- 1Department of Aquatic Ecology and Environmental Biology, Institute for Water and Wetland Research, Radboud University Nijmegen, Nijmegen, Netherlands
- 2Institute for Environmental Sciences, RPTU Kaiserslautern-Landau, Landau, Germany
- 3Groupe de Recherche Interuniversitaire en Limnologie, Département des Sciences Biologiques, Université du Québec à Montréal, Montréal, QC, Canada
Freshwater ecosystems, including lakes, wetlands, and running waters, are estimated to contribute over half the natural emissions of methane (CH4) globally, yet large uncertainties remain in the inland water CH4 budget. These are related to the highly heterogeneous nature and the complex regulation of the CH4 emission pathways, which involve diffusion, ebullition, and plant-associated transport. The latter, in particular, represents a major source of uncertainty in our understanding of inland water CH4 dynamics. Many freshwater ecosystems harbor habitats colonized by submerged and emergent plants, which transport highly variable amounts of CH4 to the atmosphere but whose presence may also profoundly influence local CH4 dynamics. Yet, CH4 dynamics of vegetated habitats and their potential contribution to emission budgets of inland waters remain understudied and poorly quantified. Here we present a synthesis of literature pertaining CH4 dynamics in vegetated habitats, and we (i) provide an overview of the different ways the presence of aquatic vegetation can influence CH4 dynamics (i.e., production, oxidation, and transport) in freshwater ecosystems, (ii) summarize the methods applied to study CH4 fluxes from vegetated habitats, and (iii) summarize the existing data on CH4 fluxes associated to different types of aquatic vegetation and vegetated habitats in inland waters. Finally, we discuss the implications of CH4 fluxes associated with aquatic vegetated habitats for current estimates of aquatic CH4 emissions at the global scale. The fluxes associated to different plant types and from vegetated areas varied widely, ranging from−8.6 to over 2835.8 mg CH4 m−2 d−1, but were on average high relative to fluxes in non-vegetated habitats. We conclude that, based on average vegetation coverage and average flux intensities of plant-associated fluxes, the exclusion of these habitats in lake CH4 balances may lead to a major underestimation of global lake CH4 emissions. This synthesis highlights the need to incorporate vegetated habitats into CH4 emission budgets from natural freshwater ecosystems and further identifies understudied research aspects and relevant future research directions.
1 Introduction
Freshwater ecosystems, including wetlands, lakes, reservoirs, rivers, and streams, play an important role in global carbon cycling and are estimated to contribute roughly 50% to global methane (CH4) emissions: 149 ± 15 Tg CH4 yr−1 for wetlands and 151 (± 73.0) Tg CH4 y−1 for lakes (Saunois et al., 2020; Rosentreter et al., 2021). Estimates of CH4 emissions are, however, highly variable, and freshwater systems represent a major source of uncertainty in the global CH4 budget (Saunois et al., 2020). Within freshwater systems, there are several major sources of uncertainty, that include the large temporal and spatial heterogeneity that characterizes CH4 fluxes, as well as system and geographic biases in the extant data bases (Rosentreter et al., 2021). One additional source of uncertainty is CH4 emissions from vegetated habitats within inland waters. Aquatic plants, and in particular emergent macrophytes, which colonize many inland waters, have been known for a relatively long time to be potentially important sources of CH4 (Carmichael et al., 2014). Studies focusing on single systems have shown that fluxes from vegetated areas can disproportionately contribute to the total CH4 emissions, up to 78% even when the only a limited area of the entire system is vegetated (Dacey and Klug, 1979; Holzapfel-Pschorn et al., 1986; Larmola et al., 2004; Desrosiers et al., 2022), yet these habitats are seldom accounted for in aquatic CH4 budgets, whether at the ecosystem, regional, or global scales. A notable exception is the recent study on emergent vegetation in arctic-boreal lakes which indicates that accounting for the vegetation increases CH4 emission estimates by 21% (Kyzivat et al., 2022). The general omission of vegetated habitats in aquatic CH4 budgets is partly a consequence of the fact that the distribution of aquatic vegetation is itself highly heterogeneous, the information on greenhouse gas dynamics associated to this aquatic vegetation is fragmented, biased geographically and by ecosystem type, and the approaches used are often not comparable. As a result, the contribution of aquatic vegetation to whole ecosystem CH4 budgets remains largely unquantified, and this is perhaps one of the largest sources of uncertainty in the current global inland water CH4 budget.
Clearly, the contribution of vegetated areas to total system emissions from inland waters depends on the extent of vegetation coverage on the one hand and the impact of plants on CH4 dynamics on the other. CH4 emissions reported from vegetated sites vary widely—being higher or lower—than those of surrounding unvegetated sites (see an overview in e.g., Kosten et al., 2016), although they tend to be in the upper range of aquatic fluxes (Kyzivat et al., 2022). This large variability has been attributed to differences in the influence on CH4 production, CH4 oxidation, and CH4 transport (Fritz et al., 2011; Dean et al., 2018; Grasset et al., 2018). The influence of vegetation on these processes varies among plant species (Holzapfel-Pschorn et al., 1986; Yoshida et al., 2014; Villa et al., 2020) and depends on a variety of variables, including plant biomass (particularly below-ground biomass; e.g., Liu et al., 2019), CH4 concentration around the roots (Struik et al., 2022), plant growth dynamics (Kankaala et al., 2003), plant tissue composition (Grasset et al., 2019), sediment temperature (Kankaala et al., 2004), sediment composition (Kankaala et al., 2005), water depth (Ding et al., 2002) and herbivory (Dingemans et al., 2011), all of which vary seasonally, leading to seasonal differences in CH4 emissions. This long list highlights the complexity of the mechanistic underpinnings of plant impacts on CH4 fluxes and the challenges associated with understanding and predicting these processes, to be able to accurately extrapolate and upscale CH4 emissions from aquatic vegetated areas across ecosystems at regional and even global scales.
Insight into the quantitative effect of aquatic vegetation on CH4 fluxes is in part hampered by the wide range of different approaches that have been used to assess plant effects on CH4 emissions, which vary in the type of fluxes they include. Some studies have focused exclusively on the flow of CH4 through the plant tissue—mostly in emergent plants (Sanders et al., 2007) -, whereas other studies have assessed CH4 fluxes more generally within vegetated habitats. Within the latter, a few have made the distinction between direct and indirect plant effects; some have included the effect of plants on ebullition (Davidson et al., 2018; Desrosiers et al., 2022), others have explicitly excluded ebullition (e.g., Petruzzella et al., 2015). This diversity of approaches complicates comparison among studies, and is also reflected in the rather inconsistent use of the term “plant-mediated” or “plant-associated” fluxes to indicate both direct plant-mediated emissions (direct transport by the plants), and emissions that occur in habitats that are dominated by plants, which include both direct fluxes from plants and other effects plants have on CH4 emissions in the habitat. While both definitions are linguistically correct, the pathways and implications are very different. To avoid ambiguities, we use the term plant-associated CH4 fluxes generically to denote CH4 fluxes occurring in vegetated habitats, and these include direct plant-mediated fluxes and other CH4 emission pathways that occur in these vegetated habitats. In this work, we aim to advance the understanding of the effect of aquatic vegetation on the CH4 dynamics and emissions in inland waters, to advance the quantification of CH4 emissions from vegetated habitats, and to identify knowledge gaps. We conducted a semi-quantitative literature review, aiming at (i) providing an overview of the different ways aquatic vegetation can influence CH4 production, consumption, and transport (Section 2), (ii) providing an overview of approaches applied to measure CH4 fluxes associated to aquatic plants and the determine the potential effect of aquatic vegetation on CH4 dynamics (Section 2), and (iii) summarizing the extant data on CH4 fluxes of vegetated areas and its variation (Section 3). Finally, we discuss the implications of CH4 fluxes from vegetated habitats in inland waters for current global CH4 estimates (Section 4).
2 The impact of vegetation on CH4 dynamics
This section summarizes the effects of aquatic vegetation on CH4 production, oxidation, and transport (Figure 1). For a more elaborate discussion on plant effects on CH4 emissions in different ecosystems, we refer to Bastviken et al. (2023). More details on the physiology of plant CH4 transport can be found in Vroom et al. (2022).
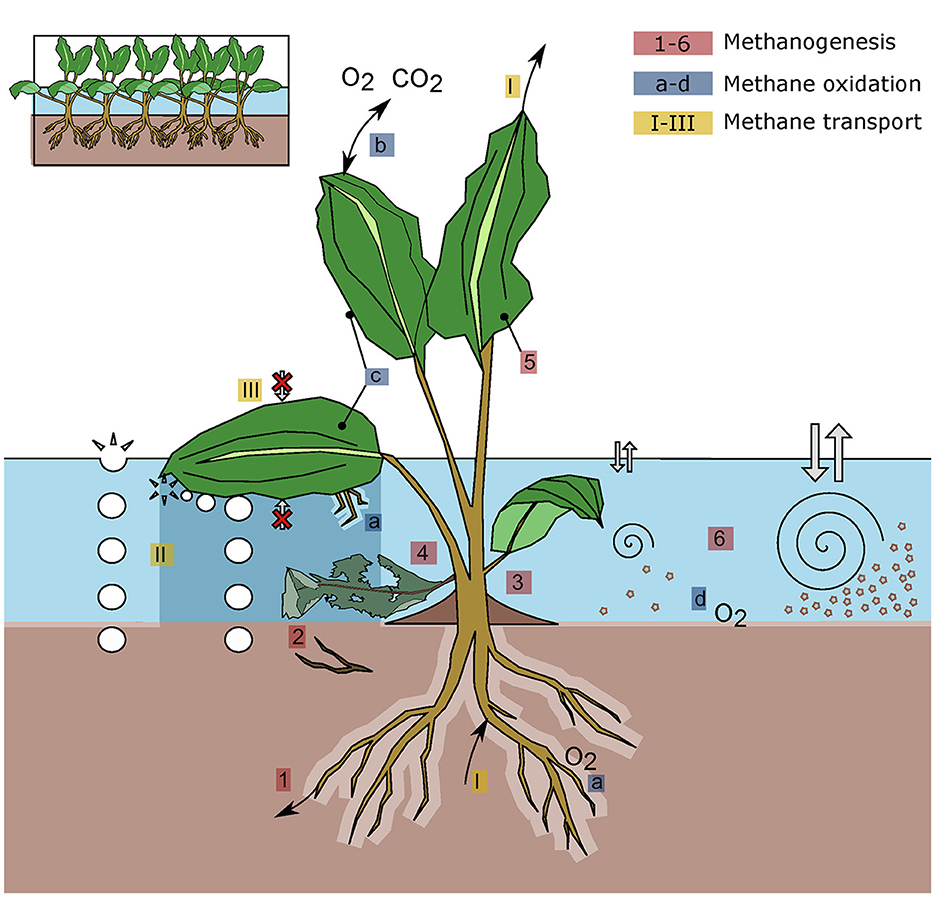
Figure 1. Plant-mediated processes that influence methane (CH4) emissions from freshwater systems. The depicted plant represents a hybrid of emergent, floating, and submerged vegetation to illustrate the main processes of these three different functional groups. The water layer is divided into an oxic (light blue) and anoxic (dark blue) zone. The processes illustrated are related to CH4 production (1-6, red), CH4 oxidation (a-d, blue) and CH4 transport (I-III, yellow). Plant-associated effects on CH4 production include the provision of organic carbon through root exudates (1) and decaying organic matter (2), sediment accumulation due to reduced water flow (3), dissolved carbon release followed by oxic methanogenesis in the water column (4), CH4 production within the plant (5) and reduced sediment resuspension (6). CH4 oxidation is affected by radial oxygen loss (ROL) in the root zone (a) potentially related to photosynthesis (b), CH4 oxidation by epiphytic and endophytic methanotrophs (c), and oxygen (O2) availability in the oxic water or sediment layer (d). Plants can alter the transport of CH4 by serving as a bypass between the anoxic sediment and the atmosphere (I), by impeding the movement of bubbles from the water to the atmosphere (II), and by reducing water-atmosphere gas exchange (III).
2.1 CH4 production
CH4 is for a large part a final product of organic matter decomposition by methanogenic archae in anaerobic environments and is produced mainly in sediments (Bastviken, 2009). Plant-associated effects on CH4 production are described below and include the provision of organic carbon through root exudates and decaying organic matter and sediment accumulation due to reduced water flow. In addition, plants release dissolved carbon in the water column—potentially followed by oxic methanogenesis—and CH4 production may take place within the plant (Figure 1).
Decaying plant matter can enhance CH4 production in the sediment by promoting anoxia, and by providing organic substrates for methanogens (Webster and Benfield, 1986; Segers, 1998; Marinho et al., 2015). The intensity of methanogenesis depends on plant species and composition of the decaying matter. In a study of a boreal lake, for instance, Lemna trisulca detritus produced twice as much CH4 per gram biomass as Phragmites australis detritus (Kankaala et al., 2003). Bottles incubation experiments showed that CH4 production was positively related to a plant's water content, and negatively related to its C:N and C:P ratio (Grasset et al., 2018, 2019). Root exudates also form an important pool of labile carbon substrates, such as acetate potentially fueling methanogenesis (Joabsson et al., 1999; Ström et al., 2003). Acetate exudation per gram root can vary considerably between plant species. For instance, Eriophorum vaginatum may release over seven times more acetate than Carex rostrata and Juncus effusus (Ström et al., 2005). The release of labile organic carbon could fuel methanogenesis not only in the sediment, but also in the oxic water column, as has been suggested for algae in rivers and lakes (Bogard et al., 2014; Donis et al., 2017; Mei et al., 2020). Besides enhancing methanogenesis, root exudates may suppress CH4 oxidation by competition for electron acceptors, as was found in a thermokarst bog (Turner et al., 2020).
In addition to producing organic matter that can serve as a precursor for methanogenesis, aquatic vegetation can increase sedimentation rates by reducing water flow velocity and turbulence (Sand-Jensen and Pedersen, 1999; Clarke, 2002). In particular, fine organic sediments form an optimal environment for methanogens due to high sediment surface area and a high potential for microbial biofilm formation (Sanchez et al., 1994; Grasset et al., 2019; Bodmer et al., 2020). Methanogenesis may therefore be enhanced in vegetated areas of water bodies due to high sedimentation rates (Kankaala et al., 2005; Crawford and Stanley, 2016).
An additional CH4 production pathway could be oxic, non-enzymatic production of CH4 within plants (Keppler et al., 2006; Bruhn et al., 2012). This process has been observed in living and litter material of several terrestrial plant species and is likely related to the oxic chemical breakdown of pectin when a plant is exposed to UV radiation or other stressors (Keppler et al., 2008; Wang et al., 2011). This process has been suggested to take place in macrophytes as well (Hilt et al., 2022).
2.2 CH4 oxidation
Aerobic CH4 oxidation (i.e., consumption), performed by methane-oxidizing bacteria, is most extensive at the oxic–anoxic interface where both O2 and CH4 are available (Bastviken, 2009). Plants affect CH4 oxidation by radial oxygen loss (ROL) in the root zone (potentially related to photosynthesis), CH4 oxidation by epiphytic and endophytic methanotrophs, and O2 availability in the oxic water or sediment layer (Figure 1).
