- 1Center for Limnology, University of Wisconsin-Madison, Madison, WI, United States
- 2Joseph J. Zilber School of Public Health, University of Wisconsin-Milwaukee, Milwaukee, WI, United States
- 3Center for Global Health, University of Oregon, Eugene, OR, United States
- 4Kisumu Research Center, Kenya Marine and Fisheries Research Institute, Kisumu, Kenya
Increased nutrient concentrations have been linked to water quality impairments across the world. In Lake Victoria, the second largest freshwater lake in the world, decades of eutrophication have challenged water quality management and led to conditions with compromising levels of cyanobacteria and cyanotoxins. Our study focuses on Winam Gulf, where a resurgence of water hyacinth has led to renewed challenges with navigation and anoxia, but also may present a local-scale opportunity for water quality improvements. Over the course of 1.5 years, we conducted three, in situ experiments to vary levels of water hyacinth density and determined its effects on lake water nutrient composition, biochemical parameters (chlorophyll a, cyanotoxins), and fecal indicators (fecal coliforms and Escherchia coli). While our study did not show overwhelming improvements in water quality with increasing water hyacinth density, we did find that small-scale removal of water hyacinth led to decreases in coliforms (F1, 11 = 11.14, p < 0.01), E. coli (F1, 11 = 22.95, p < 0.001), and total microcystins (F1, 9 = 3.47, p = 0.095). Throughout the experiment, the majority of samples collected had both E. coli and microcystin concentrations, notably exceeding World Health Organization guidelines for drinking water. Our data suggest that magnitude of scale, as well as season considerations such as rainfall and background level of water hyacinth, will strongly influence the phytoremediation potential of water hyacinth. The data encourages further exploration of remediation potential of native and introduced macrophytes.
1. Introduction
Inland waters across the world have been impacted by eutrophication. The impacts of increased concentrations of nitrogen and phosphorus are numerous (Carpenter et al., 1998; Smith, 2003; Diaz and Rosenberg, 2008; Glibert, 2017), particularly the formation and growth of cyanobacterial harmful algal blooms (cyanoHABs), which in turn, may release toxic compounds into aquatic resources. Humans, wildlife, or livestock may be exposed to these compounds through epidermal contact, ingestion of contaminated fish, or consumption of drinking water (Plaas and Paerl, 2021). These compounds, aka “cyanotoxins,” can have hepatotoxic, cytotoxic, or neurotoxic effects in both acute and chronic exposure scenarios (Buratti et al., 2017; Codd et al., 2020).
Cyanobacteria are capable of producing thousands of cyanotoxin variants within multiple classes including microcystins, cylindrospermopsins, saxitoxins, anatoxins, and a variety of other emerging toxin classes. The ubiquitous microcystins (MC) are thought to be the most prevalent class and are produced by a variety of species including Microcystis, Anabaena, Planktothrix, Nostoc, Anabaenopsis among others (Jungblut and Neilan, 2006). While more than 200 MC congeners have been detected in nature, microcystin-LR, microcystin-LA, microcystin-YR, and microcystin-RR (MC-LR, MC- LA, MC-YR, and MC-RR, respectively) are among the most commonly observed (Sharma et al., 2012; Puddick et al., 2014). The chemically stable structure of microcystins, seven amino acids covalently bonded in a ring, allows for their persistence in the environment. The congeners are designated by the two variable amino acids at positions 2 and 4. For example, microcystin- LR has leucine and arginine at position 2 and 4, respectively. Cylindrospermopsin is also common in many tropical and subtropical regions (McGregor and Fabbro, 2000; Lei et al., 2014). Both MC-LR and cylindrospermopsin are addressed in drinking water guidelines published by the World Health Organization, the United States Environmental Protection Agency as well as many other federal, local state and provincial public health and environmental agencies (Chorus and Welker, 2021).
Unfortunately, due to the complicated chemical structure and the common co-occurrence of stabilizing compounds, complete removal of cyanotoxins is difficult and often impossible to achieve in water treatment (Wang et al., 2021). Hence, preventative and mitigative measures of eutrophication in source waters may also be necessary (Roegner et al., 2014; Nwankwegu et al., 2019). Combating eutrophication is not a simple task. And, the impacts of eutrophication are seldom the only stressor facing aquatic ecosystems. Increased temperatures related to climate change may amplify cyanobacteria growth, shifts in hydrological patterns related to land use change or climate change may change stratification patterns, and the spread of invasive species can fundamental transform patterns in lake food webs (Paerl et al., 2011; Havel et al., 2015). Therefore, attempts to mitigate eutrophication must also take into consideration these other processes.
In this paper, we consider the dual impacts of eutrophication and water hyacinth, Eichhornia crassipes (Mart.) Solms, recently renamed Pontederia crassipes (Pellegrini et al., 2018), a common invasive species in many tropical and subtropical aquatic ecosystems outside of its native range in South America (Kriticos and Brunel, 2016; May et al., 2022), on water quality in Lake Victoria. Water hyacinth thrives in nutrient-rich waters (Coetzee and Hill, 2012) and its growth may also be promoted by increased temperatures due to climate change (You et al., 2014). The degradation of ecosystem services related to water hyacinth invasion are reviewed elsewhere (Ongore et al., 2018: Otieno et al., 2022), and include its impairment of boat navigation (Güereña et al., 2015), its creation of habitat for dangerous animals (e.g., snakes, hippos) and vectors of infectious diseases [e.g., mosquitoes (Chandra et al., 2006; Minakawa et al., 2012; Crossetti et al., 2019) and snails (Masifwa et al., 2001)], potential stimulation of greenhouse gas production (Kosten et al., 2016; though see Oliveira-Junior et al., 2018), and its support of anoxia in bottom waters. And, water hyacinth is costly to remove (Yan et al., 2017; Wainger et al., 2018).
The inability to easily remove water hyacinth from invaded waters has caused more researchers and practitioners to consider its potential benefits and how this might influence plant management (Patel, 2012; Yan et al., 2017; Janssen et al., 2021), particularly in relation to water quality and phytoremediation. In work from eutrophic lakes in China, windy conditions led to water hyacinth trapping cyanobacteria blooms (Zhang et al., 2019). The effect was also observed when water hyacinth were cultivated in in-lake enclosures (Qin et al., 2016). Based on these observations, water hyacinth growth could potentially concentrate cyanobacterial cells, which could be detrimental to water quality unless cyanobacterial growth or toxin production was lessened. Given that the high growth rates of water hyacinth also mean high nutrient and uptake and reduced nutrient concentrations in the lakes where it is found (Reddy and D'Angelo, 1990; Reddy et al., 1990; Oliveira-Junior et al., 2018; Ting et al., 2018); water hyacinth could lead to decreased cyanobacterial levels through competition for resources, as well as through shading (Zhou et al., 2014), despite the clumping mechanism. Further, water hyacinth can produce allelopathic compounds (Shanab et al., 2010). However, these compounds may be more detrimental to other phytoplankton in lake (i.e., green algae) than cyanobacteria (Pei et al., 2018). Therefore, it is not clear whether water hyacinth would compound or promote improved water quality through their interactions with cyanobacteria.
Lake Victoria, the second largest freshwater lake in the world, supports one of the most densely populated and rapidly growing regions in East Africa (Güereña et al., 2015). An economic valuation of ecosystem services from the lake suggest that it provides $400 million USD of goods annually (Lubovich, 2007). Eutrophication of Lake Victoria began in the 20th century and between the 1930's −1990's, water clarity as measured in Secchi disk depth declined from 5 m to <1 m (Klohn and Andjelic, 1997). Concomitantly, eutrophication has degraded many ecosystem services from the lake, particularly its use as drinking water (Mchau et al., 2019; Nantaba et al., 2020, 2021; Roegner et al., 2020). The establishment of water hyacinth to Lake Victoria was well-observed by 1989 and peaked in the northeastern Winam Gulf in 1998 (Albright et al., 2004; Wilson et al., 2007). Credited to the introduction of weevils and a fortuitous El Niño season, water hyacinth densities decreased by 2000 (Wilson et al., 2007), yet a massive re-emergence appeared in Winam Gulf in 2007 (potentially due to heavy rains in the preceding year; Fusilli et al., 2013). It is likely that water hyacinth will remain a potential nuisance in Lake Victoria for years to come (Hecky et al., 2010; May et al., 2022). Thus, understanding the trade-offs between water hyacinths' effects on ecosystem services is paramount to providing food, water, and transportation to millions of people.
