results (Figure 5). We observed that oxide-P, res-P, and silicate-P were the predominant fractions of P in all studied hydroperiods, representing on average 37.7, 24.5, and 24.2%, respectively (Figure 4B). For the oxide-P fraction, despite the contents being approximately 1.3- and 2-fold higher in the flow hydroperiod (28.61 ± 21.08 mg kg−1) compared to the dry (21.67 ± 16.43 mg kg−1) and rewetting (14.83 ± 7.38 mg kg−1) hydroperiods, no statistical differences were observed among these three fractions across the hydroperiods (p-value > 0.05; Figure 5B). For silicate-P, we observed contents of 15.56 ± 10.66 mg kg−1 (dry), 14.58 ± 5.86 mg kg−1 (rewetting), and 14.87 ± 18.05 mg kg−1 (flow; Figure 5C). Regarding the res-P, the contents for dry, rewetting, and flow were 13.78 ± 5.92 mg kg−1, 10.36 ± 11.47 mg kg−1, and 15.45 ± 12.45 mg kg−1, respectively (Figure 5D).
Figure 4. Phosphorus fractionation showing the most predominant fractions among the hydroperiods (A). It can be observed that oxide-P, silicate-P, and Res-P have greater representativeness in the three investigated hydroperiods (B). The P fractions are the following: Exchangeable P (EX-P); P associated with reducible Fe and Mn oxyhydroxides (oxide-P); P associated with silicates (silicate-P); P associated with humic acids (HA-P); P bound to carbonates/apatite (CA-P); and Residual P (Res-P).
Figure 5. Phosphorus fractionation for the three studied hydroperiods (depth averages 0–9 cm), as follows: (A), Exchangeable P (EX-P); (B), P associated to reducible Fe and Mn oxyhydroxides (oxide-P); (C), P associated to silicates (silicate-P); (D), P associated to humic acids (HA-P); (E), P bound to carbonates/apatite (CA-P); and (F), Residual P (res-P). Different lowercase letters above the bars indicate mean (± standard deviation) P contents in different fractions that differ statistically among the studied hydroperiods (dry, rewetting, and flow) at the 0.05 level of the Kruskal–Wallis test.
However, significant differences were observed for Ex-P, HA-P, and CA-P among the studied hydroperiods (p < 0.001; Figures 5A,D,E). The higher Ex-P contents were recorded in the dry (2.42 ± 0.65 mg kg−1) and flow periods (2.0 ± 0.67 mg kg−1), while no contents were observed in the rewetting period (Figure 5A). Regarding the HA-P, the higher content was observed in the rewetting period (0.33 ± 0.2 mg kg−1), and no contents were observed in the dry and flow periods (Figure 5D). The CA-P contents were significantly higher in the flow (5.94 ± 7.57 mg kg−1) and dry (5.32 ± 6.21 mg kg−1) periods and no contents were observed in the rewetting period (Figure 6).
Figure 6. Mean (± standard deviation) contents for total-P (A) and orthophosphate-P (B) in water for the Cruxati River at dry and flow hydroperiods.
The pH and Eh values in sediments and water differed statistically (Table 1) among the hydroperiods (p-value < 0.05). For pH in sediments, we observed higher mean values in the dry hydroperiod, which was approximately 1.2-fold higher than rewetting and flow hydroperiods (Table 1). Additionally, no differences were observed between rewetting and flow hydroperiods (Table 1). Regarding pH values in water, we observed higher values statistically recorded in the dry hydroperiod and lower values in the rewetting. On average, the pH in water in the dry hydroperiod increased by 27.3 and 18.3% compared to rewetting and flow, respectively (Table 1). For Eh values, we observed both sediments and water; lower mean values recorded in the flow compared to the rewetting and dry hydroperiods represented a decrease of 45.3 and 38.0%, respectively, in the sediments, while in water, the Eh values decreased around 7.2 and 17.3% (Table 1).
Table 1. Mean values for pH and Eh in sediments and river water among the three studied hydroperiods.
We also observed that TOC and DO differed statistically among hydroperiods (Table 2). Higher values of TOC and DO were obtained in the flow hydroperiod, and no significant differences were observed for TOC and DO between the dry and rewetting hydroperiods. Values of TOC in flow hydroperiods were 1.7-fold higher compared to rewetting and dry hydroperiods. For DO, the flow was 1.7-fold higher than rewetting and 1.4-fold higher than dry hydroperiod.
Table 2. Mean values for total organic carbon (TOC) in sediments and dissolved oxygen (DO) in water between the three studied hydroperiods.
The contents of total-P and orthophosphate-P in water did not present significant differences between the dry and flow hydroperiods (p-value < 0.05). The means for total-P at dry and flow hydroperiods were 0.12 ± 0.04 mg L−1 and 0.09 ± 0.05 mg L−1, respectively (Figure 6A). Whereas the orthophosphate-P contents were 0.01 ± 0.01 mg L−1 and 0.02 ± 0.01 mg L−1 for dry and flow hydroperiods, respectively (Figure 6B). Due to problems with the equipment, we were not able to analyze total-P and orthophosphate-P in water for the rewetting hydroperiod.
By performing the discriminant analysis, we were able to observe a clear separation comparing the driest hydroperiod (dry) and both humid hydroperiods (rewetting and flow; Figure 7A). We observed that F1, accounting for 81.95% of the data variability, was primarily composed of pH water, Fe AO, EX-P, Ca-P, silicate-P, and oxide-P (Figure 7B). F2, explaining 18.04% of the data variance, comprised the variables TOC, pH sediments, HA-P, and Eh water (Figure 7B).
Figure 7. Discriminant analysis (DA) for sediments and water for the three studied hydroperiods. (A): hydroperiods investigated; (B): mensurated variables. Where EX-P: P-exchangeable; Oxide-P: P associated with Fe oxyhydroxides; Silicate-P: P associated with phyllosilicates and Al hydroxides; CA-P: P associated with carbonates; HA-P:P associated with humic substances; Res-P: P associated with refractory organic matter; Fe DCB: Fe associated with high crystallinity minerals; Fe AO: Fe associated with low crystallinity minerals; TOC: total organic carbon; DO: Dissolved oxygen; pH (sediments): pH in the sediments; pH (water): pH in the water; Eh (sediments): Eh in the sediments; Eh (water): Eh in the water.