To overcome anoxia-related problems in waterlogged soils, many vascular wetland plants transport oxygen from their shoots to below-ground tissues. O2 is transported within gas-filled aerenchyma via diffusion and/or pressurized flow (Armstrong, 1980; Colmer, 2003; Vroom et al., 2022). This process supplies O2 to roots and rhizomes and can also result in radial O2 loss (ROL) to the rhizosphere. ROL enables the detoxification of harmful substances (e.g., sulphide), enhances nutrient uptake, and alters the microbial community structure (Galand et al., 2005; Robroek et al., 2015). The increased O2 availability in the rhizosphere enhances aerobic CH4 oxidation (Aben et al., 2022). The importance of ROL in regulating CH4 emissions is strongly dependent on plant species and developmental stage (van der Nat and Middelburg, 1998). For example, Ström et al. (2005) showed that Carex-dominated vegetation in peat monoliths emitted more than twice as much CH4 as Eriophorum-dominated vegetation due to a lower degree of rhizospheric CH4 oxidation in Carex. In a Patagonian bog colonized by cushion plants (Astelia pumila and Donatia fascicularis), intense CH4 oxidation as a result of ROL suppressed CH4 emissions completely (Fritz et al., 2011). ROL can also occur in floating plants and adventitious roots, reducing dissolved CH4 concentrations in the water column (Visser et al., 2000; Kosten et al., 2016; Fonseca et al., 2017).
Besides enhancing CH4 oxidation, ROL can suppress methanogenesis, as O2 availability results in the oxidation of the alternative electron acceptors nitrate, manganese (IV), iron (III), and sulphate. The use of these alternative electron acceptors in organic matter decomposition is energetically favorable compared to methanogenesis. O2 release in the rhizosphere can, therefore, indirectly repress methanogenesis, depending on the pool of alternative electron acceptors and the magnitude of ROL (Laanbroek, 2010).
The presence of vegetation can alter O2 concentrations not only in the sediment but also in the water column. Floating vegetation forms a barrier at the water-air interface, limiting gas exchange. This reduces O2 diffusion into the water column and dissolved CH4 release to the atmosphere (Attermeyer et al., 2016; Kosten et al., 2016). Additionally, light limitation by overlying vegetation can reduce O2 supply by primary production, thereby potentially reducing CH4 oxidation (King, 1990). On the other hand, the lower light intrusion may also increase CH4 oxidation by preventing light inhibition (Thottathil et al., 2018). The physical barrier formed by floating plants also captures bubbles, enhancing their residence time and potential dissolution and subsequent oxidation of CH4 (Kosten et al., 2016).
In addition to impacting microbial processes through alteration of their physical environments, certain plant species also harbor methanotrophs directly on or within their tissues. Epiphytic methanotrophs have been found on the shoots of a range of emergent and submerged plant species (Heilman and Carlton, 2001; Sorrell et al., 2002; Yoshida et al., 2014; Ávila et al., 2019). Endophytic methanotrophs have been found in Sphagnum mosses and several vascular peatland plants (Raghoebarsing et al., 2005; Stepniewska et al., 2018). Iguchi et al. (2019) showed that duckweed colonizing freshwater lakes are not only inhabited by methanotrophs, but the duckweed plant actually has an enhancing effect on methane oxidation. Duckweed may stimulate methanotrophic growth, presumably by contributing certain metabolites (Iguchi et al., 2019).
2.3 CH4 transport
Besides affecting CH4 production and consumption processes, aquatic vegetation plays a key role in CH4 transport. On the one hand, plants can affect two main pathways of CH4 emission from freshwater ecosystems to the atmosphere diffusion across the air-water interface and ebullition (escape of CH4 in gas bubbles directly from the sediments to the atmosphere) (Figure 1) On the other hand, emergent rooted aerenchymous plants represent a direct conduit for CH4 from the sediment to the atmosphere, allowing CH4 to bypass oxidation in oxic sediment and water layers (Chanton and Dacey, 1991; Vroom et al., 2022). This has also been observed for floating plants rooting in the sediments of shallow waters (Oliveira-Junior et al., 2018). As CH4 transport is a side effect of ROL, it can be affected by plant functional traits and the presence of pressurized flow (Brix, 1993). Similar to ROL, plant-mediated CH4 transport is affected by, among other factors, temperature, light intensity, plant tissue porosity, ambient rates of photosynthesis, and stomatal conductance, and may therefore vary diurnally and seasonally (Yavitt and Knapp, 1995; Whiting and Chanton, 1996; Kim et al., 1999). Damage or herbivory may result in enhanced CH4 transport: CH4 emissions of damaged (clipped) plants increased to 160% of control values for Carex aquatilis (Schimel, 1995), and similar results were observed for herbivore-induced damage in a wetland plant community (Dingemans et al., 2011). This “chimney effect” has in fact been the focus of the plant impact on CH4 fluxes and has been measured in a relatively large number of emergent and floating plant species. Although measured rates vary widely as a function of both the species and the environmental condition, overall, the results agree that emergent vegetation has the capacity to sustain extremely high fluxes of CH4 to the atmosphere. For example, it is estimated that direct plant-mediated transport may contribute 55 to 85% to total CH4 fluxes in peatlands (Dean et al., 2018). Analysis of paired measurements in open water and emergent vegetation in a compiled dataset of 66 lakes furthermore indicated that on a per-area basis emissions from emergent vegetation is 6.1 times higher than from open water (Kyzivat et al., 2022). However, aquatic vegetation not only affects the emission intensity, but also may affect the emission pathway. Plant-mediated transport may reduce sediment CH4 concentrations to such an extent that it reduces ebullition. For example, in an experimental set-up with Phragmites australis, plant clipping resulted in a 5 to 10-fold increase in ebullition, whereas the total emission, including plant-associated transport, was about 1.8 times higher in the presence of intact plants (van den Berg et al., 2020).
2.4 Effect of plants on overall CH4 emissions: a whole habitat perspective
The effect of aquatic plants on the whole habitat CH4 budget is at present difficult to predict, because as discussed in the sections above, the presence of plants influences CH4 dynamics and emissions in multiple ways, and in addition, there have been relatively few studies that have assessed the complete CH4 budget of vegetated habitats in freshwaters. The common denominator of studies that have assessed total CH4 fluxes from vegetated habitats in lakes and wetlands is that these tend to be high relative to adjacent, non-vegetated sites and to open water, pelagic habitats (Kankaala et al., 2004; Larmola et al., 2004; Wang et al., 2006; Marinho et al., 2015; Jeffrey et al., 2019; Desrosiers et al., 2022). It is interesting to note that whereas total CH4 emissions tend to be consistently high in vegetated habitats, the pathways that contribute to these emissions are highly variable. For example, Jeffrey et al. (2019) reported an average annual contribution of plant-mediated fluxes to total habitat CH4 emissions of around 59% in a tropical wetland dominated by floating lilies, with diffusion and ebullition each accounting for roughly 20%, and studies in temperate vegetated lake habitats have also reported dominance of direct plant-mediated fluxes (Chu et al., 2014). A recent study showed that in vegetated habitats in a boreal lake colonized by Typha sp., ebullitive and diffusive fluxes were suppressed because their dense root mats acted as both a barrier for the vertical flux of bubbles and also greatly suppressed turbulence, yet CH4 emissions from the habitat were nevertheless extremely high, driven by direct plant-mediated CH4 transport (Desrosiers et al., 2022). In contrast, in an adjacent vegetated habitat in the same lake dominated by the rooted, floating Brassenia sp., the authors reported extremely high ebullitive fluxes, presumably driven by plant-enhanced sediment methanogenesis, with direct plant-mediated fluxes contributing much less to total CH4 fluxes in this habitat (Desrosiers et al., 2022). Ebullitive flux was reported to be of the same magnitude as plant-mediated emissions at the outermost Phragmites reed band but contributed much less within the Phragmites bed in a boreal lake (Kankaala et al., 2004), and Marinho et al. (2015) also reported elevated rates of CH4 ebullition in littoral habitats of tropical lake colonized by the emergent Schoenoplectus californicus, yet total CH4 emissions were nevertheless dominated by diffusion. The pattern that emerges is that the dominant pathway of CH4 emission may vary greatly between ecosystems and even between habitats within the same ecosystem, as a function of the plant functional type and density, sediment and water properties, and other environmental factors, and also seasonally with plant succession and climatic factors. Direct plant-associated transport often dominates the CH4 budget in these habitats, but not always depending on the season and dominant plant type. Modeling and upscaling total CH4 fluxes from vegetated freshwater habitats is therefore challenging, involving multiple pathways and complex interactions, yet this is important because regardless of what the main pathways of emission are, there is increasing evidence that vegetated habitats in lakes, wetlands and rivers, tend to contribute disproportionately to whole ecosystem emissions (Juutinen et al., 2003; Desrosiers et al., 2022). The large influence of vegetated habitats on overall CH4 fluxes extends to seasons when plants may not be present. For example, the vegetated littoral contributed disproportionately (66–78%) to winter CH4 emissions from two boreal lakes (Larmola et al., 2004).
2.5 Methods to assess CH4 fluxes of vegetated areas
A wide variety of methods have been used to assess CH4 fluxes in vegetated areas, all with different strengths and drawbacks. The main differences between the approaches are the different CH4 flux pathways that they incorporate, and the spatial and temporal resolution of this coverage (Table 1). These approaches can be grouped into four main categories: (1) Those that quantify the plant-associated fluxes with whole plant chambers (Figure 2A), and those focusing on plant-mediated fluxes including submerged whole plant chambers (Figure 2B), leaf chambers (Figure 2C), inverted water filled bottles to capture stem flux (Figure 2D), stacked chambers to capture both submersed and emergent plant fluxes (Figure 2E), gas sampling from plant tissue (Figure 2F), experimental determination of diffusion potential of specific plant parts (Figure 2G), porewater sampling to determine potential root transport (Figure 2H) and incubation of plant material to measure potential CH4 production (Figure 2I); (2) approaches to determine ebullitive fluxes, which include inverted funnels (Figure 2J) and porewater sampling (Figure 2H); (3) approaches to quantify diffusive fluxes, which include floating chambers (Figure 2A) and measurements of gas concentration in the surface water combined with gas exchange (Figure 2K); (4) approaches to estimate whole habitat CH4 emissions, which include eddy covariance towers (Figure 2L). Note that some of these approaches overlap: Floating chambers, which are typically used in aquatic studies to measure diffusive fluxes and gas exchange, have also been used to determine ebullition and plant-mediated fluxes, whereas porewater sampling has been used for multiple purposes, including quantification of sediment CH4 production, diffusion from sediment, ebullition, and plant-mediated transport.
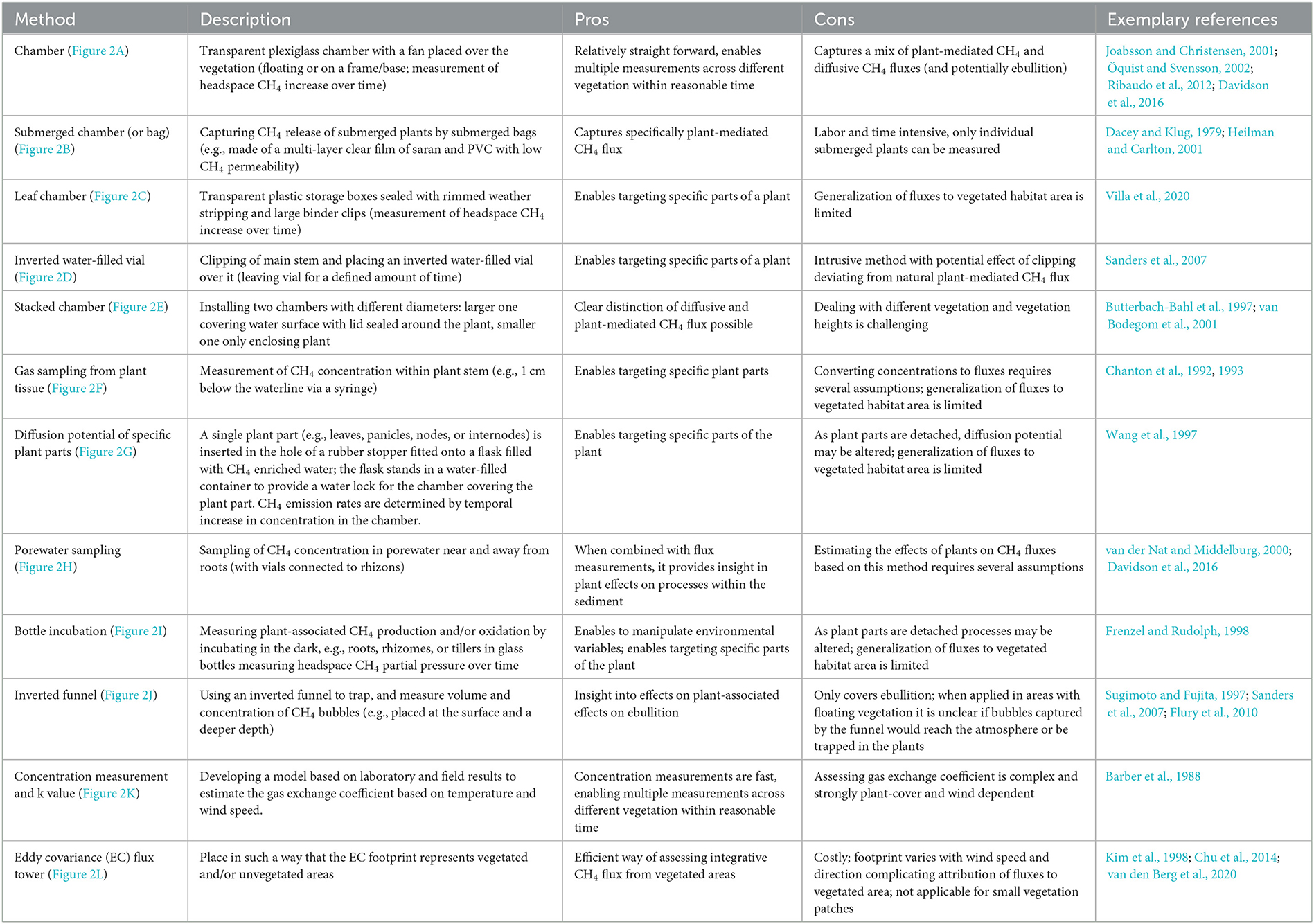
Table 1. Summary of methods used to determine CH4 fluxes of vegetated areas with some examples of method related pros and cons.