To evaluate the interaction between eutrophication and water hyacinth on water quality parameters, we conducted a field experiment to manipulate water hyacinth density during different lake conditions (dry vs. wet season). We considered several categories of water quality features including nutrient parameters (nitrogen and phosphorus concentrations), biochemical parameters (chlorophyll a, cyanotoxins), and fecal indicators (fecal coliforms and E. coli). Given the potential diversity of cyanotoxins present in Winam Gulf (Roegner et al., 2020), we analyzed for 11 congeners of microcystins. We performed the experiment near a lakeside community and repeated it thrice for 2-week deployments.
2. Materials and methods
2.1. Field site description
The experiments were conducted within Winam Gulf (also known as Kavirondo Gulf or Nyanza Gulf), located within the Kenyan coast of Lake Victoria, on the shoreline of the Usoma Beach Management Unit (BMU) community (lat = −0.10506, long = −34.71816; Figure 1). Winam Gulf has a shallow bottom (e.g., avg. 10 m dept) and an area of 1,350 km2 (Romero and Alexander, 2006). The region has two rainy seasons, a long rainy season occurring from April–June and a short rainy season in November–December.
2.2. Experimental design
Water hyacinth cages were constructed of 1.5” PVC pipe with dimensions of 2 x 2 x 0.3 m. To prevent displacement of water hyacinth, wire fencing was wrapped in a double layer around the edges of the cage and fishing net was tied loosely to the bottom. The four treatment levels of water hyacinth were no addition, 1.5 plants m−2, 6.25 m−2, and 13 plants m−2. The densities were chosen to represent the range of natural densities found in the lake (Figure 2). Water hyacinth cages were anchored within 20 m of the shoreline and no more than 50 m apart for the duration of the experiment. The size of the enclosure, smaller than previous work (e.g., Qin et al., 2016) was designed to mimic a potential water hyacinth management approach that could be replicated by a Beach Management Unit or other community organization in their shoreline area, whether in Lake Victoria or elsewhere.
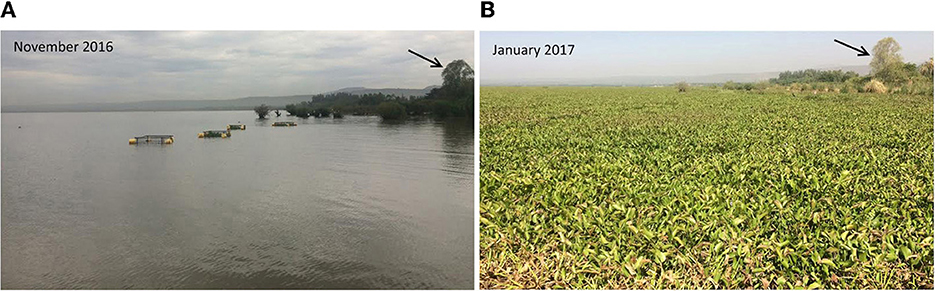
Figure 2. Comparison of ambient water hyacinth density in (A) November 2016 and (B) January 2017. Placement of experimental cages can be seen in (A). Note that while November 2016 is during one of the seasons investigated (WET16), (B) is not, but is included to represent typical high water hyacinth coverage conditions.
The experiment was repeated three times to capture variation in lake bloom and water hyacinth conditions. The first 4-week trial occurred in Oct/Nov 2016 (WET16), when water hyacinth coverage was low (Figure 2). The second trial occurred in Mar/April 2017 (DRY17), when water hyacinth coverage was high. The third trial occurred in Oct/Nov 2017 (WET17), when water hyacinth conditions were low. Each trial lasted 4 weeks; water sampling occurred weekly. Water sampling was conducted using a boat to access cages. Physicochemical parameters (temperature, specific conductivity) were monitored within each cage and the surrounding lake water using a multiparameter sonde (WQC-24, DKK-TOA, Cambridge, UK). Water samples were collected from surface waters in the interior of each cage and from the surrounding lake water using an acid-washed polyethylene bottle. Water samples were stored at 4oC until processed at the Kenyan Marine and Fisheries Research Institute (KMFRI) Research Center in Kisumu, Kenya.
2.3. Laboratory analyses
Water samples were processed for nutrient analyses, biological constituents, and cyanotoxins. For nutrient analyses, water was filtered (Whatman GF/C, nominal pore size = 1.2 μm) and processed for total dissolved nitrogen (TDN), total dissolved phosphorus (TDP), nitrate (-N), ammonium (-N), and soluble reactive phosphorus (SRP). Total dissolved N and TDP were determined colorimetrically following a persulfate digestion (Valderrama, 1981), while -N, -N, and SRP were determined using the sodium salicylate, indophenol blue, and ammonium molybdate methods, respectively. Chlorophyll a was analyzed on filters (Whatman GF/C, nominal pore size = 1.2 μm) according to standard methods (Baird and Bridgewater, 2017). Analysis of E. coli and total coliforms were performed with Colilert* (Idexx Laboratories), utilizing a Quanti-Tray System to deliver semiautomated quantification of the Most Probable Number (MPN) mode (Baird and Bridgewater, 2017) at the partner organization, SafeWater. Serial dilutions were performed with initial samples to select a 1:100 dilution. Dilutions and incubations were carried out within 24 h of collection. MPNs were determined after a 22–24-h incubation period. All analyses, except for the cyanotoxins, were conducted at the Kenya Marine and Fisheries Research Institute, Kisumu, Kenya.
As coliform bacteria include members that live outside of the gastrointestinal tract in the environment, false positives are possible, though the tests used in this study are widely used and recognized as indicators of fecal contamination in diverse water bodies and by public health and environmental agencies (Pisciotta et al., 2002). The particular test used also focuses on a more specific indicator of fecal contamination, Escherichia coli, which can grow at 44.5oC and expresses a particular enzyme that hydrolyzes 4-methylumbelliferyl-β-d-glucuronide (MUG) to form a fluorescent reaction visible with fluorescent light. Both false negatives and positives in this test are also possible in that some strains of E coli are MUG-negative, and other pathogenic strains can result in MUG-positive reactions. These tests were developed and validated in a narrow range of water types, despite being the standard of practice, so it is also possible that environmental bacteria in Lake Victoria waters could result in false positives, as well. However, they do serve as an overall relative proxy for human health risk (Ramoutar, 2020; Gibson et al., 2021).
The eleven MC congeners were analyzed by targeted liquid chromatography tandem mass spectrometry (LC-MS/MS). Certified reference standards (CRM) of MC-LR and [Dha7]MC-LR were purchased from the National Research Council of Canada Biotoxins program (Halifax, Nova Scotia) and CRM of MC-LA, MC-RR, MC-LF, MC-YR, MC-WR, MC-LY, MC-LW, MC-HtyR, and MC-HilR (all >95%) were purchased from Enzo Life Sciences (Farmingdale, NY).
Algal toxins were extracted from 30 ml lyophilized samples and analyzed by liquid chromatography tandem mass spectrometry as previously described (Bartlett et al., 2018). Lyophilized samples were resuspended in 1 ml 0.1% formic acid and subjected to three freeze-thaw cycles before adding 2 ml 100% methanol and sonicating in a water bath for 10 min. The final extract was centrifuged at 10,000 x g for 10 min to precipitate cellular debris prior to LC-MS/MS analysis. The toxins were separated by reverse phase chromatography and detected using scheduled multiple reaction monitoring with the mass spectrometer operating in positive mode. Product ion spectra, quantification limits, and representative chromatograms have been published previously (Beversdorf et al., 2017).
2.4. Statistical analysis
Prior to analysis of experimental data, we examined differences in lake water physicochemical condition among the experiments using a repeated measures analysis of variance (rmANOVA) of weekly temperature, specific conductivity, nutrient concentrations, or chlorophyll a concentrations from samples collected in the surrounding lake water. As samples from the surrounding lake for total coliforms and E. coli were lost due to a logistical oversight for DRY17 and WET17, the analysis of lake condition was made using all samples collected from within the cage. For WET16 when samples for total coliforms and E. coli were available in the surrounding lake water, the analysis of lake water condition included both the samples collected from within the cage and the surrounding lake water. Parameters directly relevant to human health concerns, the fecal parameters and microcystins, were further compared to recreational use or chronic exposure guidelines (USEPA, 2012; World Health Organization, 2020).