The positioning of the DO vector toward the flow denotes higher contents of this variable during this hydroperiod, distinguishing it from the dry and rewetting hydroperiods. Additionally, the vector of Eh sediments indicates that it is the second-best variable to distinguish between dry and rewetting, with higher values, and flow, with lower values. The positioning of vectors for HA-P toward rewetting and EX-P, CA-P, oxide-P, and TOC positioned oppositely differentiates between rewetting and flow. pH (water) and pH (sediments) are the best discriminators between dry and flow and, mutually with EX-P, between dry and rewetting.
In the Cruxati River, the studied sites were classified as sandy sediments with clay contents below 5%. The Cruxati River basin is geologically characterized by the presence of metamorphic and sedimentary rocks from the Barreiras formation (Nunes et al., 2011). Generally, the soils derived from this formation have low clay content and a mineralogical assemblage consisting of kaolinite and Fe oxyhydroxides (Melo et al., 2002a,b; Correa et al., 2008). Indeed, the XRD analysis indicated a kaolinite predominance (Figure 2), and the extractions of Fe forms [i.e., FeDCB: 5 ± 2.5 g kg−1; FeAO: 0.06 ± 0.05 g kg−1 (Figure 4)]. These values of Fe content are similar to those observed in previous studies investigating the sediments from the Barreiras formation demonstrating low Fe contents as well, ranging from 4 to 43 g kg−1 for Fe DCB and 0.08 to 1.18 g kg−1 for Fe AO (Melo et al., 2002a,b; Correa et al., 2008).
In fact, despite the low clay content in the sediments of the Cruxati River, P was primarily associated with the clay minerals (i.e., oxides-P and silicate-P), representing 38 and 24% of the total P, respectively, in the studied hydroperiods (Figure 5). Therefore, P is predominantly retained on 1:1 clay minerals (e.g., kaolinite) in soil and sediments through processes such as anion ligand exchange (i.e., adsorption), precipitation, and lattice diffusion (Sparks, 2003; Penn and Warren, 2009). Additionally, kaolinite under wetting and drying cycles, which commonly occurs in intermittent rivers, has a low crystallinity because of the Fe3+ present in their crystal structure (Melo et al., 2002a,b; Correa et al., 2008). This low degree of crystallinity increases their specific surface area and their adsorption capacity (Dixon and Schulze, 2002).
Moreover, present in the studied intermittent river, Fe oxyhydroxides have an elevated specific surface area and have been reported to be an important sorbent of P (Darke and Walbridge, 2000; Melo et al., 2001; Da-Peng and Yong, 2010; Queiroz et al., 2021). Additionally, the observed physicochemical conditions (i.e., pH of 6.6 ± 0.3 and Eh of 350 ± 134; Table 1) in the studied hydroperiods indicate a favorable environment for the occurrence and formation of Fe oxyhydroxides (Dixon and Schulze, 2002). The Eh values above +270 mV and pH values circumneutral in sediments in all hydroperiods indicate oxic conditions favorable to Fe oxyhydroxide precipitation (Essington, 2015).
The maintenance and stability of kaolinite and Fe oxyhydroxides under oxic conditions results in long-term sequestration of P in sediments, leading to its low availability in the water column (Baldwin et al., 2000; Melo et al., 2002a,b; Da-Peng and Yong, 2010; Attygalla et al., 2016; Kong et al., 2021). This condition restricts the supply of this essential nutrient to primary producers, higher trophic levels, and nutrient cycling (Martins et al., 2007; Lacerda et al., 2008; Attygalla et al., 2016; O’Connell et al., 2018; Horppila, 2019). Furthermore, the low P content and limited availability contribute to its low exportation to other ecosystems associated with the IRES along the basin (e.g., P transportation toward the estuary).
Among the mineral phases, CA-P is an important fraction controlling the P release under a semi-arid environment (Nóbrega et al., 2013) and ultimately controls its availability in IRES exposed to these dry conditions (Skoulikidis et al., 2017). Our results revealed that P bound to carbonates/apatite represented only 8% of the total P, likely due to susceptibility to dissolution in IRES (Carranza-Edwards et al., 2005; Skoulikidis et al., 2017). According to Kong et al. (2021), in IRES, the wetting and drying cycles regulate the Ca availability and are associated with microbial respiration, which affects the carbonic acid formation, ultimately altering the carbonate mineral formation and dissolution. As a result, the CA-P fraction can serve both as a sink and source of P due to its mineral instability, in contrast to the oxide-P and silicate-P fractions.
However, P associated with organic fractions represents 26% of the total P and may exhibit contrasting dynamics (Figure 4). For example, under oxidizing conditions present in the semi-arid climate, P bound to organic fractions becomes a potential source to the aquatic system because these dry conditions stimulate organic matter decomposition and ultimately the release of organic-bound P (O’Connell et al., 2018). Parallelly, there is an increase in microbial activity in an oxygen-rich environment, which favors organic matter mineralization (Kong et al., 2021). Indeed, the P content associated with organic fractions (i.e., HA-P and Res-P) was significantly lower compared to the P associated with mineral forms (i.e., Oxide-P and Silicate-P) (Figures 5D,F). Furthermore, the lower content of HA-P (on average: 0.33 ± 0.2 mg kg−1), in comparison to Res-P (on average: 13 ± 20 mg kg−1), reflects its higher susceptibility to decomposition for the HA-P fraction (Kelleher and Simpson, 2006; Primo et al., 2011). On the other hand, Res-P is defined as a refractory organic fraction with lower decomposition rates than HA-P (Kelleher and Simpson, 2006). Furthermore, seasonality in a semi-arid climate, which reflects the presence of the three different hydroperiods observed, significantly affects the soil moisture, soil organic matter content, and decomposition, and the P release from soil/sediment to the aqueous phase (Nóbrega et al., 2013, 2014) and dictates the function of IRES ecosystems.