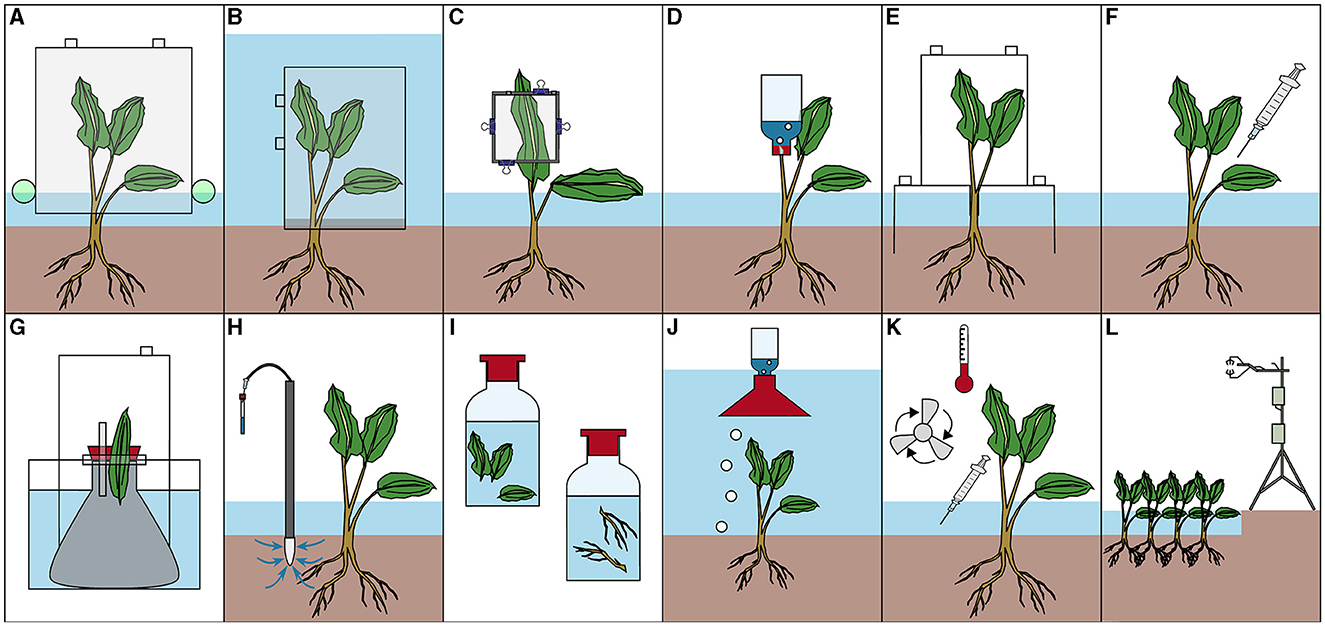
Figure 2. Methods to investigate CH4 fluxes of vegetated areas (field and laboratory): Chamber (A), Submerged chamber (B), Leaf chamber (C), Inverted water-filled vial (D), Stacked chamber (E), Gas sampling from plant tissue (F), Diffusion potential of specific plant parts (G), Porewater sampling (H), Bottle incubation (I), Inverted funnel (J), Concentration measurement and k value (K), Eddy covariance flux tower (L).
3 Analysis of extant data from vegetated areas in freshwaters
In this section we discuss existing plant-associated CH4 fluxes of a semi-quantitative literature search. Specifically, we discuss a compilation of the frequency of measurement approaches used and their specificities and limitations. In addition, we provide an overview of flux intensities associated with vegetated habitats among aquatic ecosystems, seasons, and plant types, and geographic regions.
3.1 Literature review and data compilation
To obtain an overview of studies on CH4 fluxes of vegetated areas in inland waters, we used two approaches. First, we selected four key papers (cited at least 200 times in Google Scholar; 4th of April 2023) dealing with the topic in distinct ways: Laanbroek (2010), reviewing microbial processes, Brix et al. (1996), focusing on Phragmites, Sebacher et al. (1985), investigating a large range of aquatic plants, and Ström et al. (2005), focusing on carbon turnover in multiple wetland plant species. We then exported the references citing these key papers. Secondly, a literature search was carried out using Web of Science on the 4th of April 2023. Search inquiry for Web of Science consisted of the following keywords: plant* or macrophyte* and aquatic or freshwater, and methane (asterisks where used to consider singular and plural), mediated, and methane. The combination of keywords resulted in the following formula: “TS = ((plant* OR macrophyte*) AND (aquatic OR freshwater) AND methane)” TS = (plant* AND mediated AND methane), TS meant that the search was done in the title, keywords, and the abstract of research papers. The search terms were used with no restriction on publication year. Lastly, we added papers from an earlier search with slightly different keywords (see Bodmer et al., 2021) and other relevant papers that we encountered while evaluating above-mentioned papers.
The data from the search inquiry, together with studies that had cited above mentioned key papers and the additional papers, were integrated into a database, and we retained CH4 flux data that were not associated to experimental manipulations (e.g., nutrient additions). We point out that the data collected in our search have been derived from papers that focused mostly on determining direct, plant-associated CH4 fluxes, usually of individual plant taxa, sometimes of mixed stands. There is a limited number of studies that have focused more broadly on various pathways of CH4 emission in vegetated habitats in inland waters, and there is often overlap between these two categories since the latter sometimes also determine direct plant-associated fluxes. Although we have not quantitatively reviewed other CH4 related processes in vegetated habitats (e.g., ebullition) because of the sparseness of available data, we do discuss the potential significance of these processes in the section below. Data were taken from the main text or from the Supplementary material of the retained papers. If the relevant data was not reported in the text, we used the WebPlotDigitizer tool (https://automeris.io/WebPlotDigitizer/) to read values from graphs. Some studies reported CH4 emissions e.g., under different circumstances or plant genera and hence have multiple entries, whereas other studies only reported averages of multiple observations and hence have only one entry in the database. Non-English and non-peer-reviewed papers were excluded, the rest of the papers were screened according to the title (if the title was not clear, the abstract was screened). If publications did not include original CH4 flux data (e.g., reviews) or did not include vegetation, we did not include them in the dataset. Additionally, we constrained this review by focusing on natural freshwater ecosystems or mesocosms mimicking natural systems. Hence papers on rice fields, constructed wetlands receiving wastewater, and water treatment facilities were not considered. Although we excluded papers dealing with CH4 fluxes from rice fields from our vegetated habitat flux database, we did review the methods used in rice field research and discuss their applicability in other freshwater systems.
Based on the data from relevant articles (188), we compiled a database with specific information (437 entries), including the genus of the dominated plant, range of CH4 fluxes, description of study site, and—when applicable—the type of experimental approach. To explore the geographical distribution of the studies, climate zones were assigned according to the latitude of reported coordinates: 60–90° northern; 60–40° temperate; 40–20° subtropical; 20–0° tropical (Delwiche et al., 2021). For experimental studies (e.g., based on mesocosms), we considered the location of the experiment, which does not necessarily represent the geographical location of the mimicked ecosystem.
3.2 Data analysis
After compiling the data obtained from the literature, we conducted the following steps: First, for 37% of the 437 entries, we calculated the average for each entry when this was not given (but when a range was published, for example). This was done by using the minimum and maximum value (geometric mean to consider mostly skewed data; applied in 31% of the cases), a time range presented (60%) or from replicates (9%). Second, all fluxes were converted to mg CH4 m−2 d−1. Third, we classified studies into three main groups: running waters (stream + river), lakes (ponds + lakes), and wetlands (bog + marsh + fen + mire + swamp).
In a first linear mixed-effect model (LME), we tested the fixed effects ecosystem type (running waters, lakes, wetlands), study period (growing, non-growing), plant type (emergent, submerged, floating), and climate zone (northern, temperate, subtropical; tropical) on CH4 fluxes of vegetated areas (response variable). In a second LME, we zoomed in on the emergent plants, for which we had most data. We tested the fixed effects plant genus (48 individual genera), ecosystem type (lakes, wetlands; there were no studies of emergent plants in running waters), study period (growing, non-growing season), and climate zone (arctic, boreal, temperate, tropic) on CH4 fluxes of vegetated areas (response variable). We used the “lmer” function of the R-package “lme4” (Bates et al., 2015) with Maximum Likelihood estimation. For the LMEs, we included study ID as a random effect on the intercept to account for the fact that one study can have multiple entries. Statistical significances of fixed effects were assessed with likelihood ratio tests using the function “drop1” (Zuur et al., 2009). The LMEs were followed by a model validation, checking the residuals for normal distribution and homogeneity of variances. For both LMEs, we transformed the response variable [log10(plant_flux) + min(plant_flux) + 1] to improve the model performance and validity. Both LMEs were followed by a pairwise comparison posthoc test (Tukey adjustment for multiple comparisons), comparing the individual levels of the significant fixed effects using the R-package “emmeans” (Lenth, 2019).
3.3 Geographical distribution of studies and methods used in the literature
We found that out of the screened literature (a total of 188 studies, 437 entries), the majority of studies used chamber measurements (162 studies; 382 entries), mostly with the objective of determining plant-associated fluxes. In a practical sense, this method seems straightforward, but it comes along with certain issues. Firstly, it is difficult to place the floating chamber on top of emerging and floating vegetation without disturbing the plants. Disturbance of vegetation may liberate gas bubbles therefore artificially inflating emissions; in addition, the chamber alters moisture, light, temperature, and carbon dioxide (CO2) concentrations which impacts stomata and may consequently influence CH4 fluxes. Secondly, often the chamber does not capture the plant's entire root system; when part of the root system exchanges gas with the overlying water outside the chamber area or other plant shoots with which they are connected (e.g., in case of Typha), these fluxes may be missed (e.g., Bansal et al., 2020). Thirdly, and more importantly, fluxes measured in floating chambers integrate direct plant-associated, diffusive water-atmosphere and potentially ebullitive pathways that are not always easy to differentiate, although some studies have attempted to disentangle these pathways, for example by modeling diffusive fluxes based on surface water concentrations (e.g., Desrosiers et al., 2022). The stacked chamber approach, used in rice studies (Butterbach-Bahl et al., 1997; van Bodegom et al., 2001) could be used to better differentiate between actual plant-associated CH4 fluxes, diffusion, and/or ebullition, avoiding the potentially misleading integration discussed above. The diffusion potential method (Wang et al., 1997) can be used to scan for possible within-plant hotspots of CH4 flux, but these approaches have been applied much less frequently.
The remainder of the studies used one or more of the other approaches listed in Table 2, sometimes singly, often in combination: porewater sampling (17 studies; 27 entries), eddy covariance flux tower (14 studies; 23 entries), inverted funnel (12 studies; 22 entries), concentration measurement and k value (9 studies; 18 entries), gas sampling from plant tissue (6 studies; 10 entries), bottle incubation (3 studies; 11 entries), submerged chamber (3 studies; 4 entries), leaf chamber (1 studies; 3 entries), and inverted water-filled bottles (1 study; 1 entry). Most of these approaches, but not all, target direct plant-associated fluxes, so they are not all strictly comparable, and there are very few studies that have reported the total CH4 emission from vegetated habitats (i.e., diffusive + ebullitive + plant-mediated).
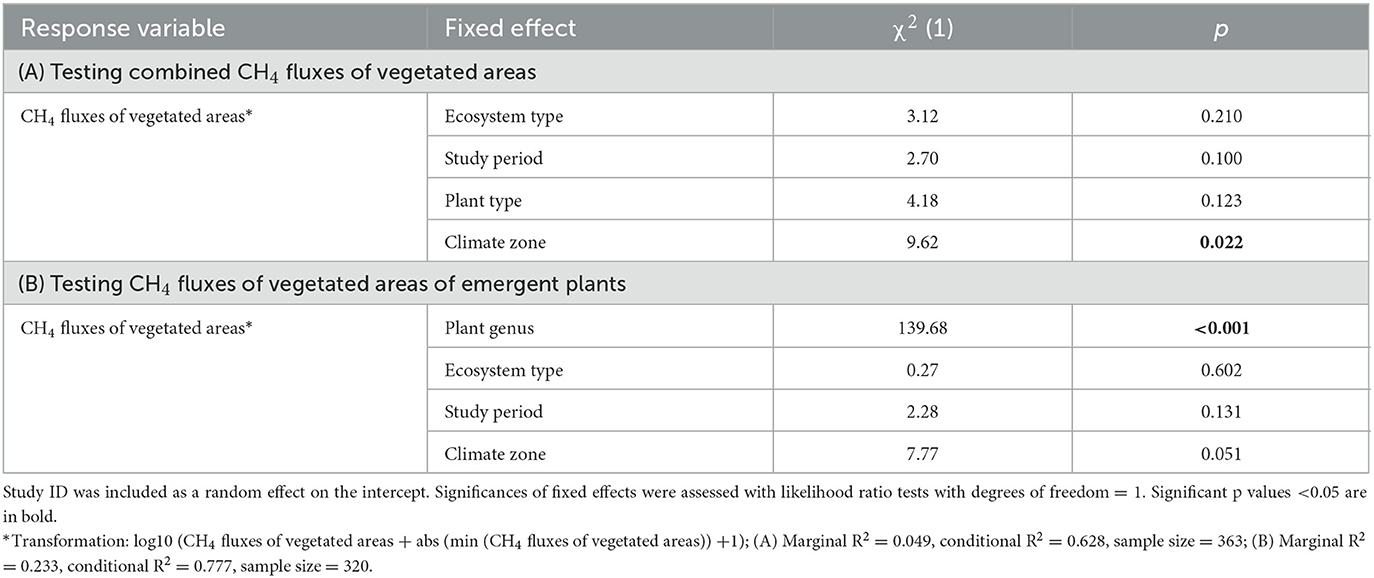
Table 2. Results of the linear mixed model testing effects of ecosystem type (running water, lake, wetland), study period (growing, non-growing), plant type (emergent, floating, submerged), climate zone (arctic, boreal, temperate, tropic) on CH4 fluxes of vegetated areas (A), and testing the effects of plant genus (48 individual genera), ecosystem type (lake, wetland), study period (growing, non-growing), and climate zone (arctic, boreal, temperate, tropic), on CH4 fluxes of vegetated areas of emergent plants (B).
Of the reviewed studies (188 studies, 437 entries; 7 studies contained a field and mesocosm component), most were performed in the field (157 studies; 333 entries) compared to mesocosm experiments (38 studies; 104 entries). Studies on all scales are valuable to both obtain mechanistic understanding and enable upscaling efforts. Furthermore, mesocosm experiments can be used to simulate running waters/flow conditions, which would help to constrain and to understand CH4 fluxes of vegetated areas in running waters.
From the 188 compiled studies (of which seven studies were performed on two continents), 79 were performed in North America (162 entries), followed by 67 in Europe (171 entries), and 36 (84 entries), 7 (10 entries), 5 (9 entries), and 1 (1 entry) in Asia, South America, Oceania, and Africa, respectively (Figure 3). For some regions we found but one study—e.g., Africa, and in other regions there are only a handful of studies conducted, e.g., in South America (e.g., Silva et al., 2016; Oliveira-Junior et al., 2020), yet this is where some of the most extensive inland aquatic systems are located (e.g., Amazon and Pantanal). In addition, there is a strong bias toward growing season-only data and focus on a small subset of genera and plant types. These biases all contribute to the large uncertainty of freshwater systems in global CH4 budgets (Saunois et al., 2020). Consequently, our current understanding of the magnitude of CH4 fluxes of vegetated areas is strongly biased.