Next, we examined the impacts of water hyacinth density directly on lake nutrient concentrations and biological and fecal parameters. To consider impacts of water hyacinth density on lake nutrients and fecal indicators across experiments, we conducted a global rmANOVA for each nutrient and fecal indicator across all experimental data with cage and experiment as factors. To consider impacts of water hyacinth density on cyanobacteria, cyanotoxins, and fecal indicators, we also conducted rmANOVAs independently on each parameter for each experiment with cage as the factor. For all models, assumptions of normality and homogeneous variance were assessed by visual analysis of model residuals using a qq-plot. The rmANOVA were conducted using the nlme package in R (R Core Team, 2020; Pinheiro et al., 2022).
To consider the impacts of water hyacinth on cyanotoxins, we conducted a redundancy analysis using the relative abundance of cyanotoxins in each cage during each sampling event. The relative abundance was calculated using the decostand function with the argument “total” in R; the multivariate analysis was performed using the rda function from the vegan package in R (Oksanen et al., 2020; R Core Team, 2020).
3. Results
3.1. Lake water physicochemical and biological parameters
The field experiments were performed at the end of the dry season (DRY17) and during the short rainy season (WET16 and WET17). The density of water hyacinth at the shoreline also differed across experiments: during DRY17, water hyacinth coverage was so dense that it needed to be removed to achieve experimental treatments; during WET16 and WET17, water hyacinth coverage was lower so that it needed to be consolidated to achieve experimental treatments (Figure 2). Lake physicochemical characteristics were similar across the seasons, but TDN was higher during DRY17 (F2, 10 = 4.39, p < 0.05) and -N was lower during WET17 (F2, 10 = 5.79, p < 0.05; Table 1). While it did not vary significantly across experiments, chl a concentrations were highly variable, ranging over an order of magnitude. Other water quality parameters varied across experiments, as well (Figure 3). Biological parameters and fecal parameters followed similar patterns, though the two types varied. Chlorophyll a and microcystin concentrations were both lowest during DRY17, while fecal parameters were lowest during WET17. Mean total microcystins detected across all background lake water samples were 16 ± 23 μg L−1, with 77% of the samples overall exceeding the WHO guidance value for chronic MC exposure in drinking water. Mean total microcystins exceeded this guidance for two out of the three seasons (Figure 3), captured in this study. While the majority of samples did not exceed USEPA recreational guidelines for E. coli (Figure 3), 77% of the samples overall had detectable levels of E. coli.
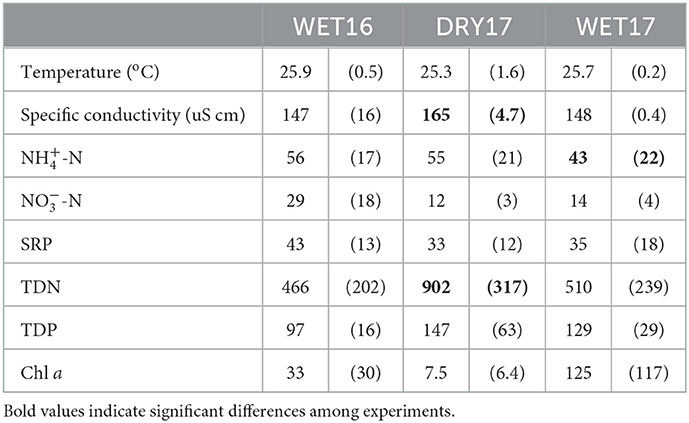
Table 1. Average (+/− standard deviation) physicochemical condition and nutrient concentrations (in ug L−1) during cage experiments.
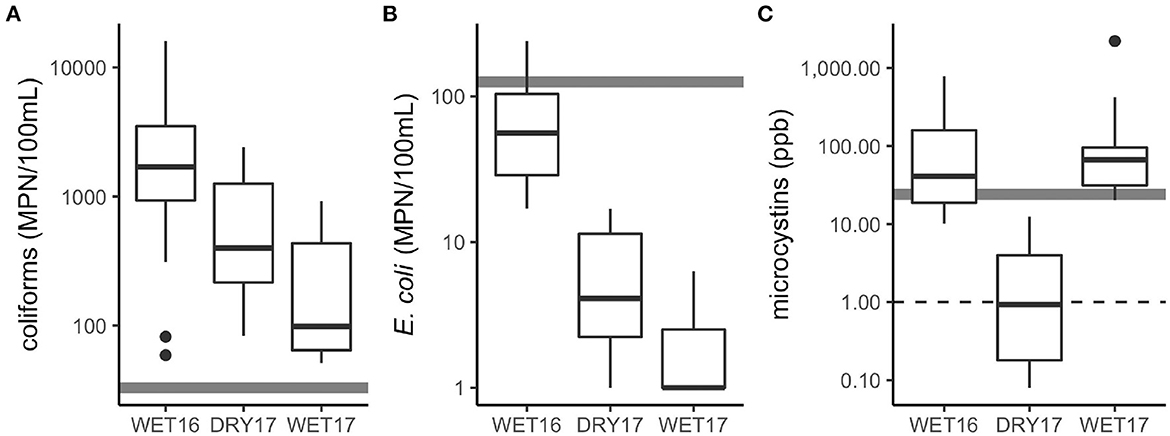
Figure 3. Concentration of select water quality parameters across experiments including (A) total coliforms, (B) E. coli, and (C) total microcystins. Gray, solid line indicates water quality threshold for recreational use (Coliforms, E. Coli–USEPA, 2012, microcystins–World Health Organization, 2020), black, dashed line indicates water quality threshold for chronic exposure (microcystins–World Health Organization, 2020).
3.2. Water hyacinth impacts on nutrients
While water hyacinth density did not significantly impact dissolved inorganic nutrient concentrations, it did impact the total dissolved portion. Total dissolved nutrient concentrations increased with water hyacinth density across all experiments (TDN: F1, 32 = 5.21, p < 0.05; TDP: F1, 33 = 18.23, p < 0.01), though the increases differed significantly for TDP among the experiments (F2, 33 = 14.50, p < 0.01; Figure 4).
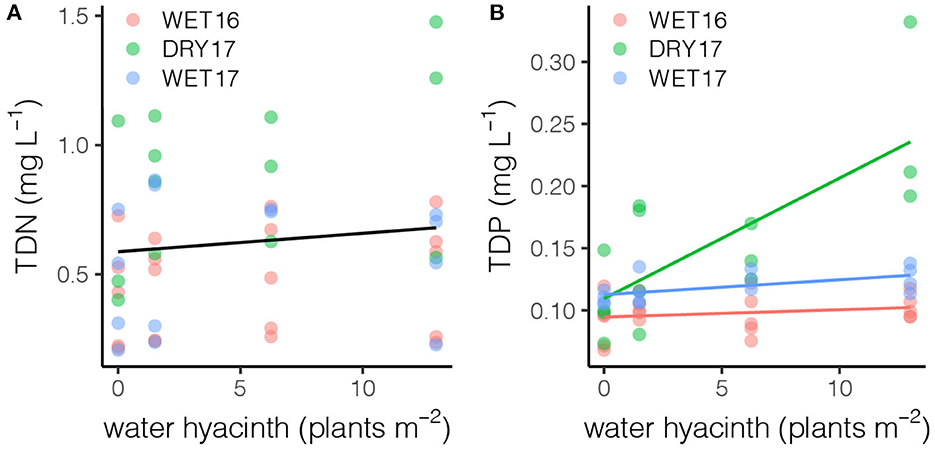
Figure 4. Concentrations of (A) total dissolved nitrogen increased across all experiments (p < 0.05), while (B) concentrations of total dissolved phosphorus increased most greatly in DRY17.
3.3. Water hyacinth impacts on fecal indicators
Water hyacinth did not influence fecal parameters strongly across all experiments (Figure 5). Across all experiments, water hyacinth tended to increase coliform concentrations (t = 1.94, p = 0.06), but the effect was only significant in DRY17 (F1, 11 = 11.14, p < 0.01; Figure 5A). The impact of water hyacinth on E. coli concentrations varied across experiments (F2, 32 = 7.46, p < 0.01); when experiments were considered individually, the impact of water hyacinth was strongly, but only, detected in DRY17 (F1, 11 = 22.95, p < 0.001; Figure 5B). However, while water hyacinth may augment of coliforms and E coli aggregation and/or growth, our cage manipulation data do not suggest a substantial shift in magnitude of human health risk. On the contrary, seasonal effects dictate the magnitude of scale for both (Figure 3).