The seasonality that directly impacts the hydrology of IRES poses an effect on P associated with organic fractions, as presented by the easily decomposable form of organic matter (i.e., HA-P) (Kelleher and Simpson, 2006; Primo et al., 2011). Indeed, HA-P was significantly higher during the rewetting than in the dry and flow hydroperiods, and these latter two were similar statistically in P content (Figure 5D). During the rewetting hydroperiod, the decrease in total P (Figure 4) and the absence of EX-P (Figure 5A) are possibly linked to higher nutrient demand by organisms (Kong et al., 2021). This period emerges as a seasonal hot spot for P mobilization and transport, particularly to coastal ecosystems. Consequently, higher concentrations of HA-P (Figure 5D), coinciding with a minimal water pH (Table 1), suggest hydrocarbonate release from decomposing organic material to flow hydroperiod. Subsequently, the flow hydroperiod showed low sediment Eh values (Table 1), indicating slightly hypoxic conditions (Eh: 274 ± 132 mV; Table 1), likely due to the slowed decomposition of fresh organic material deposited during rewetting. Subsequently, the flow hydroperiod showed low sediment Eh values (Table 1), indicating slightly hypoxic conditions (Eh: 274 ± 132 mV; Table 1), likely due to the slowed decomposition of recently deposited fresh organic material during rewetting. Nevertheless, the rapid replenishment of water flow and freshwater influx within the IRES during the flow hydroperiod promotes elevated DO levels (Gómez et al., 2017; Schreckinger et al., 2022; Table 2). Simultaneously, enhanced hydrocarbonate release during rewetting could dissolve carbonates, resulting in low Ca-P concentration in the sediment (Figure 5) and phosphorus mobilization (Baldwin and Mitchell, 2000). Accordingly, the presence of higher levels of organic compounds and enhanced biological activity increase respiratory rates, leading to elevated CO2 release and carbonic acid formation, and promoting carbonate dissolution and the release of associated P during subsequent hydroperiods (Kong et al., 2021).
On the other hand, the discriminant analysis reveals that HA-P is a determining factor in distinguishing the dry and flow data clusters due to its lower content in the dry period (Figure 7). In this regard, the position of the Eh (water) and Eh (sediments) vectors toward the dry period also indicates that this hydroperiod is marked by more oxidizing conditions which favor greater organic matter decomposition and consequently the release of organic-bound P (Datry et al., 2011; Mariluan et al., 2015; Abril et al., 2016). On the other hand, although the Flow hydroperiod presents lower Eh values (Table 1), this hydroperiod is still characterized by oxidizing conditions (i.e., Eh > 250 mV; Essington, 2015).
In fact, semi-arid regions are characterized by high evapotranspiration rates that promote oxidizing conditions throughout the year (Schaeffer-Novelli et al., 1990; Maia et al., 2005; de Aguiar et al., 2013). The DO vector position toward the flow period also corroborates the higher presence of oxygen and the prevalence of oxidizing conditions. These higher values may be associated with plant activity (Boulton et al., 2017) and lower water temperatures, as we obtained an average of 21.1°C for the flow and 25.6°C for the dry hydroperiods (see Hladzy et al. for more details). Furthermore, in IRES, the flow hydroperiod represents a moment when water flows through the river channel, transporting and exporting suspended materials and dissolved compounds (e.g., organic-bound P) to downstream ecosystems (Tzoraki et al., 2007; von Schiller et al., 2007, 2011; Obermann et al., 2009).
Additionally, we observed that the EX-P, which is the bioavailable P form, was significantly higher in the dry and flow periods compared to rewetting (Figure 5A). This indicates a greater potential of input of P to the aquatic system in the dry and flow hydroperiods. Total-P and orthophosphate-P in water were also similar for both dry and flow hydroperiods. Indeed, the discriminant analysis indicates a significantly inverse correlation between the vectors of HA-P and EX-P (Figure 7), indicating that this organic fraction is the main source of P during dry and flow periods.
On the other hand, the contents of Res-P did not differ significantly among the hydroperiods (Figure 5F), reflecting the recalcitrance and high resistance to the decomposition of this organic fraction (Kelleher and Simpson, 2006). However, the vector Res-P points toward the flow period and points in the opposite direction to the dry period, revealing a separation of these groups due to lower Res-P contents in the dry phase (Figure 7). Consequently, in the dry period, there is a higher decomposition of the Res-P fraction, which acts as a reservoir during periods of drought (Arias-Real et al., 2020; Granados et al., 2022).
Furthermore, the results for the CA-P fraction indicate that during the rewetting hydroperiod, this fraction acts as a source of P, while in dry and flow periods, it acts as a sink of P. In IRES, hypoxic conditions in the rewetting phase may cause rapid release of ammonia and phosphates from sediments (von Schiller et al., 2011; Skoulikidis et al., 2017). This condition may promote the dissolution of carbonates and the release of associated P to them (Kong et al., 2021). In the flow hydroperiod, there is an increasing input of inorganic material from adjacent ecosystems that combined with the low solubility of carbonates promotes the precipitation of CA-P even during wet periods (Dixon and Schulze, 2002; Skoulikidis et al., 2017). On the other hand, in the dry periods, the significantly higher pH values in both water and sediments (Table 1) decrease the solubility Ca and favor the carbonate precipitation, which favors P adsorption (Shen et al., 2011; Kim et al., 2016).
The Cruxati River is a tributary of the Mundaú River, which has an estuarine region that is part of a sustainable use conservation unit created by Ceará State Decree No. 24,414 on 29 March 1999, covering an area of 1,596 hectares (Greentec, 2021). The Mundaú River estuary is a biologically valuable refuge (Greentec, 2021). Similar to other estuaries worldwide, in many tropical semi-arid estuaries, P is a limiting nutrient to primary productivity (Lacerda et al., 2006, 2008; Barroso et al., 2016) and, consequently, for the functioning of the ecosystem.