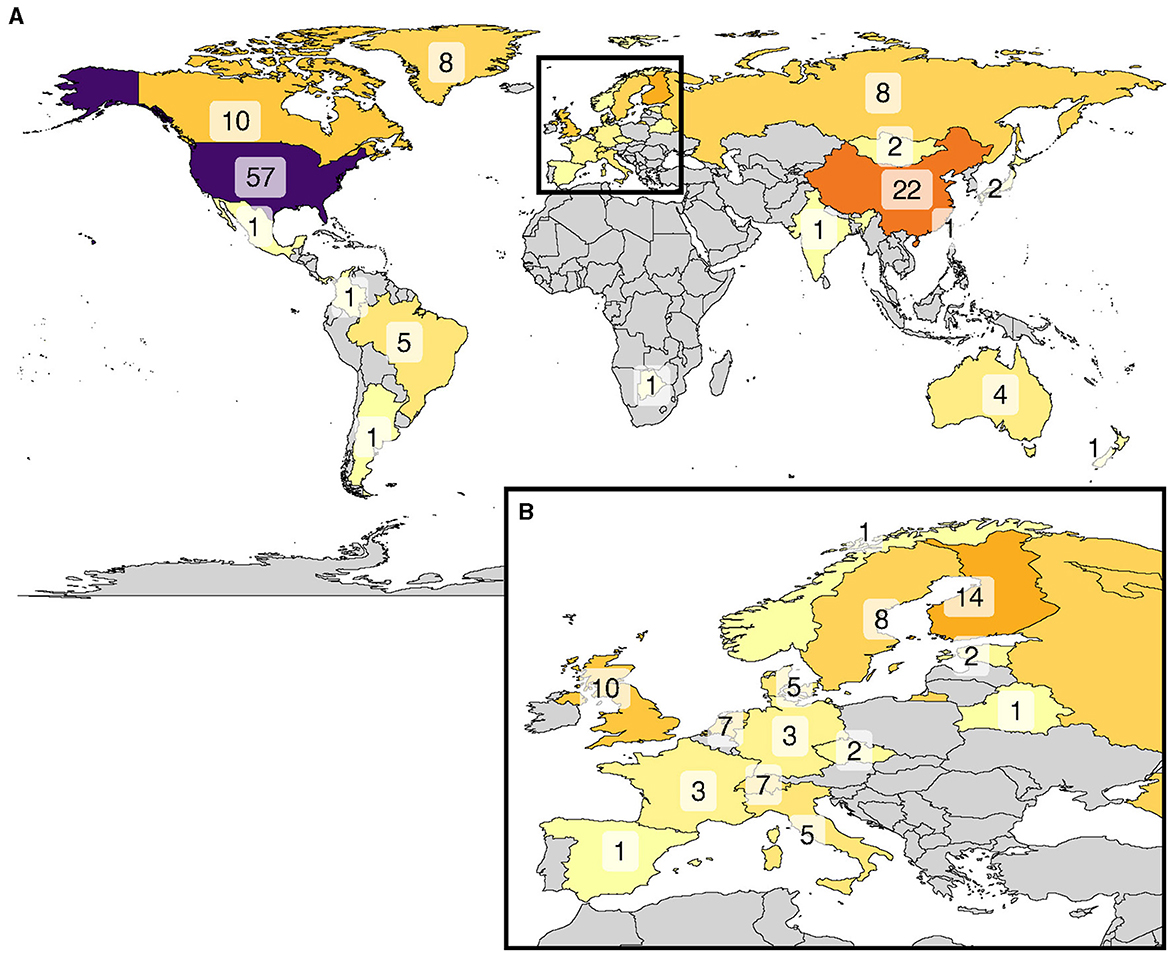
Figure 3. Geographical distribution of studies related to CH4 fluxes of vegetated areas (A) with a zoom-in of Europe (B). The number of studies is indicated on the respective countries accompanied by different color intensities indicating the higher frequency (higher color intensity) and lower frequency (lower color intensity) of performed studies. Grey areas indicate no available studies according to our literature search parameters.
3.4 CH4 flux intensity and variability from vegetated areas
Although our systematic literature review points to major spatial and temporal data gaps, and differences in methods that complicate data comparisons, we nevertheless attempt here to provide an overview of the extant CH4 flux estimates for freshwater vegetated habitats. We should reiterate that most of the data that we were able to collect were derived from chamber-based measurements involving emergent plants, which likely include both water-atmosphere diffusive fluxes that are more or less impacted by the plants and direct plant-mediated fluxes. These literature fluxes do mostly not fully capture ebullitive fluxes, and therefore likely significantly underestimate total CH4 emissions from these vegetated habitats, as we have discussed in previous sections.
From an ecosystem perspective, of total 188 studies (437 entries; seven studies were performed in two different ecosystems), 156 were carried out in wetlands (357 entries), whereas only 37 (78 entries) in lakes and 2 (2 entries) in running waters. This implies that the available data are strongly imbalanced in terms of the habitats covered, and there is a clear need for more studies in standing and running waters.
CH4 fluxes of vegetated areas ranged from−8.6 (uptake) to 2835.8 (emission) mg CH4 m−2 d−1 {65.0 (9.3, 194.4); median [interquartile range (IQR)]} in wetlands, from 0.2 to 971.8 mg CH4 m−2 d−1 [86.1 (28.0, 235.0)] in lakes, and from 5.4 to 39.0 mg CH4 m−2 d−1 in running waters [22.2 (13.8, 30.6)] (Figure 4). There was no significant effect of ecosystem type on CH4 fluxes of vegetated areas, whereas climate zone was a significant driver (p = 0.022; Table 2A).
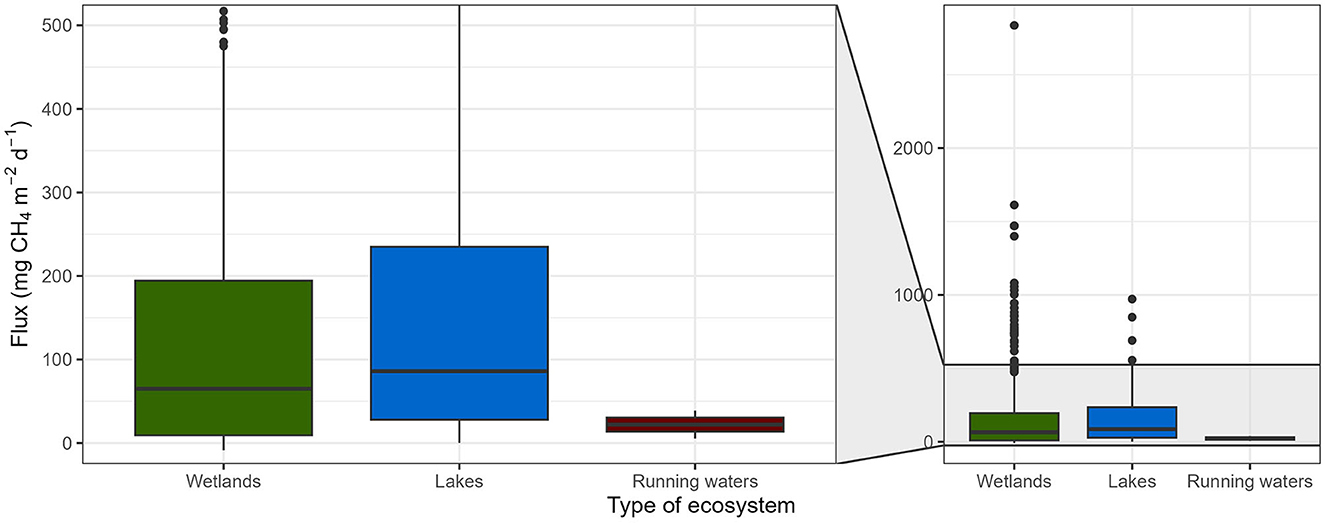
Figure 4. CH4 fluxes of vegetated areas of different ecosystem types (wetlands, lakes, and running waters). Boxplots represent median (black line), first and third quartiles (hinges), range (whiskers), and outliers (black dot).
Of all the 188 studies (437 entries), only 11 studies (16 entries) are from the non-growing season, compared to 146 studies (347 entries) performed in the growing season, while 36 studies (69 entries) include measurements throughout the year. The very similar median fluxes (Figure 5) in the growing and non-growing season calls for more attention to CH4 fluxes of vegetated areas outside the growing season. For example, the genus Phragmites does not grow in winter but may facilitate CH4 emissions by creating a direct pathway or “chimney“ from the sediments to the atmosphere while the water column is sealed off from the atmosphere by ice. Fluxes outside the growing period may be considerable and need further attention.
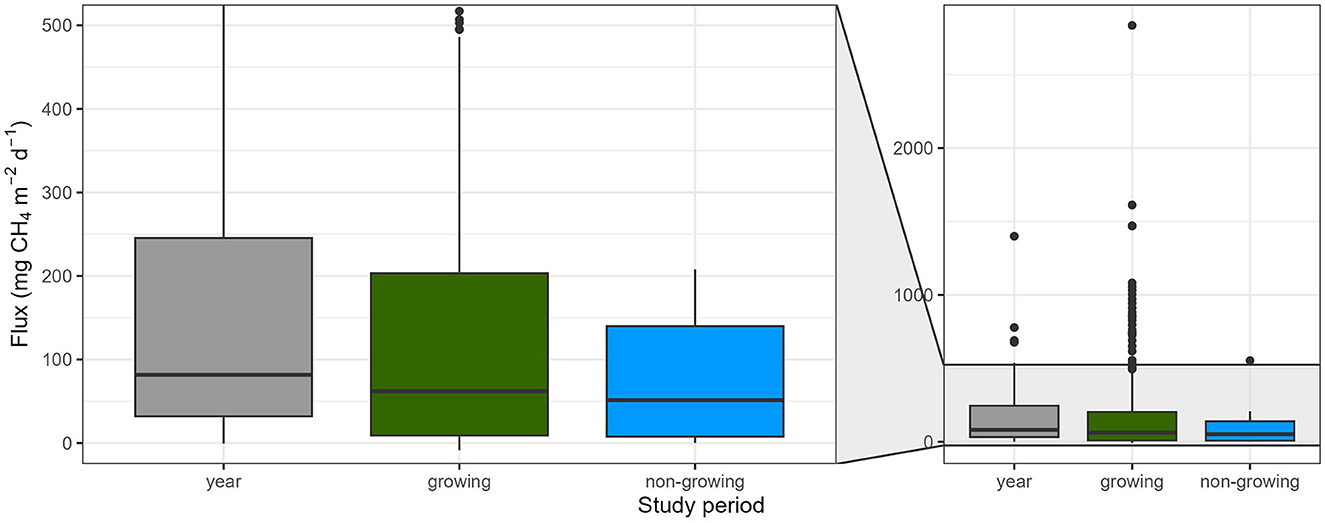
Figure 5. CH4 fluxes of vegetated areas from different plant types combined, measured in 3 different seasons (growing and non-growing season, and year-round studies). Boxplots represent median (black line), first and third quartiles (hinges), range (whiskers), and outliers (black dot).
Study period did not significantly influence CH4 fluxes of vegetated areas (p = 0.100; Table 2A) with fluxes ranging from−8.6 to 2835.8 mg CH4 m−2 d−1 (62.0 [9.0, 203.3]) in the growing season, and from 0.1 to 553.2 mg CH4 m−2 d−1 (51.4 [7.7, 140.0]) in the non-growing season (Figure 5). Also, when we zoom in on emergent macrophytes alone, we find no significant effect of study period (p = 0.131; Table 2B). One explanation for the absence of a significant effect might be that organic matter supply rate (Grasset et al., 2021), e.g., due to dying vegetation, could actually be more relevant in driving CH4 production and emissions than the actual presence of growing plants. Alternatively, there may be geographic biases location of annual studies that minimize potential seasonal differences. Regardless, these annual patterns certainly deserve more attention.
There was a strong imbalance in the focus on different plant growth forms/types, with the majority of studies focusing on emergent plants, with only a few studies focusing on floating plants and barely any on submerged vegetation (Figure 6).
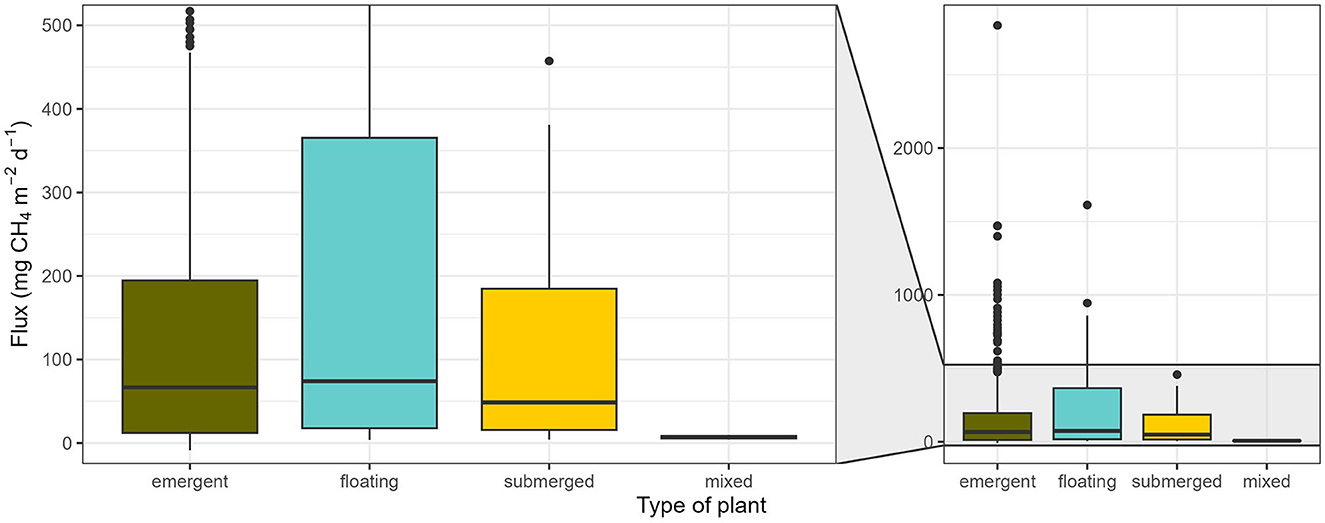
Figure 6. CH4 fluxes of vegetated areas from different plant types. Emergent: 171 studies, 384 entries; floating: 22 studies, 33 entries; submerged: 8 studies, 18 entries; mixed: 1 study, 2 entries. Boxplots represent median (black line), first and third quartiles (hinges), range (whiskers), and outliers (black dot).