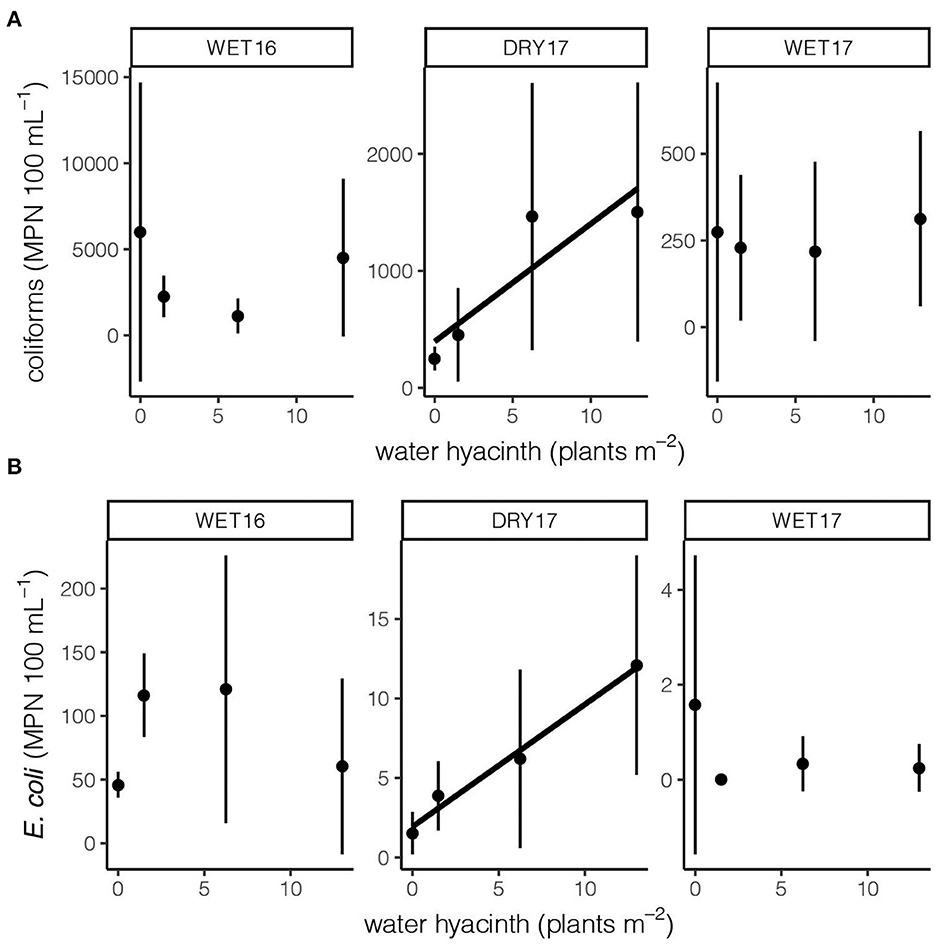
Figure 5. The impact of water hyacinth density on (A) total coliform concentrations and (B) E. coli concentrations were only evident during the March/April experiment (DRY17). The black line indicates a significant relationship between water hyacinth density and fecal parameter (p < 0.05). Note differences in scale among panels.
3.4. Water hyacinth impacts on cyanobacteria and cyanotoxins
Similar to fecal parameters, the impacts of water hyacinth density on biological parameters were not consistent across experiments (Figure 6). While we hypothesized that there would be either a strong positive or negative interaction between water hyacinth and cyanobacteria, we only found evidence of a positive interaction during WET17 (F1, 10 = 7.63, p < 0.05; Figure 6A), based on chlorophyll a concentration. We also only found evidence of a weak, positive influence of water hyacinth density on total microcystin concentration in DRY17 (F1, 9 = 3.47, p = 0.095; Figure 6B).
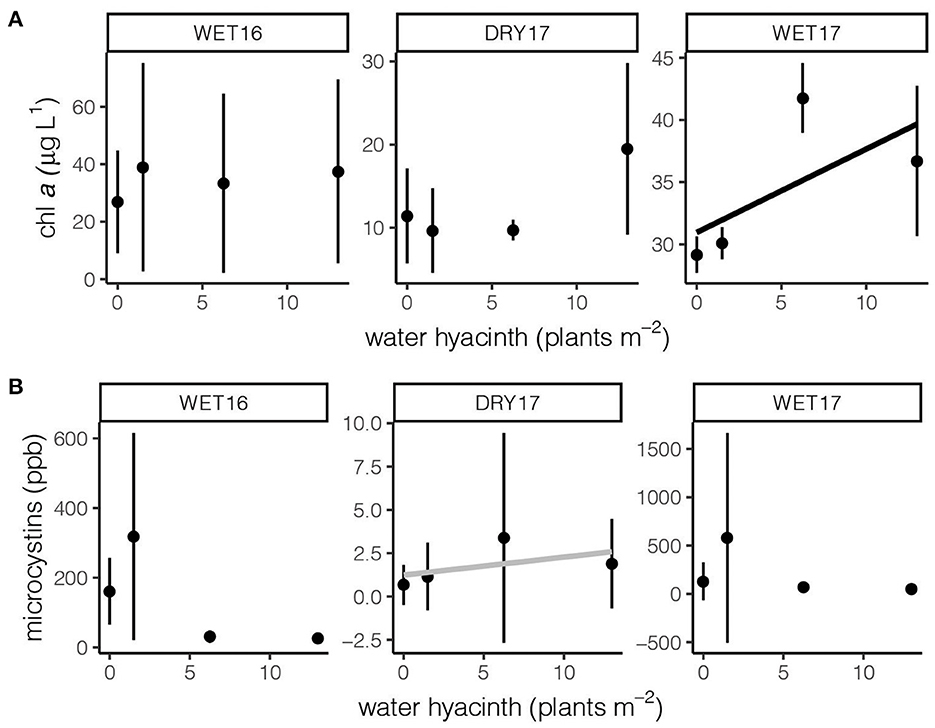
Figure 6. The impact of water hyacinth density on (A) chlorophyll a and (B) total microcystin concentration. The black line indicates a significant response (p < 0.05), the gray line indicates a nearly significant response (p < 0.10). Note differences in scale among panels.
All cyanotoxins screened for (ten congeners of microcystins and cylindrospermopsin) were detected in our samples during our experiment. There was more variation in cyanotoxin diversity based on when the experiment occurred than among cage treatments (Supplementary Figure 1), hence water hyacinth impacts based on treatment are not shown here, but can be found in the Supplementary material. The relative proportion of cyanotoxins varied among experiments (Figure 7). MC-LR and MC-YR were the most abundant across all experiments. MC-RR, MC-LY, MC-LA, and MC-WR were also detected at least once during each experiment, though, relative abundances were low (<10%; Figure 7). Notably, fewer cyanotoxins, both in terms of abundance and diversity, were found during DRY17. And, DRY17 was the only time the Cyl was detected (Figure 7).