The total input of P from natural sources in the Mundaú River basin is 122 t year−1 (Lacerda et al., 2008), similar to pristine environments (Golley et al., 1978; Burns, 2004). When compared to other estuaries in the Ceará State (e.g., 26.1 t year−1 for the Pacoti basin; 8.23 t year−1 for the Icapuí basin; and 1.10 t year−1 for the Malcozinhado) (Lacerda et al., 2008), these values are significant and relevant for nutrient loading into the estuaries. These highest values for the Mundaú basin may be attributed to the discharges from the Cruxati River (i.e., which is a tributary of the Mundaú River). The Mundaú River has a mean estimated flow of 15 m3 s−1 during the rainy season, dropping to <1 m3 s−1 during the dry periods (Molisani et al., 2006). Thus, the Crúxati River is an important propellant to water discharge, and P, consequently, in the rainy season, into the Mundaú River and the estuary.
Considering that P is limiting nutrients for primary production in semi-arid estuaries due to the dependence on water input from rivers (Barroso et al., 2016), the dynamics and P inputs from the Cruxati River are important to supply the estuarine region to guarantee the forests development and associated ecosystem services.
We investigated the role of hydrological dynamics on P bioavailability in an intermittent river in Northeast Brazil and the implications for nutrient cycling to adjacent and downstream ecosystems. We observed that seasonality influences the bioavailability of P in the studied intermittent river and that P bioavailability is strongly influenced by organic fractions. Seasonality in a semi-arid climate affects soil moisture, soil organic matter content, decomposition, and, consequently, the P release from riverine sediments to the water. Moreover, in river basins where the natural sources of P are predominant, wetting and drying cycles are important for the release and bioavailability of P. Hence, IRES plays an important role in the transport of nutrients across the watershed and reaching the estuaries, and its dynamic hydrological system may influence the nutrient discharge in estuaries, their ecosystem functioning, and the environmental services they provide.
The raw data supporting the conclusions of this article will be made available by the authors without undue reservation.
AS: Conceptualization, Data curation, Formal analysis, Investigation, Methodology, Project administration, Resources, Software, Supervision, Validation, Visualization, Writing – original draft, Writing – review & editing. HQ: Conceptualization, Data curation, Formal analysis, Investigation, Software, Validation, Visualization, Writing – original draft, Writing – review & editing. DO: Conceptualization, Methodology, Project administration, Software, Supervision, Validation, Writing – original draft, Writing – review & editing. GN: Formal analysis, Investigation, Methodology, Project administration, Software, Supervision, Validation, Visualization, Writing – original draft. TP: Formal analysis, Methodology, Project administration, Supervision, Validation, Writing – original draft, Writing – review & editing, Conceptualization, Data curation. PF: Formal analysis, Investigation, Methodology, Supervision, Validation, Writing – review & editing. DB: Conceptualization, Data curation, Validation, Writing – review & editing. CR: Conceptualization, Formal analysis, Funding acquisition, Investigation, Project administration, Resources, Software, Supervision, Validation, Visualization, Writing – original draft, Writing – review & editing. TF: Conceptualization, Formal analysis, Funding acquisition, Resources, Validation, Visualization, Writing – original draft, Writing – review & editing.
The author(s) declare financial support was received for the research, authorship, and/or publication of this article. The authors were financially supported by grants from AS Fundação Cearense de Apoio ao Desenvolvimento Científico e Tecnológico (FUNCAP) and CFR (Conselho Nacional de Desenvolvimento Científico e Tecnológico -CNPq, process PQ CNPQ 311627/2015-3) and funds from Programa de Apoio a Núcleos Emergentes PRONEM/FUNCAP/CNPq (PNE-0112-00026.01 0.00/16).
This study is part of the first author’s Master’s Programa de Pós-Graduação em Ecologia e Recursos Naturais from Universidade Federal do Ceará.
The authors declare that the research was conducted in the absence of any commercial or financial relationships that could be construed as a potential conflict of interest.
All claims expressed in this article are solely those of the authors and do not necessarily represent those of their affiliated organizations, or those of the publisher, the editors and the reviewers. Any product that may be evaluated in this article, or claim that may be made by its manufacturer, is not guaranteed or endorsed by the publisher.
Abril, M., Muñoz, I., and Menendez, M. (2016). Heterogeneity in leaf litter decomposition in a temporary Mediterranean stream during flow fragmentation. Sci. Total Environ. 553, 330–339. doi: 10.1016/j.scitotenv.2016.02.082
Acuña, V., Datry, T., Marshall, J., Dahm, D. C. N., Ginebreda, A., Mcgregor, G., et al. (2014). Why should we care about temporary waterways? Science 343, 1080–1081. doi: 10.1126/science.1246666
Acuña, V., Hunter, M., and Ruhí, A. (2017). Managing temporary streams and rivers as unique rather than second-class ecosystems. Biol. Conserv. 211, 12–19. doi: 10.1016/j.biocon.2016.12.025
Arias-Real, R., Muñoz, I., Gutiérrez-Cánovas, C., Granados, V., Lopez-Laseras, P., and Menéndez, M. (2020). Subsurface zones in intermittent streams are hotspots of microbial decomposition during the non-flow period. Sci. Total Environ. 703, 135485–135489. doi: 10.1016/j.scitotenv.2019.135485
Attygalla, N. W., Baldwin, D. S., Silvester, E., Kappen, P., and Whitworth, K. L. (2016). The severity of sediment desiccation affects the adsorption characteristics and speciation of phosphorus. Environ. Sci.: Processes Impacts 18, 64–71. doi: 10.1039/c5em00523j
Baldwin, D. S., and Mitchell, A. M. (2000). The effects of drying and re-flooding on the sediment and soil nutrient dynamics of lowland river–floodplain systems: a synthesis. Regul. Rivers: Res. Mgmt. 16, 457–467. doi: 10.1002/1099-1646(200009/10)16:5<457::AID-RRR597>3.0.CO;2-B
Baldwin, D. S., Mitchell, A. M., and Rees, G. N. (2000). The effects of in situ drying on sediment–phosphate interactions in sediments from an old wetland. Hydrobiologia 431, 3–12. doi: 10.1023/A:1004015019608
Barcellos, D., Queiroz, H. M., Nóbrega, G. N., Oliveira Filho, R. L. S., Sandra, T., Otero, X. L., et al. (2019). Phosphorus enriched effluents increase eutrophication risks for mangrove systems in northeastern Brazil. Mar. Pollut. Bull. 142, 58–63. doi: 10.1016/j.marpolbul.2019.03.031
Barroso, H. S., Becker, H., and Melo, V. M. M. (2016). Influence of river discharge on phytoplankton structure and nutrient concentrations in four tropical semiarid estuaries. Braz. J. Oceanogr. 64, 37–48. doi: 10.1590/S1679-87592016101406401
Bonada, N., Cañedo-Argüelles, M., Gallart, F., von Schiller, D., Fortuño, P., Latron, J., et al. (2020). Conservation and management of isolated pools in temporary rivers. Water 12, 01–24. doi: 10.3390/w12102870
Boulton, A. J., Rolls, R. J., Jaeger, K. L., and Datry, T. (2017). “Hydrological connectivity in intermittent Rivers and ephemeral streams” in Intermittents rivers and ephemeral streams: Ecology and management. eds. T. Datry, N. Bonada, and A. Boulton (London, United Kingdom: Elsevier Academic Press).