The fluxes for habitats with emergent plants ranged from−8.6 to 2835.8 mg CH4 m−2 d−1 (66.7 [12.2, 194.6]), for floating plants from 3.9 to 1612.3 mg CH4 m−2 d−1 [74.0 (17.7, 365.4)], and for submerged plants from 4.2 to 457.3 mg CH4 m−2 d−1 [48.6 (15.7, 184.7)]. Fluxes from mixed stands ranged from 4.2 to 9.9 mg CH4 m−2 d−1 [7.1 (5.6, 8.5)] (Figure 6). These differences however did not lead to a significant effect of plant type on CH4 fluxes of vegetated areas (p = 0.123; Table 2A). This result underlines that none of the plant types is of lesser importance in terms of CH4 emissions. Different processes may underlie the high emissions from areas with floating plants, particularly the low oxygen conditions below the floating plants resulting from the inhibition of water column photosynthesis and the decreased oxygen exchange imposed by floating plants (see also Section 2). The low oxygen availability in the water column and sediment creates favorable circumstances for methanogenesis and may impede CH4 oxidation and thus large CH4 build up. When floating plants are rooted in the sediment, connecting sediments and bottom waters with high CH4 concentrations directly to the atmosphere through plant-associated transport, emissions may be particularly high (Oliveira-Junior et al., 2018, 2020). However, only a small fraction of studies indicated whether the studied floating plants were free-floating or rooted in the sediment. Especially since some species can be both rooted or not rooted (see e.g., Oliveira-Junior et al., 2020), this information should be definitely better considered and reported in future studies.
Within the emergent plants (384 entries), most data are from mixed plant stands (32.3%), followed by Carex (16.1%), Eriophorum (8.9%), Phragmites (8.6%), and Typha stands (5.2%). Not surprisingly, as seen in the distribution of the studies (Figure 3), emphasis has been on plants widely occurring in the subarctic, boreal and temperate regions. The remaining 43 genera/combination of genera represent < 4.0% of the entries (Supplementary Table S1). Within floating plants (33 entries), 32.3% of the data are from the genus Nuphar, followed by Eichhornia (21.2%), mixed plant stands (12.1%), and Nelumbo, Nymphaea, and Trapa with 9.1% each (Supplementary Table S1). Finally, most data for submerged plants (18 entries) are from Myriophyllum (33.3%), and Ceratophyllum, Ranunculus, and Vallisneria with 11.1% each (Supplementary Table S1).
CH4 fluxes from different genera within the different plant types are highly variable (Figure 7 and Supplementary Figure S1). Genus was the strongest predictor of fluxes of emergent plants in the LME run of emergent plants (p < 0.001; Table 2B). Hence, there are differences among genera, e.g., Panicum had significantly higher emissions than Betula, Mimulus, Cassiope, Salix, and Sphagnum, respectively (pairwise comparison posthoc p < 0.05). As mentioned above, there is a strong imbalance related to the number of entries of individual genera. A higher number of entries for a specific genus (e.g., for Phragmites) likely leads to higher variability since it has been studied in very different systems, which creates a certain bias and an imbalanced model. We are therefore currently unable to predict CH4 fluxes based on occurring genera on a large scale. Given the large variability in CH4 fluxes within genera it is also questionable if reasonable genera inferred predictions of CH4 fluxes can be developed. Predictions made based on vegetation types—aggregating several genera at a specific site, thereby “controlling” for climate and soil type—are likely more promising (see e.g., Juutinen et al., 2003; Robroek et al., 2015).
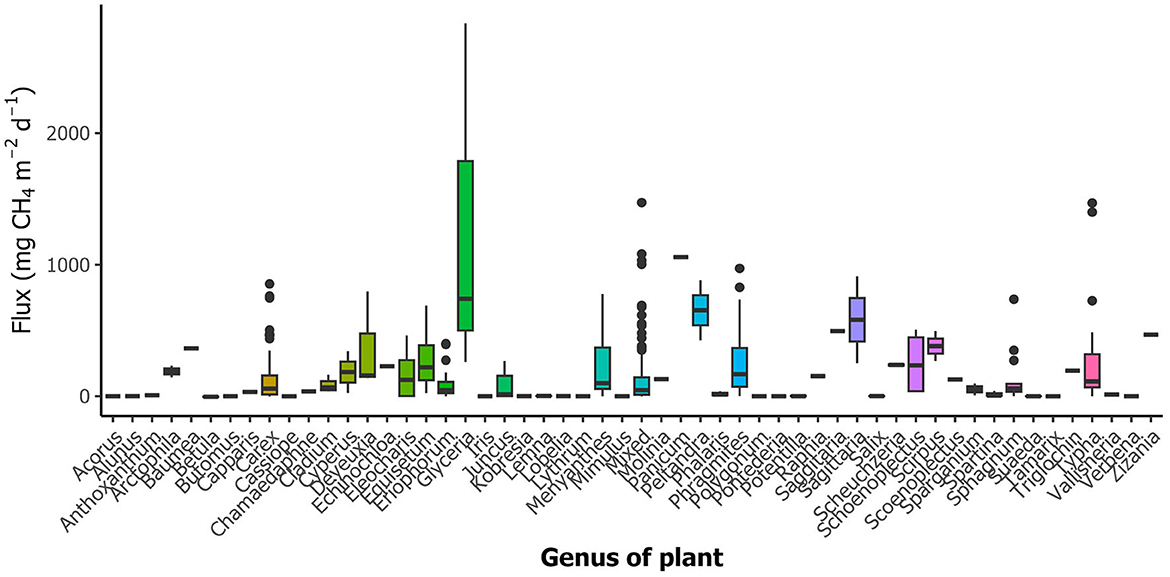
Figure 7. CH4 fluxes of vegetated areas from individual genera of investigated emergent plants. Boxplots represent median (black line), first and third quartiles (hinges), range (whiskers), and outliers (black dot).
4 Plant effects on CH4 fluxes: implications for current global CH4 estimates from freshwaters
The extent to which CH4 emissions from vegetated habitats have been incorporated into current local, regional, and global freshwater CH4 budgets varies greatly and is not always specified. In the following sections we discuss some of the challenges and implications of incorporating fluxes from vegetated habitats into current global estimates of CH4 emissions from inland waters.
4.1 Incorporating vegetated habitats into lake CH4 budgets
Recent global lake CH4 emission estimates range from 24.0 ± 8.4 Tg CH4 yr−1 (Zhuang et al., 2023), over 41.6 ± 18.3 Tg CH4 yr−1 (Johnson et al., 2022), up to 150.9 ± 73.0 Tg CH4 yr−1 (Rosentreter et al., 2021). These global estimates differ in various aspects, such as lake size cut-off, global lake cartographies, upscaling approach, and consideration of seasonality and ice cover. However, these estimates have one thing in common: none of them considers plant-associated CH4 emissions from vegetated areas. In a recent overview study on the global regulation of CH4 emissions from lakes, the emission via vegetation is even explicitly excluded, and only open water fluxes are considered (Sanches et al., 2019). The median of plant-associated CH4 emissions in lakes (Figure 4) is 86 mg CH4 m−2 d−1, i.e., 1.8 to 1.3 times higher than the median open-water lake emission estimated by Rosentreter et al. (2021; i.e., 46 mg CH4 m−2 d−1 extracted from Figure 1) or the estimated diffusive summer emissions by Johnson et al. (2022; 68 mg CH4 m−2 d−1; July data extracted from Supplementary Figure S2), respectively. Vegetated areas tend to make up a considerable portion of total area of many lakes—for example the vegetated habitat (either floating, emergent, or submerged, or different combinations of these) had a median coverage of 33% in 109 lakes around the world (Zhang Y. et al., 2017). Given the consistently high emissions that characterize those areas, incorporating CH4 fluxes from vegetated habitats will likely result in significant increases in current estimates of global CH4 emissions.
The magnitude of plant-associated CH4 fluxes likely varies regionally, depending on the proportion of vegetated habitat coverage. For example, Kyzivat et al. (2022) recently showed that neglecting vegetated areas leads to an underestimation of 21% of the CH4 emission for lakes across four Arctic-boreal study areas. As pointed out in the sections above, it is important to emphasize that the median flux of 86 mg CH4 m−2 d−1 for vegetated areas that we report here does not reflect the total CH4 emissions of these habitats (e.g., the ebullitive flux is often missing, see Section 2.5), although it is still unclear what proportion of these emissions are accounted for by the existing data. Hence, we argue that our estimate of the importance of vegetated areas is very conservative.
4.2 Incorporating vegetated habitats into river CH4 budgets
The current global annual CH4 emission for rivers is estimated to be in the order of 27.9 (16.7–39.7) Tg CH4 yr−1 (Rocher-Ros et al., 2023). These fluxes exclude emissions from emergent vegetation, and it is unclear to what extent they include submerged vegetation. Our literature search, unfortunately, yielded only two studies (Sanders et al., 2007; Kuhn et al., 2017) where both CH4 emissions from vegetated river sections and unvegetated sections were explicitly included. It is therefore difficult to speculate on the relevance of vegetation for riverine CH4 fluxes, but many rivers worldwide develop extensive vegetated habitats, particularly in lowland tropical and subtropical regions, and there is no question that global river CH4 emissions will have to be eventually adjusted for fluxes from these habitats as new information is acquired on the extent and composition of these riverine habitats. One of the difficulties ahead is that the distinction between vegetated river habitats and wetlands is not always clear, so avoiding double accounting while effectively capturing these riverine features remains a major challenge.
4.3 Wetlands
Current estimates of global wetland CH4 emissions are in the order of 149 Tg CH4 yr−1 (Rosentreter et al., 2021), and global models of wetland-methane feedbacks indicate that wetland CH4 emissions could play a significant role in driving 21st-century climate forcing, with global wetland emissions potentially matching or exceeding anthropogenic emissions by 2100 (Zhang B. et al., 2017). It is therefore of key importance to improve our understanding of the magnitude, regulation and sources of uncertainty of wetland CH4 emissions, which necessarily implies a better accounting for the role of vegetation. Contrary to the situation with lakes and rivers, where vegetated habitats have been systematically excluded from estimates of whole ecosystem CH4 emission, and therefore from global inland CH4 budgets, wetland CH4 budgets have variously incorporated emissions from plants and from vegetated habitats. Regional (Ito, 2021) and global (Ito and Inatomi, 2012) wetland CH4 estimates have been derived using process-based models. For example, the VISIT (the Vegetation Integrative Simulator for Trace gases) and other wetland process models (Cao et al., 1996; Walter and Heimann, 2000; Hodson et al., 2011; Arora et al., 2018; Parker et al., 2018) have incorporated wetland CH4 modules that integrate CH4 diffusive, ebullitive, and plant-mediated CH4 fluxes. The latter have been modeled on the basis of vegetation coverage and composition (e.g., Bubier et al., 1995; Dias et al., 2010; Robroek et al., 2017), and primary production of vegetation as it fuels carbon stocks that are subsequently available for decomposition. Only a handful of models have explicitly incorporated the transport through plants in wetlands (e.g., Riley et al., 2011; Ito and Inatomi, 2012; Kleinen et al., 2020). Potentially influential factors such as the impact of vegetation community composition on transport through plants, as well as plant effects on CH4 oxidation, are generally not included in larger-scale models (but see Knoblauch et al., 2015 and Zhang B. et al., 2017, the latter included CH4 oxidation during plant-associated transport in their global wetland model). Modeled wetland CH4 emissions vary greatly and not always coherently (Melton et al., 2013; Ciais et al., 2014), and it is clear that there is still much to be learnt concerning the role of vegetation in CH4 production, oxidation, and transport (Berrittella and van Huissteden, 2011; Riley et al., 2011). These uncertainties may partly underlie the large discrepancies that still exist between inverse modeling and bottom-up estimates of the global and regional CH4 budgets, which have been at least in part attributed to problems with estimates of wetland emissions (Bohn et al., 2015; Saunois et al., 2017, 2020). These uncertainties are related to the extent of global wetland distribution, as well as to our lack of understanding of the key underlying CH4 processes, notably the contribution of plants themselves (Bloom et al., 2017; Peltola et al., 2019). In wetlands in particular, herbaceous plants (Carmichael et al., 2014) and trees (Covey and Megonigal, 2019) are emerging as major contributors to wetland CH4 emissions, yet their role has yet to be fully quantified and incorporated in wetland models. The literature that we collected and the statistical models we developed of plant-associated fluxes do not allow us to further speculate on a potential impact of plant associated fluxes on wetland CH4 emissions, because on the one hand, we do not have herbaceous and tree flux data, and on the other, it is at present, very difficult to assess to what extent the aquatic plant fluxes are explicitly incorporated in current global wetland emission estimates, and if they are, how accurate they are.
5 Conclusions and outlook
CH4 fluxes from vegetated areas in inland waters, especially in lakes and rivers, are largely understudied and neither well understood nor quantified. Our review represents, to our knowledge, the first attempt to synthesize the many ways in which submerged, floating, and emergent vegetation may influence CH4 fluxes in different freshwater ecosystems, combined with a compilation of methodological approaches used to assess vegetation effects on CH4-related processes. Moreover, we provide an overview of published CH4 fluxes from vegetated habitats from various types of ecosystems across a geographical, seasonal, and vegetation types, and assess the potential significance of CH4 fluxes from vegetated areas for global lake CH4 emissions.
From a methodological perspective we urge for a more standardized approach of measuring CH4 fluxes from vegetated areas. We emphasize the importance of considering not only the growing season in order to obtain more robust annual estimates, since the influence of plants extends well beyond their growing period. To specifically capture direct plant-associated CH4 fluxes we suggest the stacked chamber method (Table 1) as the most appropriate one, since it allows to separate the direct plant-mediated emission from the diffusive flux. Further, we suggest assessing if plants are rooted or non-rooted when measuring fluxes from floating plants, since this information is relevant for future upscaling or generalization efforts. More importantly perhaps, our review highlights the need for studies that capture total CH4 emissions from the vegetated habitats, by simultaneously measuring diffusion, ebullition, and direct plant-associated—also referred to as plant-mediated—fluxes. The few studies that have done so suggest that the magnitude of these pathways and their contribution to overall CH4 emission can vary greatly between vegetated habitats, and therefore focusing on any given pathway yields an incomplete and biased perspective of CH4 dynamics in these systems.
The presence of emergent and submerged vegetation influences CH4 dynamics in multiple ways. Although it is challenging, disentangling the effects of plants on CH4 production, oxidation, and transport is important to understand and model CH4 emissions from vegetated areas, and particularly to predict how plants will affect future CH4 emissions. Aerenchyma development and root organic carbon release, for instance, will respond differently to increasing atmospheric carbon dioxide levels, warmer temperatures, and altered precipitation and soil moisture patterns (Armstrong, 1980; Gregory et al., 1995; Cheng, 1999; Visser et al., 2000; Leakey, 2009) thereby influencing the plant effects on CH4 emission in potentially synergistic but possibly also antagonistic ways. Future studies should therefore focus on vegetation-related aspects of CH4 production, oxidation, and/or transport in the light of global and land-use change and link these processes to quantifiable plant traits as a way to facilitate modeling of CH4 dynamics in vegetated habitats.