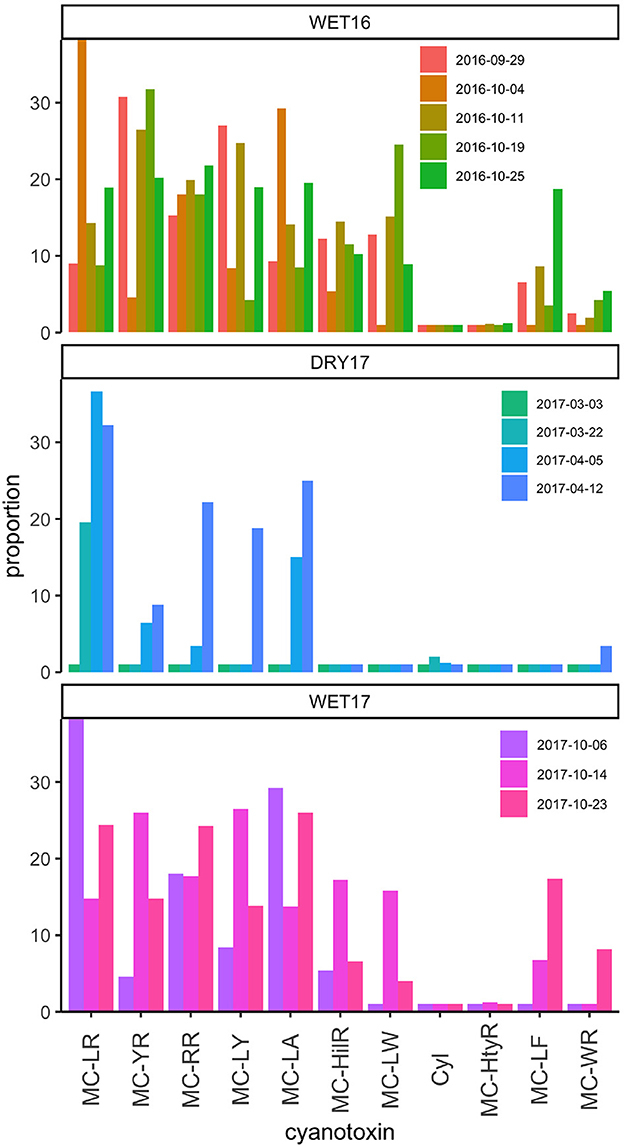
Figure 7. Relative proportion of cyanotoxins, microcystin congeners and cylindrospermopsin, during experiments. Microcystins are represented by congener designation MC-[variable amino acid position 2], MC-[variable amino acid position 4]. Changes in side groups are also denoted by position through standard chemical nomenclature. CYN denotes cylindrospermopsin.
4. Discussion
4.1. Small-scale physical removal of water hyacinth may lead to improved localized water quality during dense water hyacinth coverage
Our experiments add to the literature suggesting the complicated nature of managing water hyacinth to promote ecosystem services (Patel, 2012; Yan et al., 2017; Janssen et al., 2021). The ability for caged water hyacinth to promote improved water quality was highly dependent on the environmental context in which the experiment occurred and was only documented with a subset of the parameters we assessed. The greatest improvements to water quality came when water hyacinth coverage in Winam Gulf was highest, during DRY17 (Figure 5). Hence, the already present water hyacinth had to be removed, rather than added, to achieve experimental plant density treatments. Indeed, local fisher communities have been recruited for physical manual removal of water hyacinth as a widespread management technique and socioeconomic boon (Sikoyo and Goldman, 2007). Our results suggest that in addition to these benefits, creating a caged off region in the bay that could be used for water collection may also help minimize potential contamination from E. coli, coliforms, and possibly microcystins. However, biological, chemical, and even physical removal of water hyacinth can have unforeseen consequences when performed at large scale (Villamagna and Murphy, 2010) and can actually increase cyanobacterial cell counts or cyanotoxin production. Therefore, the size and extent of small clearings should be further studied and optimized for local conditions in other regions of Lake Victoria or other tropical lakes. And, any adoption of water hyacinth management for water quality improvements should be made in the context of other nuisances or threats that water hyacinth can pose (e.g., creation of habitat for dangerous animals and disease-spreading organisms, Masifwa et al., 2001; Chandra et al., 2006; Minakawa et al., 2012; Crossetti et al., 2019).
4.2. Potential impacts on magnitude of scale on in situ experiments
The lack of a positive response of water quality to increased water hyacinth density in WET16 and WET17 was unexpected. Research consistently shows that water hyacinth are successful at reducing nutrient concentrations and can filter suspended particles (Reddy et al., 1990; Patel, 2012, and others). In our experiment, with increasing water hyacinth density, we found no strong reduction of dissolved inorganic nutrients and, unexpectedly, an increase in total dissolved nitrogen concentrations. We did not analyze for particulate nutrient concentrations. These results are not likely due to limited growth of water hyacinth, as dissolved nutrients were detectable throughout the experiment. Instead, the results may be due to the spatial scale at which this work was conducted–the cages were on the scale of m2 in a gulf on the scale of km2. Hence, flow of lake water through the cages may mask any signal of changes to dissolved inorganic constituents. Alternatively, background concentrations of dissolved inorganic constituents may have been too high to detect a change. The detection of increased total dissolved nitrogen concentrations may be due to the continuous sloughing off of dissolved organics from the fibrous water hyacinth roots.
With respect to health parameters, seasonal rains may have a dilution effect through direct rainfall or pulse contribution through runoff to mute any discernable effect in small scale remediation efforts across cage manipulations. DRY17 data suggest that physical trapping or biofilms within water hyacinth may augment the presence of fecal bacteria of concern for humans recreating, working, or fetching water for household usage. Treatment recommendations prior to household usage (including filtration and chlorination) should be made, as well as for workers harvesting the water hyacinth for market purposes (see section 4.3). Similarly, the results from DRY17 suggest that when water hyacinth are in high density along the shoreline, there may be a slight reduction in human health risk of exposure to cyanotoxins during water collection if water is collected from open patches (either created manually or naturally) within the water hyacinth matrix. The data, as well as previous work with macrophytes (Romero-Oliva et al., 2014), suggest there likely is an ideal magnitude of scale of cyanotoxins for which this removal strategy is effective. Future work must both carefully exact the interaction between cyanobacteria and macrophytes, as well as delineate uptake of microcystin into macrophytes and relevant saturation points. In addition to water hyacinth, there are numerous native and introduced species of submerged, emergent, and floating macrophytes at Lake Victoria that could be utilized for their biofiltration or bioremediation potential.
4.3. Water quality concerns for human health in study area
Consistent with previous work in the region (Hecky et al., 2010; Mchau et al., 2019; Roegner et al., 2020), the elevated total coliforms, E. coli, and microcystin concentrations detected suggest lake water in household usage without prior treatment presents a formidable health risk for fisher communities. While fecal indicator levels did not consistently exceed recreational guidelines suggested by the USEPA, microcystins during WET16 and WET17 suggest that even recreational or labor exposures may be of risk for communities at the lake's edge, with increased risk for gastrointestinal illness and skin irritation from contact with bloom, accidental ingestion, or aerosolization. Data suggest baseline health risk and indicate water should be treated prior to any household use or drinking water intake. Handwashing and rinsing should be encouraged after manual labor in the lake.
While the study did not capture sufficient data or seasons to examine rainfall pattern effects, pulse flooding and heavy rainfalls may result in both a dilution effect and an augmentation through cellular lysis and release of dissolved microcystins into the water column. The data in this study represent total microcystins, including both intracellular and dissolved toxins, and thus represent total risk in the water column rather than microcystins present within healthy cyanobacterial cells, which may be higher during the dry season. While further work is needed to understand seasonal and flooding effects on both fecal indicators and microcystins, public health work in the region should extend to include ways for both households and workers to reduce their risk of exposures through personal protective approaches, hygiene, and treatment, regardless of season and exact lake water conditions. Emphasis should be made that disappearance of cyanobacterial cells may not eliminate risk of cyanotoxin exposure, and fecal indicators may vary widely depending upon recent climactic conditions. The predominant cyanobacterial species (Microcystis and Limnoraphis, formerly Anabaena spp,) and microcystin congeners detected in this study are consistent with previous work (Okello et al., 2010; Sitoki et al., 2012; Roegner et al., 2020). PCA analysis (Supplementary Figure 1) may indicate seasonal or climatic effects on congener production, and lends further argument for a closer examination of rainfall patterns in relationship with toxin production and overall human health risk. Recent work longitudinal analysis of the Great Lakes Basin has suggested a strong influence by meteorological patterns (Taranu et al., 2019) with MC-LR correlating strongly with strong winds, warm temperatures, and nutrient-rich conditions, while MC-LA predominated under intermediate winds, wetter, and nutrient-poor conditions.
Though Microcystis is presumed to be the predominant microcystin producer (Okello et al., 2010; Sitoki et al., 2012), no study has definitely examined MC producing genes in relationship with cyanotoxin concentrations in Kenyan waters. Cylindrospermopsin was detected in this study, albeit only in three samples and at trace 0.40 ± 0.03 μg/L. To our knowledge, this cyanotoxin has not been detected in Kenyan Lake Victoria waters, previously, and is not known to be produced by Microcystis spp., although Limnoraphis, Cylindrospermopsis spp., among others are capable. Recently, a co-occurrence of cylindrospermopsins and MCs was documented in Ugandan waters (Mchau et al., 2021). This narrow previous detection may reflect limited testing capability, rather than new occurrence, but either way, further investigation into diverse metabolites produced and the role of cyanobacterial species is in dire need of investigation to adequately protect human health. Cylindrospermopsin is both an acute and chronic liver toxicant, and a carcinogen (de la Cruz et al., 2013).