Brasil, Ministério do Meio Ambiente,. (2018). Atlas dos manguezais do Brasil. Brasília: Instituto Chico Mendes De Conservação da Biodiversidade.
Burns, D. A. (2004). The effects of atmospheric nitrogen deposition in the rocky mountains of Colorado and southern Wyoming, USA – a critical review. Environ. Pollut. 127, 257–269. doi: 10.1016/S0269-7491(03)00264-1
Carranza-Edwards, A., Rosales-Hoz, L., Urrutia-Fucugauchi, J., Sandoval-Fortanel, A., la Garza, E. M., and Cruz, R. L. S. (2005). Geochemical distribution pattern of sediments in an active continental shelf in southern Mexico. Cont. Shelf Res. 25, 521–537. doi: 10.1016/j.csr.2004.09.013
Cavalcante, H., Araújo, F., Noyma, N. P., and Becker, V. (2018). Phosphorus fractionation in sediments of tropical semiarid reservoirs. Sci. Total Environ. 619-620, 1022–1029. doi: 10.1016/j.scitotenv.2017.11.204
Chen, P.Y. (1977). Table of key lines in X-ray powder diffraction patterns of minerals in clays and associated rocks Bloomington, Indiana: Department of natural resources, Indiana Geological Survey.
Cornell, R.M., and Schwertmann, U. (2003). The Iron oxides: structure, reactions occurences and uses, Weinheim: Wiley-VCH.
Correa, M. M., Ker, J. C., Barrón, V., Fontes, M. P., Torrent, J., and Curi, N. (2008). Characterizing iron oxides from coastal and central plain soils. R. Bras. Ci. Solo 32, 1017–1031. doi: 10.1590/S0100-06832008000300011
Da-Peng, L., and Yong, H. (2010). Sedimentary phosphorus fractions and bioavailability as influenced by repeated sediment resuspension. Ecol. Eng. 36, 958–962. doi: 10.1016/j.ecoleng.2010.04.014
Darke, A. K., and Walbridge, M. R. (2000). Al and Fe biogeochemistry in a floodplain forest: implications for P retention. Biogeochemistry 51, 1–32. doi: 10.1023/A:1006302600347
Datry, T., Bonada, N., and Boulton, A. (2017). Intermittents rivers and ephemeral streams: Ecology and management. London, United Kingdom: Elsevier Academic Press.
Datry, T., Corti, R., Claret, C., and Philippe, M. (2011). Flow intermittence controls leaf litter breakdown in a French temporary alluvial river: the “drying memory”. Aquat. Sci. 73, 471–483. doi: 10.1007/s00027-011-0193-8
Datry, T., Corti, R., Foulquier, A., von Schiller, D., and Tockner, K. (2016). One for all, all for one: a global river research network. Eos Trans. Am. Geophys. Union 97, 2–8. doi: 10.1029/2016EO053587
Datry, T., Larned, S. T., and Tockner, K. (2014). Intermittent Rivers: a challenge for freshwater ecology. Bioscience 64, 229–235. doi: 10.1093/biosci/bit027
de Aguiar, P. F., El-Robrini, M., Freire, G. S. S., and de Carvalho, R. G. (2013). Morfodinâmica de praias dominadas por mesomarés na planície arenosa de Almofala, nw do ceará (Brasil). Pesqui. em Geociencias 40, 61–73. doi: 10.22456/1807-9806.40835
Dixon, J. B., and Schulze, D. G. (2002). Soil mineralogy with environmental applications. Madison, WI, USA: Soil Science Society of America.
Duarte, M. R. N., Pereira, T. M., Lima, P. F., Pereira, E. C. B., Lopes, F. B., and Rezende, C. F. (2021). Limnological dynamics in an artificial reservoir and intermittent river in the semi-arid region as a function of land use and occupation. Rev. Cienc. Agron. 52, 01–10.
Farias, R. L., Carvalho, L. K., and Medeiros, E. S. F. (2012). Distribution of Chironomidae in a semiarid Intermittent River of Brazil. Neotrop. Entomol. 41, 450–460. doi: 10.1007/s13744-012-0070-8
Fink, J. R., Inda, A. V., Tiecher, T., and Barrón, V. (2016). Iron oxides and organic matter on soil phosphorus availability. Cienc. E Agrotecnol. 40, 369–379. doi: 10.1590/1413-70542016404023016
Funceme – Fundação Cearense de Meteorologia e Recursos Hídricos (2018). Projetos e mapas. Fortaleza: Funceme.