Although beyond the scope of this study, we recommend incorporating CO2 and N2O fluxes besides CH4 pathways to fully capture the role of vegetated areas in the carbon and greenhouse gas balance of inland waters (see e.g., Aben et al., 2022). It is important to consider that these vegetated habitats, while generally being hotspots of CH4 emission, may also take up and store significant amounts of carbon, and also have variable roles in terms of N2O dynamics; their radiative balance can therefore only be determined if a complete GHG is carried out. For example, it has been shown that CH4 emissions partially offset “blue carbon” burial in mangroves (Rosentreter et al., 2018), yet similar assessments in freshwater systems are scarce (but see Bastviken et al., 2011; van Bergen et al., 2019).
At present, upscaling CH4 fluxes from vegetated areas to the ecosystem, landscape, and global scale is challenging [but see Kyzivat et al. (2022) for an example of how vegetated areas can be included on a regional scale]. We have shown here that although plant-associated fluxes are extremely variable, depending on plant type and habitat, they tend to be consistently high relative to pelagic non-vegetated areas (Desrosiers et al., 2022; Kyzivat et al., 2022), such that vegetated habitats in inland waters tend to contribute disproportionately to whole ecosystem CH4 emissions on an areal basis. In this regard, our preliminary assessment suggests that inclusion of vegetated habitats would likely increase current estimates of global lake CH4 emissions. We cannot at present derive a similar assessment for rivers, but in all likelihood, it will not be negligible either because many lowland rivers in the world develop extensive vegetated areas, so efforts should be made to collect the data necessary to carry out this exercise in the near future.
To narrow down estimates of the global contribution of this source depends on (i) more standardized methods with clear accounting for and disentangling of different CH4 flux pathways or making sure that the vegetated habitat is captured as a whole, (ii) flux measurements taken across the complete geographic extent and vegetation type (i.e., emergent, floating, and submerged), and (iii) a better and more complete assessment of vegetation cover, for example using remote sensing techniques (e.g., Silva et al., 2008; Melack and Hess, 2023). General trends in plant coverage in inland waters as a function of environmental and climate change are difficult to predict (Zhang Y. et al., 2017), and differ among types of vegetation (i.e., emergent, floating, submerged) and regions. Specifically, global change seems to favor floating over submerged vegetation (Netten et al., 2011; Peeters et al., 2013) and may lead to an increase in invasive vegetation (Shih and Finkelstein, 2008; Emilson et al., 2018) which may profoundly impact CH4 budgets of aquatic systems (Tong et al., 2012; Beyene et al., 2022). Hence, the understudied and likely globally underestimated CH4 emissions from vegetated areas might become more relevant due to global warming, land-use change, and shifts in species distributions. CH4 emissions from vegetated habitats are largely unaccounted for in current global estimates of lake and river CH4 emission, and it is clear that these emissions merit more attention.
Data availability statement
The dataset compiled for this study can be found on figshare [dx.doi.org/10.6084/m9.figshare.24847161].
Author contributions
PB: Conceptualization, Data curation, Formal analysis, Methodology, Project administration, Validation, Visualization, Writing—original draft, Writing—review & editing. RV: Data curation, Validation, Visualization, Writing—review & editing, Writing—original draft. TS: Conceptualization, Data curation, Validation, Writing—review & editing. PdG: Conceptualization, Resources, Supervision, Writing—review & editing. SK: Conceptualization, Methodology, Resources, Supervision, Writing—review & editing.
Funding
The author(s) declare financial support was received for the research, authorship, and/or publication of this article. PB was partially financed by an individual research grant from the German Research Foundation (BO 5050/1-1). SK was partially financed by NWO-TTW Project No. 18661 and NWO-VIDI 203.098. This work is part of the CarBBAS (Carbon Biogeochemistry in Boreal Aquatic Systems) Research program, co-funded by the Natural Sciences and Engineering Research Council of Canada and Hydro-Québec (NSERC Grant CRDPJ 543873 – 19).
Acknowledgments
We thank Vincent Fugère for his help in setting up the linear mixed models and Joan Pere Casas-Ruiz for his helpful input. We also thank Karelle Desrosiers for fruitful discussions and input into the wetland section. We thank the reviewers for their constructive feedback which improved the clarity of the manuscript.
Conflict of interest
The authors declare that the research was conducted in the absence of any commercial or financial relationships that could be construed as a potential conflict of interest.
Publisher's note
All claims expressed in this article are solely those of the authors and do not necessarily represent those of their affiliated organizations, or those of the publisher, the editors and the reviewers. Any product that may be evaluated in this article, or claim that may be made by its manufacturer, is not guaranteed or endorsed by the publisher.
Supplementary material
The Supplementary Material for this article can be found online at: https://www.frontiersin.org/articles/10.3389/frwa.2023.1332968/full#supplementary-material
References
Aben, R. C. H., Oliveira Junior, E. S., Carlos, A. R., van Bergen, T. J. H. M., Lamers, L. P. M., Kosten, S., et al. (2022). Impact of plant species and intense nutrient loading on CH4 and N2O fluxes from small inland waters: an experimental approach. Aquat. Bot. 180, 103527. doi: 10.1016/j.aquabot.2022.103527
Armstrong, W. (1980). “Aeration in higher plants,” in Advances in Botanical Research, (Amsterdam: Elsevier), 225–332.
Arora, V. K., Melton, J. R., and Plummer, D. (2018). An assessment of natural methane fluxes simulated by the CLASS-CTEM model. Biogeosciences 15, 4683–4709. doi: 10.5194/bg-15-4683-2018
Attermeyer, K., Flury, S., Jayakumar, R., Fiener, P., Steger, K., Arya, V., et al. (2016). Invasive floating macrophytes reduce greenhouse gas emissions from a small tropical lake. Sci. Rep. 6, 1–10. doi: 10.1038/srep20424
Ávila, M. P., Oliveira-Junior, E. S., Reis, M. P., Hester, E. R., Diamantino, C., Veraart, A., et al. (2019). The water hyacinth microbiome: link between carbon turnover and nutrient cycling. Microb. Ecol. 78, 575–588. doi: 10.1007/s00248-019-01331-9
Bansal, S., Johnson, O. F., Meier, J., and Zhu, X. (2020). Vegetation affects timing and location of wetland methane emissions. J. Geophy. Res. Biogeosci. 125, 1–39. doi: 10.1029/2020JG005777
Barber, T. R., Burke, R. A., and Sackett, W. M. (1988). Diffusive flux of methane from warm wetlands. Glob. Biogeochem. Cycles 2, 411–425. doi: 10.1029/GB002i004p00411
Bastviken, D. (2009). “Methane” in Encyclopedia of Inland Waters, Ed. G. E. Likens (Cambridge, MA: Academic Press).
Bastviken, D., Tranvik, L. J., Downing, J. A., Crill, P. M., and Enrich-Prast, A. (2011). Freshwater methane emissions offset the continental carbon sink. Science 331, 50. doi: 10.1126/science.1196808
Bastviken, D., Treat, C. C., Pangala, S. R., Gauci, V., Enrich-Prast, A., Karlson, M., et al. (2023). The importance of plants for methane emission at the ecosystem scale. Aquat. Bot. 184, 103596. doi: 10.1016/j.aquabot.2022.103596
Bates, D., Mächler, M., Bolker, B., and Walker, S. (2015). Fitting linear mixed-effects models using lme4. J. Stat. Softw. 67, 1–48. doi: 10.18637/jss.v067.i01
Berrittella, C., and van Huissteden, J. (2011). Uncertainties in modelling CH4 emissions from northern wetlands in glacial climates: the role of vegetation parameters. Clim. Past 7, 1075–1087. doi: 10.5194/cp-7-1075-2011
Beyene, B. B., Li, J., Yuan, J., Dong, Y., Liu, D., Chen, Z., et al. (2022). Non-native plant invasion can accelerate global climate change by increasing wetland methane and terrestrial nitrous oxide emissions. Glob. Chan. Biol. 28, 5453–5468. doi: 10.1111/gcb.16290
Bloom, A. A., Bowman, M., Lee, A. J., Turner, R., Schroeder, J. R., Worden, R., et al. (2017). CMS: Global 0.5-deg wetland methane emissions and uncertainty (WetCHARTs v1. 0). Oak Ridge, TN: ORNL DAAC.
Bodmer, P., Vroom, R., Stepina, T., del Giorgio, P. A., and Kosten, S. (2021). Methane fluxes of vegetated areas in natural freshwater ecosystems: assessments and global significance. EarthArXiv. doi: 10.31223/X5ND0F
Bodmer, P., Wilkinson, J., and Lorke, A. (2020). Sediment properties drive spatial variability of potential methane production and oxidation in small streams. J. Geophy. Res. Biogeosci. 2016, 1–15. doi: 10.1029/2019JG005213
Bogard, M. J., Giorgio, P. A., d Boutet, L., Chaves, M. C. G., Prairie, Y. T., Merante, A., et al. (2014). Oxic water column methanogenesis as a major component of aquatic CH 4 fluxes. Nat. Commun. 5, 1–9. doi: 10.1038/ncomms6350
Bohn, T. J., Melton, J. R., Ito, A., Kleinen, T., Spahni, R., Stocker, B. D., et al. (2015). WETCHIMP-WSL: intercomparison of wetland methane emissions models over West Siberia. Biogeosciences 12, 3321–3349. doi: 10.5194/bg-12-3321-2015
Brix, H. (1993). “Macrophyte-mediated oxygen transfer in wetlands: transport mechanisms and rates,” in Constructed Wetlands for Water Quality Improvement, (Boca Raton, FL: Lewis Publishers), 391–398.
Brix, H., Sorrell, B. K., and Schierup, H.-H. (1996). Gas fluxes achieved by in situ convective flow in Phragmites australis. Aquat. Bot. 54, 151–164. doi: 10.1016/0304-3770(96)01042-X
Bruhn, D., Møller, I. M., Mikkelsen, T. N., and Ambus, P. (2012). Terrestrial plant methane production and emission. Physiol. Plant. 144, 201–209. doi: 10.1111/j.1399-3054.2011.01551.x
Bubier, J. L., Moore, T. R., Bellisario, L., Comer, N. T., and Crill, P. M. (1995). Ecological controls on methane emissions from a northern peatland complex in the zone of discontinuous permafrost, Manitoba, Canada. Glob. Biogeochem. Cycl. 9, 455–470. doi: 10.1029/95GB02379
Butterbach-Bahl, K., Papen, H., and Rennenberg, H. (1997). Impact of gas transport through rice cultivars on methane emission from rice paddy fields. Plant Cell Environ. 20, 1175–1183. doi: 10.1046/j.1365-3040.1997.d01-142.x
Cao, M., Marshall, S., and Gregson, K. (1996). Global carbon exchange and methane emissions from natural wetlands: Application of a process-based model. J. Geophy. Res. Atmosph. 101, 14399–14414. doi: 10.1029/96JD00219
Carmichael, M. J., Bernhardt, E. S., Bräuer, S. L., and Smith, W. K. (2014). The role of vegetation in methane flux to the atmosphere: should vegetation be included as a distinct category in the global methane budget? Biogeochemistry 119, 1–24. doi: 10.1007/s10533-014-9974-1
Chanton, J. P., and Dacey, J. W. H. (1991). “Effects of vegetation on methane flux, reservoirs, and carbon isotopic composition,” in Trace Gas. Eds. H. Sharkey, T. Holland, E. Mooney. San Diego, CA: Academic Press.
Chanton, J. P., Whiting, G. J., Happell, J. D., and Gerard, G. (1993). Contrasting rates and diurnal patterns of methane emission from emergent aquatic macrophytes. Aquat. Bot. 46, 111–128. doi: 10.1016/0304-3770(93)90040-4
Chanton, J. P., Whiting, G. J., Showers, W. J., and Crill, P. M. (1992). Methane flux from Peltandra virginica: stable isotope tracing and chamber effects. Glob. Biogeochem. Cycl. 6, 15–31. doi: 10.1029/91GB02969
Cheng, W. (1999). Rhizosphere feedbacks in elevated CO2. Tree Physiol. 19, 313–320. doi: 10.1093/treephys/19.4-5.313
Chu, H., Chen, J., Gottgens, J. F., Ouyang, Z., John, R., Czajkowski, K., et al. (2014). Net ecosystem methane and carbon dioxide exchanges in a Lake Erie coastal marsh and a nearby cropland. J. Geophy. Res. Biogeosci. 119, 722–740. doi: 10.1002/2013JG002520
Ciais, P., Sabine, C., Bala, G., and Peters, W. (2014). “Carbon and other biogeochemical cycles. in Climate change 2013: the physical science basis,” in Contribution of Working Group I to the Fifth Assessment Report of the Intergovernmental Panel on Climate Change. Cambridge: Cambridge University Press. p. 465–570.