4.4. Conclusions and future work
Water hyacinth invasion and expansion in aquatic ecosystems across the world continues to provide management challenges. Our experiment provides evidence of the role of environmental conditions in management outcomes. In this case, we found the strongest impacts of water hyacinth density when water hyacinth were removed, rather than consolidated, for the experimental manipulations. While our results do suggest a course of action for very localized water quality improvements during high periods of water hyacinth abundance in Winam Gulf, further work is needed to discern its practicality for adoption by local communities, optimal size of enclosure units, and trade-offs with other nuisances or threats posed by water hyacinth. Additionally, our study results suggest that seasonality or climatic conditions in tropical settings may be important to cyanotoxin congener production. Given our growing understanding and concern about human health risks associated with different cyanotoxin compounds, future work should investigate the cause(s) of this variability in cyanobacteria metabolite production and presence in Lake Victoria and other tropical lakes.
Data availability statement
The original contributions presented in the study are included in the article/Supplementary material, further inquiries can be directed to the corresponding author.
Author contributions
JC and AR designed the study. ZO carried out the field experiments. CA oversaw data collection. AR and TM oversaw cyanotoxin analysis. JC analyzed and interpreted the results. All authors contributed to the writing and editing of the manuscript.
Funding
Funding for the experiment was provided by the University of Wisconsin's Global Health Initiative's Seed Grant program. JC was supported by the National Science Foundation (OIA-2019596).
Acknowledgments
The authors thank the Homa Bay Beach Management Unit for assistance in installing and tending the experimental cages, as well as research chemists, staff, and interns at Kenya Marine and Fisheries Research Institute.
Conflict of interest
The authors declare that the research was conducted in the absence of any commercial or financial relationships that could be construed as a potential conflict of interest.
Publisher's note
All claims expressed in this article are solely those of the authors and do not necessarily represent those of their affiliated organizations, or those of the publisher, the editors and the reviewers. Any product that may be evaluated in this article, or claim that may be made by its manufacturer, is not guaranteed or endorsed by the publisher.
Supplementary material
The Supplementary Material for this article can be found online at: https://www.frontiersin.org/articles/10.3389/frwa.2023.917837/full#supplementary-material
References
Albright, T. P., Moorhouse, T., and McNabb, T. (2004). The rise and fall of water hyacinth in Lake Victoria and the Kagera River Basin. J. Aquat. Plant Manag. 42, 73–84.
Baird, R., and Bridgewater, L. (2017). Standard Methods for the Examination of Water and Wastewater. 23rd edition. Washington, DC: American Public Health Association.
Bartlett, S. L., Brunner, S. L., Klump, J. V., Houghton, E. M., and Miller, T. R. (2018). Spatial analysis of toxic or otherwise bioactive cyanobacterial peptides in Green Bay, Lake Michigan. J Gt Lakes Res. 44, 924–933. doi: 10.1016/j.jglr.2018.08.016
Beversdorf, J. L., Weirich, A. C., Bartlett, L. S., and Miller, R. T. (2017). Variable cyanobacterial toxin and metabolite profiles across six eutrophic lakes of differing physiochemical characteristics. Toxins 9, 62. doi: 10.3390/toxins9020062
Buratti, F. M., Manganelli, M., Vichi, S., Stefanelli, M., Scardala, S., Testai, E., et al. (2017). Cyanotoxins: producing organisms, occurrence, toxicity, mechanism of action and human health toxicological risk evaluation. Arch. Toxicol. 91, 1049–1130. doi: 10.1007/s00204-016-1913-6
Carpenter, S. R., Caraco, N. F., Correll, D. L., Howarth, R. W., Sharpley, A. N., and Smith, V. H. (1998). Nonpoint pollution of surface waters with phosphorus and nitrogen. Ecol. Appl. 8, 559–568. doi: 10.1890/1051-0761(1998)008[0559:NPOSWW]2.0.CO;2
Chandra, G., Ghosh, A., Biswas, D., and Chatterjee, S. (2006). Host plant preference of Mansonia mosquitoes. J. Aquat. Plant Manag. 44, 142–144
Chorus, I., and Welker, M. (2021). Toxic Cyanobacteria in Water: a Guide to their Public Health Consequences, Monitoring and Management. Boca Raton, FL: Taylor and Francis, on behalf of the World Health Organization, Geneva, CH.
Codd, G. A., Testai, E., Funari, E., and Svirčev, Z. (2020). “Cyanobacteria, cyanotoxins, and human health,” in Water Treatment for Purification from Cyanobacteria and Cyanotoxins, eds A. E. Hiskia, T. M. Triantis, M. G. Antoniou, T. Kaloudis, and D. D. Dionysiou (Hoboken, NJ: Wiley), 37–68.
Coetzee, J. A., and Hill, M. P. (2012). The role of eutrophication in the biological control of water hyacinth, Eichhornia crassipes, in South Africa. Biocontrol 57, 247–261. doi: 10.1007/s10526-011-9426-y
Crossetti, L. O., Bicudo, D. D. C., Bini, L. M., Dala-Corte, R. B., Ferragut, C., and de Mattos Bicudo, C. E. (2019). Phytoplankton species interactions and invasion by Ceratium furcoides are influenced by extreme drought and water-hyacinth removal in a shallow tropical reservoir. Hydrobiologia 831, 71–85. doi: 10.1007/s10750-018-3607-y
de la Cruz, A. A., Hiskia, A., Kaloudis, T., Chernoff, N., Hill, D., Antoniou, M. G., et al. (2013). A review on cylindrospermopsin: the global occurrence, detection, toxicity and degradation of a potent cyanotoxin. Environ. Sci. Process. Impacts. 15, 1979–2003. doi: 10.1039/c3em00353a
Diaz, R. J., and Rosenberg, R. (2008). Spreading dead zones and consequences for marine ecosystems. Science. 321, 926–929. doi: 10.1126/science.1156401
Fusilli, L., Collins, M. O., Laneve, G., Palombo, A., Pignatti, S., and Santini, F. (2013). Assessment of the abnormal growth of floating macrophytes in Winam Gulf (Kenya) by using MODIS imagery time series. Int. J. Appl. E. Obs. 20, 33–41. doi: 10.1016/j.jag.2011.09.002
Gibson, C. J., Maritim, A. K., and Marion, J. W. (2021). Comparison of the ColiPlateTM kit with two common E. coli enumeration methods for water. Water 13, 1804. doi: 10.3390/w13131804
Glibert, P. M. (2017). Eutrophication, harmful algae and biodiversity—Challenging paradigms in a world of complex nutrient changes. Mar. Pollut. Bull. 124, 591–606. doi: 10.1016/j.marpolbul.2017.04.027
Güereña, D., Neufeldt, H., Berazneva, J., and Duby, S. (2015). Water hyacinth control in Lake Victoria: transforming an ecological catastrophe into economic, social, and environmental benefits. Sustain. Prod. Consum. 3, 59–69. doi: 10.1016/j.spc.2015.06.003
Havel, J. E., Kovalenko, K. E., Thomaz, S. M., Amalfitano, S., and Kats, L. B. (2015). Aquatic invasive species: challenges for the future. Hydrobiologia. 750, 147–170. doi: 10.1007/s10750-014-2166-0
Hecky, R. E., Mugidde, R., Ramlal, P. S., Talbot, M. R., and Kling, G. W. (2010). Multiple stressors cause rapid ecosystem change in Lake Victoria. Freshw. Biol. 55, 19–42. doi: 10.1111/j.1365-2427.2009.02374.x
Janssen, A. B., Hilt, S., Kosten, S., de Klein, J. J., Paerl, H. W., and Van de Waal, D. B. (2021). Shifting states, shifting services: linking regime shifts to changes in ecosystem services of shallow lakes. Freshw. Biol. 66, 1–12. doi: 10.1111/fwb.13582
Jungblut, A. D., and Neilan, B. A. (2006). Molecular identification and evolution of the cyclic peptide hepatotoxins, microcystin and nodularin, synthetase genes in three orders of cyanobacteria. Arch. Microbiol. 185, 107–114. doi: 10.1007/s00203-005-0073-5
Klohn, W., and Andjelic, M. (1997). Lake Victoria: A Case in International Cooperation. Rome: Food and Agriculture Organization.