Gee, G. W., and Bauder, J. W. (1986). “Particle-size analysis” in Methods of soil analysis: Part 1—Physical and mineralogical methods (Soil Science Society of America, American Society of Agronomy)
Godoy, M. D. P., and Lacerda, L. D. (2015). Mangroves response to climate change: a review of recent findings on mangrove extension and distribution. An. Acad. Bras. Cienc. 87, 651–667. doi: 10.1590/0001-3765201520150055
Golley, F. B., Mc Guiness, J. T., Clements, R. G., Child, G. I., and Duever, M. J. (1978). Ciclagem de minerais em um ecossistema de floresta tropical úmida. São Paulo: EDUSP.
Gómez, R., Arce, M. I., Baldwin, D. S., and Dahm, C. N. (2017). “Water Physicochemistry in intermittent Rivers and ephemeral streams” in Intermittents rivers and ephemeral streams: Ecology and management. eds. T. Datry, N. Bonada, and A. Boulton (London, United Kingdom: Elsevier Academic Press).
Gonçalves-Silva, M., Manna, L. R., Rodrigues-Filho, C. A. S., Teixeira, F. K., and Rezende, C. F. (2022). Effect of drying dynamics on the functional structure of a fish assemblage from an intermittent river network. Front. Environ. Sci. 10, 1–13. doi: 10.3389/fenvs.2022.903974
Granados, V., Arias-Real, R., Gutiérrez-Cánovas, C., Obrador, B., and Butturini, A. (2022). Multiple drying aspects shape dissolved organic matter composition in intermittent streams. Sci. Total Environ. 852, 158376–158378. doi: 10.1016/j.scitotenv.2022.158376
Greentec,. (2021). Plano de manejo da área de proteção ambiental do estuário do rio mundaú: Diagnóstico Socioambiental. Fortaleza: Greentec.
Hammer, Ø., Harper, D. A. T., and Ryan, P. D. (2001). PAST: Paleontological statistics software package for education and data analysis. Palaeontol. Electron. 4, 1–9.
Horppila, J. (2019). Sediment nutrients, ecological status and restoration of lakes. Water Res. 160, 206–208. doi: 10.1016/j.watres.2019.05.074
Kelleher, B. P., and Simpson, A. J. (2006). Humic substances in soils: are they really chemically distinct? Environ. Sci. Technol. 40, 4605–4611. doi: 10.1021/es0608085
Kim, B., Gautier, M., Simidoff, A., Sanglar, C., Chatain, V., Michel, P., et al. (2016). pH and eh effects on phosphorus fate in constructed wetland's sludge surface deposit. J. Environ. Manag. 183, 175–181. doi: 10.1016/j.jenvman.2016.08.064
Kong, M., Han, T., Chen, M., Zhao, D., Chao, J., and Zhang, Y. (2021). High mobilization of phosphorus in black-odor river sediments with the increase of temperature. Sci. Tot. Environ. 775, 145595–145508. doi: 10.1016/j.scitotenv.2021.145595
Lacerda, L. D., Molisani, M. M., Sena, D., and Maia, L. P. (2008). Estimating the importance of natural and anthropogenic sources on N and P emission to estuaries along the Ceará state coast NE Brazil. Environ. Monit. Assess. 141, 149–164. doi: 10.1007/s10661-007-9884-y
Lacerda, L. D., Vaisman, A. G., Maia, L. P., Silva, C. A. R., and Cunha, E. M. S. (2006). Relative importance of nitrogen and phosphorus emissions from shrimp farming and other anthropogenic sources for six estuaries along the NE Brazilian coast. Aquaculture 253, 433–446. doi: 10.1016/j.aquaculture.2005.09.005
Larned, S. T., Datry, T., Arscott, D. B., and Tockner, K. (2010). Emerging concepts in temporary‐river ecology. Freshw. Biol. 55, 717–738. doi: 10.1111/j.1365-2427.2009.02322.x
Leigh, C., Boulton, A. J., Courtwright, J. L., Fritz, K., May, C. L., Walker, R. H., et al. (2015). Ecological research and management of intermittent rivers: an historical review and future directions. Freshw. Biol. 61, 01–19.
Lillebo, A. I., Morais, M., Guilherme, P., Fonseca, R., Serafim, A., and Neves, R. (2007). Nutrient dynamics in Mediterranean temporary streams: a case study in Pardiela catchment (Degebe River, Portugal). Limnologica 37, 337–348. doi: 10.1016/j.limno.2007.05.002
Maia, L. P., Lacerda, L. D., Monteiro, L. H. U., and Souza, G. M. (2005). Estudo das Áreas de Manguezais do Nordeste do Brasil: Avaliação das áreas de manguezais dos Estados do Piauí, Ceará, Rio Grande do Norte, Paraíba e Pernambuco. Universidade Federal do Ceará, Instituo de Ciências do Mar Sociedade Internacional Para Ecossistemas de Manguezal - ISME-BR, Fortaleza – CE, 01-62 p.
Manly, B. F. J., and Alberto, J. A. N. (2016). Multivariate statistical methods: A primer CRC Press.
Mariluan, G. D., Villanueva, V. D., and Albarino, R. J. (2015). Leaf litter breakdown and benthic invertebrate colonization affected by seasonal drought in headwater lotic systems of Andean Patagonia. Hydrobiologia 760, 171–187. doi: 10.1007/s10750-015-2324-z
Martins, R. V., Paula Filho, F. J., and Rocha, C. A. S. (2007). Phosphorus geochemistry as a proxy of environmental estuarine processes at the Jaguaribe River Northeastern Brazil. Quim. Nova 30, 1208–1214.
Mckeague, J.A. (1978). Manual on soil sampling and methods of analysis Ottowa: Canadian Society of Soil Science, 212.