Clarke, S. J. (2002). Vegetation growth in rivers: influences upon sediment and nutrient dynamics. Prog. Phy. Geography 26, 159–172. doi: 10.1191/0309133302pp324ra
Colmer, T. D. (2003). Long-distance transport of gases in plants: a perspective on internal aeration and radial oxygen loss from roots. Plant Cell Environ. 26, 17–36. doi: 10.1046/j.1365-3040.2003.00846.x
Covey, K. R., and Megonigal, J. P. (2019). Methane production and emissions in trees and forests. New Phytol. 222, 35–51. doi: 10.1111/nph.15624
Crawford, J. T., and Stanley, E. H. (2016). Controls on methane concentrations and fluxes in streams draining human-dominated landscapes. Ecol. Appl. 26, 1581–1591. doi: 10.1890/15-1330
Dacey, J. W. H., and Klug, M. J. (1979). Methane efflux from lake sediments through water lilies. Science 203, 1253–1255. doi: 10.1126/science.203.4386.1253
Davidson, S. J., Sloan, V. L., Phoenix, G. K., and Wagner, R. (2016). Vegetation type dominates the spatial variability in CH4 emissions across multiple Arctic tundra landscapes. Ecosystems 19, 1116–1132. doi: 10.1007/s10021-016-9991-0
Davidson, T. A., Audet, J., Jeppesen, E., Landkildehus, F., Lauridsen, T. L., Søndergaard, M., et al. (2018). Synergy between nutrients and warming enhances methane ebullition from experimental lakes. Nat. Clim. Chang. 8, 156–160. doi: 10.1038/s41558-017-0063-z
Dean, J. F., Middelburg, J. J., Röckmann, T., Aerts, R., Blauw, L. G., Egger, M., et al. (2018). Methane feedbacks to the global climate system in a warmer world. Rev. Geophy. 56, 207–250. doi: 10.1002/2017RG000559
Delwiche, K. B., Knox, S. H., Malhotra, A., Fluet-Chouinard, E., McNicol, G., Feron, S., et al. (2021). FLUXNET-CH4: a global, multi-ecosystem dataset and analysis of methane seasonality from freshwater wetlands. Earth Syst. Sci. Data 13, 3607–3689. doi: 10.5194/essd-13-3607-2021
Desrosiers, K., DelSontro, T., and del Giorgio, P. A. (2022). Disproportionate contribution of vegetated habitats to the CH4 and CO2 budgets of a boreal lake. Ecosystems 25, 1522–1541. doi: 10.1007/s10021-021-00730-9
Dias, A. T. C., Hoorens, B., Van Logtestijn, R. S. P., Vermaat, J. E., and Aerts, R. (2010). Plant species composition can be used as a proxy to predict methane emissions in peatland ecosystems after land-use changes. Ecosystems 13, 526–538. doi: 10.1007/s10021-010-9338-1
Ding, W., Cai, Z., Tsuruta, H., and Li, X. (2002). Effect of standing water depth on methane emissions from freshwater marshes in northeast China. Atmos. Environ. 36, 5149–5157. doi: 10.1016/S1352-2310(02)00647-7
Dingemans, B. J. J., Bakker, E. S., and Bodelier, P. L. E. (2011). Aquatic herbivores facilitate the emission of methane from wetlands. Ecology, 92, 1166–1173. doi: 10.1890/10-1297.1
Donis, D., Flury, S., Stöckli, A., Spangenberg, J. E., Vachon, D., and McGinnis, D. F. (2017). Full-scale evaluation of methane production under oxic conditions in a mesotrophic lake. Nat. Commun. 8, 1–12. doi: 10.1038/s41467-017-01648-4
Emilson, E. J. S., Carson, M. A., Yakimovich, K. M., Osterholz, H., Dittmar, T., Gunn, J. M., et al. (2018). Climate-driven shifts in sediment chemistry enhance methane production in northern lakes. Nat. Commun. 9, 1801. doi: 10.1038/s41467-018-04236-2
Flury, S., McGinnis, D. F., and Gessner, M. O. (2010). Methane emissions from a freshwater marsh in response to experimentally simulated global warming and nitrogen enrichment. J. Geophys. Res. 115:G01007. doi: 10.1029/2009JG001079
Fonseca, A. L., dos, S., Marinho, C. C., and Esteves, F. de A. (2017). Floating aquatic macrophytes decrease the methane concentration in the water column of a Tropical Coastal Lagoon: implications for methane oxidation and emission. Braz. Arch. Biol. Technol. 60, 2017. doi: 10.1590/1678-4324-2017160381
Frenzel, P., and Rudolph, J. (1998). Methane emission from a wetland plant: the role of CH 4 oxidation in Eriophorum. Plant Soil 202, 27–32. doi: 10.1023/A:1004348929219
Fritz, C., Pancotto, V. A., Elzenga, J. T. M., Visser, E. J. W., Grootjans, A. P., Pol, A., et al. (2011). Zero methane emission bogs: extreme rhizosphere oxygenation by cushion plants in Patagonia. New Phytol. 190, 398–408. doi: 10.1111/j.1469-8137.2010.03604.x
Galand, P. E., Fritze, H., Conrad, R., and Yrjäl,ä, K. (2005). Pathways for methanogenesis and diversity of methanogenic archaea in three boreal peatland ecosystems. Appl. Environ. Microbiol. 71, 2195–2198. doi: 10.1128/AEM.71.4.2195-2198.2005
Grasset, C., Abril, G., Mendonça, R., Roland, F., and Sobek, S. (2019). The transformation of macrophyte-derived organic matter to methane relates to plant water and nutrient contents. Limnol. Oceanogr. 64, 1737–1749. doi: 10.1002/lno.11148
Grasset, C., Mendonça, R., Saucedo, G. V., Bastviken, D., Roland, F., and Sobek, S. (2018). Large but variable methane production in anoxic freshwater sediment upon addition of allochthonous and autochthonous organic matter. Limnol. Oceanogr. 63, 1488–1501. doi: 10.1002/lno.10786
Grasset, C., Moras, S., Isidorova, A., Couture, R.-M., Linkhorst, A., and Sobek, S. (2021). An empirical model to predict methane production in inland water sediment from particular organic matter supply and reactivity. Limnol. Oceanogr. 66, 3643–3655. doi: 10.1002/lno.11905
Gregory, P. J., Palta, J. A., and Batts, G. R. (1995). Root systems and root:mass ratio-carbon allocation under current and projected atmospheric conditions in arable crops. Plant Soil, 187, 221–228. doi: 10.1007/BF00017089
Heilman, M. A., and Carlton, R. G. (2001). Methane oxidation associated with submersed vascular macrophytes and its impact on plant diffusive methane flux. Biogeochemistry 52, 207–224. doi: 10.1023/A:1006427712846
Hilt, S., Grossart, H.-P., McGinnis, H. P., and Keppler, F. (2022). Potential role of submerged macrophytes for oxic methane production in aquatic ecosystems. Limnol. Oceanogr. 67, S76–S88. doi: 10.1002/lno.12095
Hodson, E. L., Poulter, B., Zimmermann, N. E., Prigent, C., and Kaplan, J. O. (2011). The El Niño–Southern Oscillation and wetland methane interannual variability. Geophys. Res. Lett. 38. doi: 10.1029/2011GL046861
Holzapfel-Pschorn, A., Conrad, R., and Seiler, W. (1986). Effects of vegetation on the emission of methane from submerged paddy soil. Plant Soil, 92, 223–233. doi: 10.1007/BF02372636
Iguchi, H., Umeda, R., Taga, H., Oyama, T., Yurimoto, H., and Sakai, Y. (2019). Community composition and methane oxidation activity of methanotrophs associated with duckweeds in a fresh water lake. J. Biosci. Bioeng. 128, 450–455. doi: 10.1016/j.jbiosc.2019.04.009
Ito, A. (2021). Bottom-up evaluation of the regional methane budget of northern lands from 1980 to 2015. Polar Sci. 27, 100558. doi: 10.1016/j.polar.2020.100558
Ito, A., and Inatomi, M. (2012). Use of a process-based model for assessing the methane budgets of global terrestrial ecosystems and evaluation of uncertainty. Biogeosciences 9, 759–773. doi: 10.5194/bg-9-759-2012
Jeffrey, L. C., Maher, D. T., Johnston, S. G., Kelaher, B. P., Steven, A., and Tait, D. R. (2019). Wetland methane emissions dominated by plant-mediated fluxes: Contrasting emissions pathways and seasons within a shallow freshwater subtropical wetland. Limnol. Oceanogr. 64, 1895–1912. doi: 10.1002/lno.11158
Joabsson, A., and Christensen, T. R. (2001). Methane emissions from wetlands and their relationship with vascular plants: an Arctic example. Glob. Chang. Biol. 7, 919–932. doi: 10.1046/j.1354-1013.2001.00044.x
Joabsson, A., Christensen, T. R., and Wallén, B. (1999). Vascular plant controls on methane emissions from northern peatforming wetlands. Trends Ecol. Evol. 14, 385–388. doi: 10.1016/S0169-5347(99)01649-3
Johnson, M. S., Matthews, E., Du, J., Genovese, V., and Bastviken, D. (2022). Methane emission from global lakes: new spatiotemporal data and observation-driven modeling of methane dynamics indicates lower emissions. J. Geophy. Res. Biogeosci. 127, e2022JG006793. doi: 10.1029/2022JG006793
Juutinen, S., Alm, J., Larmola, T., Huttunen, J. T, Morero, M., Martikainen, P. J., et al. (2003). Major implication of the littoral zone for methane release from boreal lakes. Global Biogeochem. Cycles 17:1117. doi: 10.1029/2003GB002105
Kankaala, P., Käki, T., Mäkel,ä, S, Ojala, A., Pajunen, H., and Arvola, L. (2005). Methane efflux in relation to plant biomass and sediment characteristics in stands of three common emergent macrophytes in boreal mesoeutrophic lakes. Glob. Chang. Biol. 11, 145–153. doi: 10.1111/j.1365-2486.2004.00888.x
Kankaala, P., Käki, T., and Ojala, A. (2003). Quality of detritus impacts on spatial variation of methane emissions from littoral sediment of a boreal lake. Arch. Hydrobiol. 157, 47–66. doi: 10.1127/0003-9136/2003/0157-0047
Kankaala, P., Ojala, A., and Käki, T. (2004). Temporal and spatial variation in methane emissions from a flooded transgression shore of a boreal lake. Biogeochemistry 68, 297–311. doi: 10.1023/B:BIOG.0000031030.77498.1f
Keppler, F., Hamilton, J. T. G., Bra,ß, M, and Röckmann, T. (2006). Methane emissions from terrestrial plants under aerobic conditions. Nature 439, 187–191. doi: 10.1038/nature04420
Keppler, F., Hamilton, J. T. G., McRoberts, W. C., Vigano, I., Braß, M., and Röckmann, T. (2008). Methoxyl groups of plant pectin as a precursor of atmospheric methane: evidence from deuterium labelling studies. New Phytol. 178, 808–814. doi: 10.1111/j.1469-8137.2008.02411.x
Kim, J., Verma, S. B., Billesbach, D. B., and Clement, R. J. (1998). Diel variation in methane emission from a midlatitude prairie wetland: significance of convective throughflow in Phragmites australis. J. Geophy. Res. Atmosph. 103, 28029–28039. doi: 10.1029/98JD02441
Kim, J., Verma, S. B., and Billesbach, D. P. (1999). Seasonal variation in methane emission from a temperate Phragmites-dominated marsh: Effect of growth stage and plant-mediated transport. Glob. Chang. Biol. 5, 433–440. doi: 10.1046/j.1365-2486.1999.00237.x
King, G. M. (1990). Regulation by light of methane emissions from a wetland. Nature 345, 513–515. doi: 10.1038/345513a0
Kleinen, T., Mikolajewicz, U., and Brovkin, V. (2020). Terrestrial methane emissions from the Last Glacial Maximum to the preindustrial period. Clim. Past 16, 575–595. doi: 10.5194/cp-16-575-2020
Knoblauch, C., Spott, O., Evgrafova, S., Kutzbach, L., and Pfeiffer, E-. M. (2015). Regulation of methane production, oxidation, and emission by vascular plants and bryophytes in ponds of the northeast Siberian polygonal tundra. J. Geophy. Res. Biogeosci. 120, 2525–2541. doi: 10.1002/2015JG003053
Kosten, S., Piñeiro, M., de Goede, E., de Klein, J., Lamers, L. P. M., and Ettwig, K. (2016). Fate of methane in aquatic systems dominated by free-floating plants. Water Res. 104, 200–207. doi: 10.1016/j.watres.2016.07.054
Kuhn, C., Bettigole, C., Glick, H. B., Seegmiller, L., Oliver, C. D., and Raymond, P. (2017). Patterns in stream greenhouse gas dynamics from mountains to plains in northcentral Wyoming. J. Geophy. Res. Biogeosci. 122, 2173–2190. doi: 10.1002/2017JG003906
Kyzivat, E. D., Smith, L. C., Garcia-Tigreros, F., Huang, C., Wang, C., Langhorst, T., et al. (2022). The importance of lake emergent aquatic vegetation for estimating arctic-boreal methane emissions. J. Geophy. Res. Biogeosci. 127, e2021JG006635. doi: 10.1029/2021JG006635
Laanbroek, H. J. (2010). Methane emission from natural wetlands: interplay between emergent macrophytes and soil microbial processes. A mini-review. Ann. Bot. 105, 141–153. doi: 10.1093/aob/mcp201
Larmola, T., Alm, J., Juutinen, S., Huttunen, J. T., Martikainen, P. J., and Silvola, J. (2004). Contribution of vegetated littoral zone to winter fluxes of carbon dioxide and methane from boreal lakes. J. Geophys. Res. 109:D19102. doi: 10.1029/2004JD004875
Leakey, A. D. B. (2009). Rising atmospheric carbon dioxide concentration and the future of C4 crops for food and fuel. Proc. R. Soc. B Biol. Sci. 276, 2333–2343. doi: 10.1098/rspb.2008.1517
Lenth, R. (2019). Estimated Marginal Means, aka Least-Squares Means. R Package Version 1.3. 5.1 R package.