Kosten, S., Piñeiro, M., de Goede, E., de Klein, J., Lamers, L. P., and Ettwig, K. (2016). Fate of methane in aquatic systems dominated by free-floating plants. Water Res. 104, 200–207. doi: 10.1016/j.watres.2016.07.054
Kriticos, D. J., and Brunel, S. (2016). Assessing and managing the current and future pest risk from water hyacinth, (Eichhornia crassipes), an invasive aquatic plant threatening the environment and water security. PLoS ONE 11, e0120054. doi: 10.1371/journal.pone.0120054
Lei, L., Peng, L., Huang, X., and Han, B. P. (2014). Occurrence and dominance of Cylindrospermopsis raciborskii and dissolved cylindrospermopsin in urban reservoirs used for drinking water supply, South China. Enviro. Monit. Assess. 186, 3079–3090. doi: 10.1007/s10661-013-3602-8
Lubovich, K. (2007). Cooperation and Competition: Managing Transboundary Water Resources in the Lake Victoria Region. Falls Church, VA: FESS, Foundation for Environmental Security and Sustainability.
Masifwa, W. F., Twongo, T., and Denny, P. (2001). The impact of water hyacinth, Eichhornia crassipes (Mart) Solms on the abundance and diversity of aquatic macroinvertebrates along the shores of northern Lake Victoria, Uganda. Hydrobiologia 452, 79–88. doi: 10.1023/A:1011923926911
May, L., Dobel, A. J., and Ongore, C. (2022). Controlling water hyacinth (Eichhornia crassipes (Mart.) Solms): a proposed framework for preventative management. Inland Waters. 12, 163–172. doi: 10.1080/20442041.2021.1965444
McGregor, G. B., and Fabbro, L. D. (2000). Dominance of Cylindrospermopsis raciborskii (Nostocales, Cyanoprokaryota) in Queensland tropical and subtropical reservoirs: implications for monitoring and management. Lake Reserv. 5, 195–205. doi: 10.1046/j.1440-1770.2000.00115.x
Mchau, G. J., Machunda, R., Kimanya, M., Makule, E., Gong, Y. Y., Mpolya, E., et al. (2021). First report of the co-occurrence of Cylindrospermopsin, Nodularin and Microcystins in the Freshwaters of Lake Victoria, Tanzania. Expos. Health 3, 185–194. doi: 10.1007/s12403-020-00372-7
Mchau, G. J., Makule, E., Machunda, E., Gong, Y. Y., and Kimanya, M. (2019). Harmful algal bloom and associated health risks among users of Lake Victoria freshwater: Ukerewe Island, Tanzania. J. Water Health. 17, 826–836. doi: 10.2166/wh.2019.083
Minakawa, N., Dida, G. O., Sonye, G. O., Futami, K., and Njenga, S. M. (2012). Malaria vectors in Lake Victoria and adjacent habitats in western Kenya. PLoS ONE 7, e32725. doi: 10.1371/journal.pone.0032725
Nantaba, F., Palm, W. U., Wasswa, J., Bouwman, H., Kylin, H., and Kuemmerer, K. (2021). Temporal dynamics and ecotoxicological risk assessment of personal care products, phthalate ester plasticizers, and organophosphorus flame retardants in water from Lake Victoria, Uganda. Chemosphere. 262, 127716. doi: 10.1016/j.chemosphere.2020.127716
Nantaba, F., Wasswa, J., Kylin, H., Palm, W. U., Bouwman, H., and Kümmerer, K. (2020). Occurrence, distribution, and ecotoxicological risk assessment of selected pharmaceutical compounds in water from Lake Victoria, Uganda. Chemosphere 239, 124642. doi: 10.1016/j.chemosphere.2019.124642
Nwankwegu, A. S., Li, Y., Huang, Y., Wei, J., Norgbey, E., Sarpong, L., et al. (2019). Harmful algal blooms under changing climate and constantly increasing anthropogenic actions: the review of management implications. 3 Biotech. 9, 449. doi: 10.1007/s13205-019-1976-1
Okello, W., Portmann, C., Erhard, M., Gademann, K., and Kurmayer, R. (2010). Occurrence of microcystin-producing cyanobacteria in Ugandan freshwater habitats. Environ. Toxicol. 25, 367–380. doi: 10.1002/tox.20522
Oksanen, J., Guillaume Blanchet, F., Friendly, M., Kindt, R., Legende, P., McGlinn, D., et al (2020). vegan: Community Ecology Package. R package version 2.5-7. Available online at: https://CRAN.R-project.org/package=vegan
Oliveira-Junior, E. S., Tang, Y., van den Berg, S. J., Cardoso, S. J., Lamers, L. P., and Kosten, S. (2018). The impact of water hyacinth (Eichhornia crassipes) on greenhouse gas emission and nutrient mobilization depends on rooting and plant coverage. Aquatic Botany 145, 1–9. doi: 10.1016/j.aquabot.2017.11.005
Ongore, C., Aura, M. C., Ogari, Z., Njiru, J. M., and Nyamweya, C. (2018). Spatial-temporal dynamics of water hyacinth, Eichhornia crassipes (Mart.), other macrophytes and their impact on fisheries in Lake Victoria, Kenya. J. Great Lakes Res. 44, 1273–1280. doi: 10.1016/j.jglr.2018.10.001
Otieno, D., Nyaboke, H., Nyamweya, S. C., Odoli, C. O., Aura, M. C., and Outa, N. O. (2022). Water Hyacinth (Eichhornia crassipes) infestation cycle, interactions with nutrients and aquatic biota in Winam Gulf, Lake Victoria, Kenya. Lakes Reserv. Sci. Policy Manag. Sustain. Use. 27, e12391. doi: 10.1111/lre.12391
Paerl, H. W., Hall, N. S., and Calandrino, E. S. (2011). Controlling harmful cyanobacterial blooms in a world experiencing anthropogenic and climatic-induced change. Sci. Total Environ. 409, 1739–1745. doi: 10.1016/j.scitotenv.2011.02.001
Patel, S. (2012). Threats, management and envisaged utilizations of aquatic weed Eichhornia crassipes: an overview. Rev. Environ. Sci. Bio/Technol. 11, 249–259. doi: 10.1007/s11157-012-9289-4
Pei, Y., Liu, L., Hilt, S., Xu, R., Wang, B., Li, C., et al. (2018). Root exudated algicide of Eichhornia crassipes enhances allelopathic effects of cyanobacteria Microcystis aeruginosa on green algae. Hydrobiologia 823, 67–77. doi: 10.1007/s10750-018-3696-7
Pellegrini, M. O., Horn, C. N., and Almeida, R. F. (2018). Total evidence phylogeny of Pontederiaceae (Commelinales) sheds light on the necessity of its recircumscription and synopsis of Pontederia L. PhytoKeys 108, 25–83. doi: 10.3897/phytokeys.108.27652
Pinheiro, J., Bates, D., and R Core Team (2022). nlme: Linear and Nonlinear Mixed Effects Models. R Package Version 3.1-157. Available online at: https://CRAN.R-project.org/package=nlme
Pisciotta, J. M., Rath, D. F., Stanek, P. A., Flanery, D. M., and Harwood, V. J. (2002). Marine bacteria cause false-positive results in the Colilert-18 rapid identification test for Escherichia coli in Florid waters. Appl. Environ. Microbiol. 68, 539–544. doi: 10.1128/AEM.68.2.539-544.2002
Plaas, H. E., and Paerl, H. W. (2021). Toxic cyanobacteria: A Growing threat to water and air quality. Environ. Sci. Technol. 55, 44–64. doi: 10.1021/acs.est.0c06653
Puddick, J., Prinsep, M. R., Wood, S. A., Kaufononga, S. A., Cary, S. C., and Hamilton, D. P. (2014). High levels of structural diversity observed in microcystins from microcystic CAWBG11 and characterization of six new microcystin congeners. Mar. Drugs 12, 5372–5395. doi: 10.3390/md12115372
Qin, H., Zhang, Z., Liu, H., Li, D., Wen, X., Zhang, Y., et al. (2016). Fenced cultivation of water hyacinth for cyanobacterial bloom control. Environ. Sci. Pollut. Res. 23, 17742–17752. doi: 10.1007/s,11356-016-6799-6
R Core Team (2020). R: A Language and Environment for Statistical Computing. R Foundation for Statistical Computing, Vienna, Austria. Available online at: https://www.R-project.org.