Mehra, O. P., and Jackson, M. L. (1960). Iron oxide removal from soils and clay by a dithionite-citrate system bulfered with sodium bicarbonate. Clay Clay Miner. 7, 317–327. doi: 10.1346/CCMN.1958.0070122
Melo, V. F., Fontes, M. P. F., Novais, R. F., Singh, B., and Schaefer, C. E. G. R. (2001). Iron and aluminum oxides of different Brazilian soils. R. Bras. Ci. Solo 25, 19–32. doi: 10.1590/S0100-06832001000100003
Melo, V. F., Novais, R. F., Schaefer, C. E. G. R., Fontes, M. P. F., and Singh, B. (2002a). Chemical and crystal properties of kaolinite and iron oxides in the Barreiras group sediments in Aracruz, Espírito Santo state. Brazil. R. Bras. Ci. Solo 26, 53–64. doi: 10.1590/S0100-06832002000100006
Melo, V. F., Novais, R. F., Schaefer, C. E. G. R., Fontes, M. P. F., and Singh, B. (2002b). Mineralogy of the sand, silt and clay fractions of Barreiras group sediments in Aracruz, Espírito Santo state. Brazil. R. Bras. Ci. Solo 26, 29–41. doi: 10.1590/S0100-06832002000100004
Molisani, M. M., Cruz, A. L. V., and Maia, L. P. (2006). Estimation of the freshwater river discharge to estuaries in Ceará state. Brazil. Arq. Ciên. Mar 39, 53–60.
Moro, M. F., Lughadha, E. N., and de Araújo, F. S. (2016). A Phytogeographical Metaanalysis of the semiarid Caatinga domain in Brazil. Bot. Rev. 82, 91–148. doi: 10.1007/s12229-016-9164-z
Murphy, J., and Riley, J. P. (1962). A modified single solution method for the determination of phosphate in natural waters. Anal. Chim. Acta 27, 31–36. doi: 10.1016/S0003-2670(00)88444-5
Nóbrega, G. N., Ferreira, T. O., Romero, R. E., Marques, A. G. B., and Otero, X. L. (2013). Iron and sulfur geochemistry in semi-arid mangroves soils (Ceará, Brazil) in relation to seasonal changes and shrimp farming effluents. Environ. Monit. Assess. 185, 7393–7407. doi: 10.1007/s10661-013-3108-4
Nóbrega, G. N., Otero, X. L., Macías, F., and Ferreira, T. O. (2014). Phosphorus geochemistry in a Brazilian semiarid mangrove soil affected by shrimp farm effluents. Environ. Monit. Assess. 186, 5749–5762. doi: 10.1007/s10661-014-3817-3
Nunes, F. C., Silva, E. F., and Vilas Boas, G. S. (2011). Grupo Barreiras: características, gênese e evidências de neotectonismo. Rio de Janeiro: Embrapa Solos, 31 p.
O’Connell, C. S., Ruan, L. S., and Whendee, L. (2018). Drought drives rapid shifts in tropical rainforest soil biogeochemistry and greenhouse gas emissions. Nat. Commun. 9, 1–9. doi: 10.1038/s41467-018-03352-3
Obermann, M., Rosenwinkel, K.-H., and Tournoud, M.-G. (2009). Investigation of first flushes in a medium-sized mediterranean catchment. J. Hydrol. 373, 405–415. doi: 10.1016/j.jhydrol.2009.04.038
Otero, X. L., De La Peña-Lastra, S., Pérez-Alberti, A., Ferreira, T. O., and Huerta-Diaz, M. A. (2008). Seabird colonies as important global drivers in the nitrogen and phosphorus cycles. Nat. Commun. 9, 1–8.
Paludan, C., and Jensen, H. S. (1995). Sequential extraction of phosphorus in freshwater wetland and lake sediment: significance of humic acids. Wetlands 15, 365–373. doi: 10.1007/BF03160891
Paludan, C., and Morris, J. T. (1999). Distribution and speciation of phosphorus along a salinity gradient in intertidal marsh sediments. Biogeochemistry 45, 197–221. doi: 10.1007/BF01106781
Parsons, C. T., Rezanezhad, F., O’Connell, D. W., and Van Cappellen, P. (2017). Sediment phosphorus speciation and mobility under dynamic redox conditions. Biogeosciences 14, 3585–3602. doi: 10.5194/bg-14-3585-2017
Penn, C. J., and Warren, J. G. (2009). Investigation phosphorus sorption onto kaolinite using isothermal titration calorimetry. Soil Sci. Sos. Am. J. 73, 560–568. doi: 10.2136/sssaj2008.0198
Perez, A. B. A., Santos, C., Sá, J. H. M., Arienti, P. F., and Chaffe, P. L. B. (2020). Connectivity of ephemeral and intermittent streams in a subtropical Atlantic Forest headwater catchment. Water 12, 01–15. doi: 10.3390/w12061526
Porto, M. M., Andrade, E. M., Costa, R. N. T., Lemos Filho, L. C. A., and Meireles, M. (2004). Identificação de bacias hidrográficas com características físicas similares no Estado do Ceará. Rev. Ciênc. Agron. 35, 17–25.
Primo, D. C., Menezes, R. S. C., and Silva, T. O. (2011). Substâncias húmicas da matéria orgânica do solo: uma revisão de técnicas analíticas e estudos no nordeste brasileiro. Scientia Plena 7, 01–13.
Queiroz, H. M., Ferreira, T. O., Barcellos, D., Nóbrega, G. N., Antelo, J., Otero, X. L., et al. (2021). From sinks to sources: the role of Fe oxyhydroxide transformations on phosphorus dynamics in estuarine soils. J. Environ. Manag. 278:111575. doi: 10.1016/j.jenvman.2020.111575
Queiroz, A. C. F., and Terra, B. F. (2019). Ecological drivers of fish metacommunities: environmental and spatial factors surpass predation in structuring metacommunities of intermittent rivers. Ecol. Freshw. Fish 29, 145–155. doi: 10.1111/eff.12502
Reimann, C., Filzmoser, P., Garrett, R. G., and Dutter, R. (2008). Statistical data analysis explained, statistical data analysis explained: applied environmental statistics with R. Chichester, UK: Jonh With & Sons Ltd.