Liu, S., Chen, Y., and Liu, J. (2019). Methane emissions from the littoral zone of Poyang lake during drawdown periods. J. Freshw. Ecol. 34, 37–48. doi: 10.1080/02705060.2018.1537941
Marinho, C. C., Palma-Silva, C., Albertoni, E. F., Giacomini, I. B., Barros, M. P. F., Furlanetto, L. M., et al. (2015). Emergent macrophytes alter the sediment composition in a small, shallow subtropical lake: implications for methane emission. Am. J. Plant Sci. 6, 315. doi: 10.4236/ajps.2015.62036
Mei, D., Ni, M., Liang, X., Hou, L., Wang, F., and He, C. (2020). Filamentous green algae Spirogyra regulates methane emissions from eutrophic rivers. Environ. Sci. Pollut. Res. 28, 3660–3671. doi: 10.1007/s11356-020-10754-8
Melack, J. M., and Hess, L. L. (2023). Areal extent of vegetative cover: a challenge to regional upscaling of methane emissions. Aquat. Bot. 184, 103592. doi: 10.1016/j.aquabot.2022.103592
Melton, J. R., Wania, R., Hodson, E. L., Poulter, B., Ringeval, B., Spahni, R., et al. (2013). Present state of global wetland extent and wetland methane modelling: conclusions from a model intercomparison project (WETCHIMP). Biogeosciences 10, 753–788. doi: 10.5194/bg-10-753-2013
Netten, J. J. C., van Zuidam, J., Kosten, S., and Peeters, E. T. H. M. (2011). Differential response to climatic variation of free-floating and submerged macrophytes in ditches. Freshw. Biol. 56, 1761–1768. doi: 10.1111/j.1365-2427.2011.02611.x
Oliveira-Junior, E. S., Tang, Y., van den Berg, S. J. P., Cardoso, S. J., Lamers, L. P. M., and Kosten, S. (2018). The impact of water hyacinth (Eichhornia crassipes) on greenhouse gas emission and nutrient mobilization depends on rooting and plant coverage. Aquat. Bot. 145, 1–9. doi: 10.1016/j.aquabot.2017.11.005
Oliveira-Junior, E. S., van Bergen, T. J. H. M., Nauta, J., Budiša, A., Aben, R. C. H., Weideveld, S. T. J., et al. (2020). Water Hyacinth's effect on greenhouse gas fluxes: a field study in a wide variety of tropical water bodies. Ecosystems 24, 988–1004. doi: 10.1007/s10021-020-00564-x
Öquist, M. G., and Svensson, B. H. (2002). Vascular plants as regulators of methane emissions from a subarctic mire ecosystem. J. Geophys. Res. 107:4580. doi: 10.1029/2001JD001030
Parker, R. J., Boesch, H., McNorton, J., Comyn-Platt, E., Gloor, M., Wilson, C., et al. (2018). Evaluating year-to-year anomalies in tropical wetland methane emissions using satellite CH4 observations. Remote Sens. Environ. 211, 261–275. doi: 10.1016/j.rse.2018.02.011
Peeters, E. T. H. M., van Zuidam, J. P., van Zuidam, B. G., Van Nes, E. H., Kosten, S., Heuts, P. G. M., et al. (2013). Changing weather conditions and floating plants in temperate drainage ditches. J. Appl. Ecol. 50, 585–593. doi: 10.1111/1365-2664.12066
Peltola, O., Vesala, T., Gao, Y., Räty, O., Alekseychik, P., Aurela, M., et al. (2019). Monthly gridded data product of northern wetland methane emissions based on upscaling eddy covariance observations. Earth Syst. Sci. Data 11, 1263–1289. doi: 10.5194/essd-11-1263-2019
Petruzzella, A., Guariento, R. D., da Rocha Gripp, A., Marinho, C. C., Figueiredo-Barros, M. P., and de Assis Esteves, F. (2015). Herbivore damage increases methane emission from emergent aquatic macrophytes. Aquat. Bot. 127, 6–11. doi: 10.1016/j.aquabot.2015.07.003
Raghoebarsing, A. A., Smolders, A. J. P., Schmid, M. C., Rijpstra, W. I. C., Wolters-Arts, M., Derksen, J., et al. (2005). Methanotrophic symbionts provide carbon for photosynthesis in peat bogs. Nature 436, 1153–1156. doi: 10.1038/nature03802
Ribaudo, C., Bartoli, M., Longhi, D., and Castaldi, S. (2012). CO2 and CH4 fluxes across a Nuphar lutea (L.) Sm. stand. J. Limnol. 71, 21. doi: 10.4081/mnol.2012.e21
Riley, W. J., Subin, Z. M., Lawrence, D. M., Swenson, S. C., Torn, M. S., Meng, L., et al. (2011). Barriers to predicting changes in global terrestrial methane fluxes: analyses using CLM4Me, a methane biogeochemistry model integrated in CESM. Biogeosciences 8, 1925–1953. doi: 10.5194/bg-8-1925-2011
Robroek, B. J. M., Jassey, V. E. J., Beltman, B., and Hefting, M. M. (2017). Diverse fen plant communities enhance carbon-related multifunctionality, but do not mitigate negative effects of drought. R. Soc. Open Sci. 4, 170449. doi: 10.1098/rsos.170449
Robroek, B. J. M., Jassey, V. E. J., Kox, M. A. R., Berendsen, R. L., Mills, R. T. E., Cécillon, L., et al. (2015). Peatland vascular plant functional types affect methane dynamics by altering microbial community structure. J.Ecol. 103, 925–934. doi: 10.1111/1365-2745.12413
Rocher-Ros, G., Stanley, E. H., Loken, L. C., Casson, N. J., Raymond, P. A., Liu, S., et al. (2023). Global methane emissions from rivers and streams. Nature 621, 530–535. doi: 10.1038/s41586-023-06344-6
Rosentreter, J. A., Borges, A. V., Deemer, B. R., Holgerson, M. A., Liu, S., Song, C., et al. (2021). Half of global methane emissions come from highly variable aquatic ecosystem sources. Nat. Geosci. 14, 225–230. doi: 10.1038/s41561-021-00715-2
Rosentreter, J. A., Maher, D. T., Erler, D. V., Murray, R. H., and Eyre, B. D. (2018). Methane emissions partially offset “blue carbon” burial in mangroves. Sci. Adv. 4, eaao4985. doi: 10.1126/sciadv.aao4985
Sanches, L. F., Guenet, B., Marinho, C. C., Barros, N., and de Assis Esteves, F. (2019). Global regulation of methane emission from natural lakes. Sci. Rep. 9, 1–10. doi: 10.1038/s41598-018-36519-5
Sanchez, J. M., Arijo, S., Muñoz, M. A., Moriñigo, M. A., and Borrego, J. J. (1994). Microbial colonization of different support materials used to enhance the methanogenic process. Appl. Microbiol. Biotechnol. 41, 480–486. doi: 10.1007/BF00939040
Sanders, I. A., Heppell, C. M., Cotton, J. A., Wharton, G., Hildrew, A. G., Flowers, E. J., et al. (2007). Emission of methane from chalk streams has potential implications for agricultural practices. Freshw. Biol. 52, 1176–1186. doi: 10.1111/j.1365-2427.2007.01745.x
Sand-Jensen, K., and Pedersen, O. (1999). Velocity gradients and turbulence around macrophyte stands in streams. Freshw. Biol. 42, 315–328. doi: 10.1046/j.1365-2427.1999.444495.x
Saunois, M., Bousquet, P., Poulter, B., Peregon, A., Ciais, P., Canadell, J. G., et al. (2017). Variability and quasi-decadal changes in the methane budget over the period 2000–2012. Atmosph. Chem. Phy. 17, 11135–11161. doi: 10.5194/acp-17-11135-2017
Saunois, M., Stavert, A. R., Poulter, B., Bousquet, P., Canadell, J. F., Jackson, R. B., et al. (2020). The global methane budget 2000–2017. Earth System Science Data, 12, 1561–1623. doi: 10.5194/essd-12-1561-2020
Schimel, J. P. (1995). Plant transport and methane production as controls on methane flux from arctic wet meadow tundra. Biogeochemistry, 28, 183–200. doi: 10.1007/BF02186458
Sebacher, D. I., Harriss, R. C., and Bartlett, K. B. (1985). Methane emissions to the atmosphere through aquatic plants 1. J. Environ. Qual. 14, 40–46. doi: 10.2134/jeq1985.00472425001400010008x
Segers, R. (1998). Methane production and methane consumption: a review of processes underlying wetland methane fluxes. Biogeochemistry 41, 23–51. doi: 10.1023/A:1005929032764
Shih, J. G., and Finkelstein, S. A. (2008). Range dynamics and invasive tendencies in Typha latifolia and Typha angustifolia in eastern North America derived from herbarium and pollen records. Wetlands 28, 1–16. doi: 10.1672/07-40.1
Silva, T. S. F., Costa, M. P. F., Melack, J. M., and Novo, E. M. L. M. (2008). Remote sensing of aquatic vegetation: theory and applications. Environ. Monit. Assess. 140, 131–145. doi: 10.1007/s10661-007-9855-3
Silva, J. P., Canchala, T. R., Lubberding, H. J., Peña, E. J., and Gijzen, H. J. (2016). Greenhouse gas emissions from a tropical eutrophic freshwater wetland. World Acad. Sci. Eng. Technol. Int. J. Environ. Chem. Ecol. Geol. Geophy. Engin. 10, 541–547.
Sorrell, B. K., Downes, M. T., and Stanger, C. L. (2002). Methanotrophic bacteria and their activity on submerged aquatic macrophytes. Aquat. Bot. 72, 107–119. doi: 10.1016/S0304-3770(01)00215-7
Stepniewska, Z., Goraj, W., Kuzniar, A., Szafranek-Nakonieczna, A., Banach, A., Górski, A., et al. (2018). Methane oxidation by endophytic bacteria inhabiting Sphagnum sp. and some vascular plants. Wetlands, 38, 411–422. doi: 10.1007/s13157-017-0984-3
Ström, L., Ekberg, A., Mastepanov, M., and Christensen, T. R. (2003). The effect of vascular plants on carbon turnover and methane emissions from a tundra wetland. Glob. Chang. Biol. 9, 1185–1192. doi: 10.1046/j.1365-2486.2003.00655.x
Ström, L., Mastepanov, M., and Christensen, T. R. (2005). Species-specific effects of vascular plants on carbon turnover and methane emissions from wetlands. Biogeochemistry 75, 65–82. doi: 10.1007/s10533-004-6124-1
Struik, Q., Oliveira Junior, E. S., Veraart, A. J., and Kosten, S. (2022). Methane emissions through water hyacinth are controlled by plant traits and environmental conditions. Aquat. Bot. 183, 103574. doi: 10.1016/j.aquabot.2022.103574
Sugimoto, A., and Fujita, N. (1997). Characteristics of methane emission from different vegetations on a wetland. Tellus B Chem. Phy. Meteorol. 49, 382–392. doi: 10.3402/tellusb.v49i4.15976
Thottathil, S. D., Reis, P. C. J., del Giorgio, P. A., and Prairie, Y. T. (2018). The extent and regulation of summer methane oxidation in Northern Lakes. J. Geophy. Res. Biogeosci. 123, 3216–3230. doi: 10.1029/2018JG004464
Tong, C., Wang, W.-Q., Huang, J.-F., Gauci, V., Zhang, L.-H., and Zeng, C.-S. (2012). Invasive alien plants increase CH 4 emissions from a subtropical tidal estuarine wetland. Biogeochemistry 111, 677–693. doi: 10.1007/s10533-012-9712-5
Turner, J. C., Moorberg, C. J., Wong, A., Shea, K., Waldrop, M. P., Turetsky, M. R., et al. (2020). Getting to the root of plant-mediated methane emissions and oxidation in a thermokarst bog. J. Geophy. Res. Biogeosci. 125, e2020JG005825. doi: 10.1029/2020JG005825
van Bergen, T. J. H. M., Barros, N., Mendonça, R., Aben, R. C. H., Althuizen, I. H. J., Huszar, V., et al. (2019). Seasonal and diel variation in greenhouse gas emissions from an urban pond and its major drivers. Limnol. Oceanogr. 64, 2129–2139. doi: 10.1002/lno.11173
van Bodegom, P. M., Groot, T., van den Hout, B., Leffelaar, P. A., and Goudriaan, J. (2001). Diffusive gas transport through flooded rice systems. J. Geophy. Res. Atmospheres 106, 20861–20873. doi: 10.1029/2001JD900176
van den Berg, M., van den Elzen, E., Ingwersen, J., Kosten, S., Lamers, L. P. M., and Streck, T. (2020). Contribution of plant-induced pressurized flow to CH4 emission from a Phragmites fen. Sci. Rep. 10, 1–10. doi: 10.1038/s41598-020-69034-7
van der Nat, F.-J., and Middelburg, J. J. (2000). Methane emission from tidal freshwater marshes. Biogeochemistry 49, 103–121. doi: 10.1023/A:1006333225100
van der Nat, F.-J. W. A., and Middelburg, J. J. (1998). Seasonal variation in methane oxidation by the rhizosphere of Phragmites australis and Scirpus lacustris. Aquat. Bot. 61, 95–110. doi: 10.1016/S0304-3770(98)00072-2
Villa, J. A., Ju, Y., Stephen, T., Rey-Sanchez, C., Wrighton, K. C., and Bohrer, G. (2020). Plant-mediated methane transport in emergent and floating-leaved species of a temperate freshwater mineral-soil wetland. Limnol. Oceanogr. 65, 1635–1650. doi: 10.1002/lno.11467
Visser, E. J. W., Colmer, T. D., Blom, C. W. P. M., and Voesenek, L. A. C. J. (2000). Changes in growth, porosity, and radial oxygen loss from adventitious roots of selected mono-and dicotyledonous wetland species with contrasting types of aerenchyma. Plant Cell Environ. 23, 1237–1245. doi: 10.1046/j.1365-3040.2000.00628.x
Vroom, R. J. E., van den Berg, M., Pangala, S. R., van der Scheer, O. E., and Sorrell, B. K. (2022). Physiological processes affecting methane transport by wetland vegetation – a review. Aquat. Bot. 182, 103547. doi: 10.1016/j.aquabot.2022.103547
Walter, B. P., and Heimann, M. (2000). A process-based, climate-sensitive model to derive methane emissions from natural wetlands: Application to five wetland sites, sensitivity to model parameters, and climate. Glob. Biogeochem. Cycl. 14, 745–765. doi: 10.1029/1999GB001204
Wang, B., Neue, H. U., and Samonte, H. P. (1997). Role of rice in mediating methane emission. Plant Soil 189, 107–115. doi: 10.1023/A:1004219024281
Wang, H., Lu, J., Wang, W., Yang, L., and Yin, C. (2006). Methane fluxes from the littoral zone of hypereutrophic Taihu Lake, China. J. Geophy. Res.Atmos. 111, D17109. doi: 10.1029/2005JD006864
Wang, Z., Keppler, F., Greule, M., and Hamilton, J. T. G. (2011). Non-microbial methane emissions from fresh leaves: effects of physical wounding and anoxia. Atmos. Environ. 45, 4915–4921. doi: 10.1016/j.atmosenv.2011.06.001
Webster, J. R., and Benfield, E. F. (1986). Vascular plant breakdown in freshwater ecosystems. Annu. Rev. Ecol. Syst. 17, 567–594. doi: 10.1146/annurev.es.17.110186.003031
Whiting, G. J., and Chanton, J. P. (1996). Control of the diurnal pattern of methane emission from emergent aquatic macrophytes by gas transport mechanisms. Aquat. Bot. 54, 237–253. doi: 10.1016/0304-3770(96)01048-0
Yavitt, J. B., and Knapp, A. K. (1995). Methane emission to the atmosphere through emergent cattail (Typha latifolia L.) plants. Tellus B Chem. Phy. Meteorol. 47, 521–534. doi: 10.3402/tellusb.v47i5.16065
Yoshida, N., Iguchi, H., Yurimoto, H., Murakami, A., and Sakai, Y. (2014). Aquatic plant surface as a niche for methanotrophs. Front. Microbiol. 5, 30. doi: 10.3389/fmicb.2014.00030
Zhang, B., Tian, H., Lu, C., Chen, G., Pan, S., Anderson, C., et al. (2017). Methane emissions from global wetlands: An assessment of the uncertainty associated with various wetland extent data sets. Atmos. Environ. 165, 310–321. doi: 10.1016/j.atmosenv.2017.07.001
Zhang, Y., Jeppesen, E., Liu, X., Qin, B., Shi, K., Zhou, Y., et al. (2017). Global loss of aquatic vegetation in lakes. Earth Sci. Rev. 173, 259–265. doi: 10.1016/j.earscirev.2017.08.013
Zhuang, Q., Guo, M., Melack, J. M., Lan, X., Tan, Z., Oh, Z., et al. (2023). Current and future global lake methane emissions: a process-based modeling analysis. J. Geophy. Res. Biogeosci. 128, e2022JG007137. doi: 10.1029/2022JG007137
Keywords: carbon cycle, freshwaters, greenhouse gas, lakes, macrophytes, plant-mediated, rivers, wetlands
Citation: Bodmer P, Vroom RJE, Stepina T, del Giorgio PA and Kosten S (2024) Methane dynamics in vegetated habitats in inland waters: quantification, regulation, and global significance. Front. Water 5:1332968. doi: 10.3389/frwa.2023.1332968
Received: 03 November 2023; Accepted: 13 December 2023;
Published: 17 January 2024.
Edited by:
Ralf Kiese, Karlsruhe Institute of Technology (KIT), GermanyReviewed by:
Haohao Wu, Nanjing Institute of Geography and Limnology (CAS), ChinaGnanamoorthy Palingamoorthy, Chinese Academy of Sciences (CAS), China
Copyright © 2024 Bodmer, Vroom, Stepina, del Giorgio and Kosten. This is an open-access article distributed under the terms of the Creative Commons Attribution License (CC BY). The use, distribution or reproduction in other forums is permitted, provided the original author(s) and the copyright owner(s) are credited and that the original publication in this journal is cited, in accordance with accepted academic practice. No use, distribution or reproduction is permitted which does not comply with these terms.
*Correspondence: Pascal Bodmer, Ym9kbWVycGFzY2FsQGdtYWlsLmNvbQ==
†Present address: Tatiana Stepina, Siberian Federal University, Krasnoyarsk, Russia
‡These authors have contributed equally to this work and share first authorship