Ramoutar, S. (2020). The use of Colilert-18, Colilert and Enterolert for the detection of faecal coliform, Escherichia coli and Enterococci in tropical marine waters, Trinidad and Tobago. Reg. Stud. Marine Sci. 40, 101490. doi: 10.1016/j.rsma.2020.101490
Reddy, K. R., Agami, M., and Tucker, J. C. (1990). Influence of phosphorus on growth and nutrient storage by water hyacinth (Eichhornia crassipes (Mart.) Solms) plants. Aquat. Bot. 37, 355–365. doi: 10.1016/0304-3770(90)90021-C
Reddy, K. R., and D'Angelo, E. M. (1990). Biomass yield and nutrient removal by water hyacinth (Eichhornia crassipes) as influenced by harvesting frequency. Biomass 21, 27–42. doi: 10.1016/0144-4565(90)90045-L
Roegner, A., Sitoki, L., Weirich, C., Corman, J., Owage, D., Umami, M., et al. (2020). Harmful algal blooms threaten the health of peri-urban fisher communities: a case study in Kisumu Bay, Lake Victoria, Kenya. Expos. Health 12, 835–848. doi: 10.1007/s12403-019-00342-8
Roegner, A. F., Brena, B., González-Sapienza, G., and Puschner, B. (2014). Microcystins in potable surface waters: toxic effects and removal strategies. J. Appl. Toxicol. 34, 441–457. doi: 10.1002/jat.2920
Romero, J. R., and Alexander, R. (2006). “Management implications of the physical limnological studies of Rusinga Channel and Winam Gulf in Lake Victoria,” in Proceedings of the 11th World Lakes Conference, eds E. Odada and D. O. Olago (Nairobi, Kenya: Ministry of Water and Irrigation).
Romero-Oliva, C. S., Contardo-Jara, V., Block, T., and Pflugmacher, S. (2014). Accumulation of microcystin congeners in different aquatic plants and crops—a case study from lake Amatitlán, Guatemala. Ecotoxicol. Environ. Saf. 102, 121–128. doi: 10.1016/j.ecoenv.2014.01.031
Shanab, S. M., Shalaby, E. A., Lightfoot, D. A., and El-Shemy, H. A. (2010). Allelopathic effects of water hyacinth [Eichhornia crassipes]. PLoS ONE. 5, e13200. doi: 10.1371/journal.pone.0013200
Sharma, V. K., Triantis, T. M., Antoniou, M. G., He, X., Pelaez, M., Han, C., et al. (2012). Destruction of microcystins by conventional and advanced oxidation processes: a review. Separ. Purific. Technol. 91, 3–17. doi: 10.1016/j.seppur.2012.02.018
Sikoyo, G. M., and Goldman, L. (2007). Assessing the assessments: case study of an emergency action plan for the control of water hyacinth in Lake Victoria. Int. J.Water Resour. Develop. 23, 443–455. doi: 10.1080/07900620701488521
Sitoki, L., Kurmayer, R., and Rott, E. (2012). Spatial variation of phytoplankton composition, biovolume, and resulting microcystin concentrations in the Nyanza Gulf (Lake Victoria, Kenya). Hydrobiologia 691, 109–122. doi: 10.1007/s10750-012-1062-8
Smith, V. H. (2003). Eutrophication of freshwater and coastal marine ecosystems a global problem. Environ. Sci. Pollut. Res. 10, 126–139. doi: 10.1065/espr2002.12.142
Taranu, Z. E., Pick, F. R., Creed, I. F., Zastepa, A., and Watson, S. B. (2019). Meteorological and nutrient conditions influence microcystin congeners in freshwaters. Toxins 11, 620. doi: 10.3390/toxins11110620
Ting, W. H. T., Tan, I. A. W., Salleh, S. F., and Wahab, N. A. (2018). Application of water hyacinth (Eichhornia crassipes) for phytoremediation of ammoniacal nitrogen: a review. J. Water Process Eng. 22, 239–249. doi: 10.1016/j.jwpe.2018.02.011
USEPA (2012). Recreational Water Quality Criteria. Office of Water, United States Environmental Protection Agency; Washington, D.C. Report No. 820-F-12-058. Available online at: https://www.epa.gov/sites/default/files/2015-10/documents/rwqc2012.pdf (accessed December 20, 2022).
Valderrama, J. C. (1981). The simultaneous analysis of total nitrogen and total phosphorus in natural waters. Mar. Chem. 10, 109–122.
Villamagna, A. M., and Murphy, B. R. (2010). Ecological and socio-economic impacts of invasive water hyacinth (Eichhornia crassipes): a review. Freshw. Biol. 55, 282–298. doi: 10.1111/j.1365-2427.2009.02294.x
Wainger, L. A., Harms, N. E., Magen, C., Liang, D., Nesslage, G. M., McMurray, A. M., et al. (2018). Evidence-based economic analysis demonstrates that ecosystem service benefits of water hyacinth management greatly exceed research and control costs. PeerJ. 6, e4824. doi: 10.7717/peerj.4824
Wang, S., Jiao, Y., and Rao, Z. (2021). Selective removal of common cyanotoxins: a review. Environ. Sci. Pollut. Res. 28, 28865–28875. doi: 10.1007/s11356-021-13798-6
Wilson, J. R., Ajuonu, O., Center, T. D., Hill, M. P., Julien, M. H., Katagira, F. F., et al. (2007). The decline of water hyacinth on Lake Victoria was due to biological control by Neochetina spp. Aquat. Bot. 87, 90–93. doi: 10.1016/j.aquabot.2006.06.006
World Health Organization (2020). Cyanobacterial Toxins: Microcystins. Background Document for Development of WHO Guidelines for Drinking-Water Quality and Guidelines for Safe Recreational Water Environments. Geneva: World Health Organization(WHO/HEP/ECH/WSH/2020.6). Available online at: https://apps.who.int/iris/handle/10665/338066 (accessed December 20, 2022).
Yan, S. H., Song, W., and Guo, J. Y. (2017). Advances in management and utilization of invasive water hyacinth (Eichhornia crassipes) in aquatic ecosystems–a review. Crit. Rev. Biotechnol. 37, 218–228. doi: 10.3109/07388551.2015.1132406
You, W., Yu, D., Xie, D., Yu, L., Xiong, W., and Han, C. (2014). Responses of the invasive aquatic plant water hyacinth to altered nutrient levels under experimental warming in China. Aquat. Biol. 119, 51–56. doi: 10.1016/j.aquabot.2014.06.004
Zhang, Y., Liu, H., Yan, S., Wen, X., Qin, H., Wang, Z., et al. (2019). Phosphorus removal from the hyper-eutrophic Lake Caohai (China) with large-scale water hyacinth cultivation. Environ. Sci. Pollut. Res. 26, 12975–12984. doi: 10.1007/s11356-019-04469-8
Keywords: eutrophication, water hyacinth, cyanotoxins, microcystins, cylindrospermopsin, Lake Victoria
Citation: Corman JR, Roegner A, Ogari Z, Miller TR and Aura CM (2023) Local-scale impacts of water hyacinth on water quality in a hypereutrophic lake. Front. Water 5:917837. doi: 10.3389/frwa.2023.917837
Received: 11 April 2022; Accepted: 12 January 2023;
Published: 02 February 2023.
Edited by:
Giovanni Laneve, Sapienza University of Rome, ItalyReviewed by:
Temitope O. Sogbanmu, University of Lagos, NigeriaGagan Matta, Gurukul Kangri Vishwavidyalaya, India
Copyright © 2023 Corman, Roegner, Ogari, Miller and Aura. This is an open-access article distributed under the terms of the Creative Commons Attribution License (CC BY). The use, distribution or reproduction in other forums is permitted, provided the original author(s) and the copyright owner(s) are credited and that the original publication in this journal is cited, in accordance with accepted academic practice. No use, distribution or reproduction is permitted which does not comply with these terms.
*Correspondence: Jessica R. Corman, amNvcm1hbjNAdW5sLmVkdQ==
†Present address: Jessica R. Corman, School of Natural Resources, University of Nebraska-Lincoln, Lincoln, NE, United States