Rodrigues Filho, C. A. S., Gurgel-Lourenço, R. C., Ramos, E. A., Novaes, J. L. C., Garcez, D. S., Costa, R. S., et al. (2020). Metacommunity organization in an intermittent river in Brazil: the importance of riverine networks for regional biodiversity. Aquat. Ecol. 54, 145–161. doi: 10.1007/s10452-019-09732-1
Schaeffer-Novelli, Y., Cintrón-Molero, G., Adaime, R. R., and de Camargo, T. M. (1990). Variability of mangrove ecosystems along the Brazilian coast. Estuaries 13, 204–218. doi: 10.2307/1351590
Schönbrunner, I. M., Preiner, S., and Hein, T. (2012). Impact of drying and re-flooding of sediment on phosphorus dynamics of river-floodplain systems. Sci. Total Environ. 432, 329–337. doi: 10.1016/j.scitotenv.2012.06.025
Schreckinger, J., Mutz, M., and Mendoza-Lera, C. (2022). When water returns: drying history shapes respiration and nutrients release of intermittent river sediment. Sci. Total Environ. 838, 155950–155908. doi: 10.1016/j.scitotenv.2022.155950
Shen, J., Yuan, L., Zhang, J., Li, H., Bai, Z., Chen, X., et al. (2011). Phosphorus dynamic: from soil to plant. Plant Physiol. 156, 997–1005. doi: 10.1104/pp.111.175232
Siregar, A., Kleber, M., Mikutta, R., and Jahn, R. (2005). Sodium hypochlorite oxidation reduces soil organic matter concentrations without affecting inorganic soil constituents. Eur. J. Soil Sci. 56, 481–490. doi: 10.1111/j.1365-2389.2004.00680.x
Skoulikidis, N., and Amaxidis, Y. (2009). Origin and dynamics of dissolved and particulate nutrients in a minimally disturbed Mediterranean river with intermittent flow. J. Hydrol. 373, 218–229. doi: 10.1016/j.jhydrol.2009.04.032
Skoulikidis, N. T., Vardakas, L., Amaxidis, Y., and Michalopoulos, P. (2017). Biogeochemical processes controlling aquatic quality during drying and rewetting events in a Mediterranean non-perennial river reach. Sci. Total Environ. 575, 378–389. doi: 10.1016/j.scitotenv.2016.10.015
Steward, A. L., von Schiller, D., Tockner, K., Marshall, J. C., and Stuart, E. B. (2012). When the river runs dry: human and ecological values of dry riverbeds. Front. Ecol. Environ. 10, 202–209. doi: 10.1890/110136
Tzoraki, O., Nikolaidis, N. P., Amaxidis, Y., and Skoulikids, N. T. H. (2007). Stream biogeochemical processes of a Temporary River. Environ. Sci. Technol. 41, 1225–1231. doi: 10.1021/es062193h
von Schiller, D., Acuña, V., Graeber, D., Martí, E., Ribot, M., Sabater, S., et al. (2011). Contraction, fragmentation and expansion dynamics determine nutrient availability in a Mediterranean forest stream. Aquat. Sci. 73, 485–497. doi: 10.1007/s00027-011-0195-6
von Schiller, D. V., Bernal, S., Dahm, C. N., and Martí, E. (2007). “Nutrient and organic matter dynamics in intermittent Rivers and ephemeral streams” in Intermittents rivers and ephemeral streams: Ecology and management. eds. T. Datry, N. Bonada, and A. Boulton (London, United Kingdom: Elsevier Academic Press), 135–160.
von Schiller, D., Bernal, S., and Martí, E. (2010). Technical note: a comparison of two empirical approaches to estimate in-stream nutrient net uptake. Biogeosci. Discuss. 7, 7527–7542.
von Schiller, D., Bernal, S., Sabater, F., and Martí, E. (2015). A round-trip ticket: the importance of release processes for in-stream nutrient spiraling. Freshw. Sci. 34, 20–30. doi: 10.1086/679015
von Schiller, D., Martí, E., and Riera, J. L. (2009). Nitrate retention and removal in Mediterranean streams bordered by contrasting land uses: a 15N tracer study. Biogeosciences 6, 181–196. doi: 10.5194/bg-6-181-2009
Keywords: fluvial environment, ecological functions, biogeochemical cycles, nutrients, phosphorus partitioning, isolated pools
Citation: da Silva AEB, Queiroz HM, de Oliveira DP, Nóbrega GN, Pereira TM, de Freitas Lima P, Barcellos D, Rezende CF and Ferreira TO (2024) Effects of hydrological dynamics in controlling phosphorus bioavailability in intermittent rivers and implications for estuaries. Front. Water. 6:1286968. doi: 10.3389/frwa.2024.1286968
Received: 01 September 2023; Accepted: 29 January 2024;
Published: 19 February 2024.
Edited by:
George P. Karatzas, Technical University of Crete, GreeceReviewed by:
Gunnar Lischeid, Leibniz Center for Agricultural Landscape Research (ZALF), GermanyCopyright © 2024 da Silva, Queiroz, de Oliveira, Nóbrega, Pereira, de Freitas Lima, Barcellos, Rezende and Ferreira. This is an open-access article distributed under the terms of the Creative Commons Attribution License (CC BY). The use, distribution or reproduction in other forums is permitted, provided the original author(s) and the copyright owner(s) are credited and that the original publication in this journal is cited, in accordance with accepted academic practice. No use, distribution or reproduction is permitted which does not comply with these terms.
*Correspondence: Diego Barcellos, ZGllZ28uYmFyY2VsbG9zQHVuaWZlc3AuYnI=
Disclaimer: All claims expressed in this article are solely those of the authors and do not necessarily represent those of their affiliated organizations, or those of the publisher, the editors and the reviewers. Any product that may be evaluated in this article or claim that may be made by its manufacturer is not guaranteed or endorsed by the publisher.
Research integrity at Frontiers
Learn more about the work of our research integrity team to safeguard the quality of each article we publish.