- 1Water Research Center (WRC), Kuwait Institute for Scientific Research, Safat, Kuwait
- 2School of Engineering, Cardiff University, Cardiff, United Kingdom
- 3Department of Chemical Engineering, University of Bath, Bath, United Kingdom
- 4Water Innovation and Research Centre (WIRC), University of Bath, Bath, United Kingdom
- 5GW4 NERC CDT in Freshwater Biosciences and Sustainability, Cardiff University, Cardiff, United Kingdom
- 6School of Civil Engineering and Water at Leeds, University of Leeds, Leeds, United Kingdom
- 7School of Biosciences, Cardiff University, Cardiff, United Kingdom
A multistage mesocosm vertical flow constructed wetland system was designed to treat synthetic domestic wastewater with a high nitrogen (N) load. The study aim was to determine the impact of design and operational variables on N removal efficiency in such systems. A tidal flow operational strategy enhanced aeration and was coupled with a step-feeding approach to promote N removal. Over the 420-day running period N removal rates were between 70 and 77 gN/m3/d, for a step-feeding ratio range of 60:40 to 80:20. The system was able to remove 91–95% of chemical oxygen demand, 74–91% of ammonium and 66–81% of total-N. Tidal flow and step-feeding strategies significantly impacted nitrogen removal with the best performance at a step-feeding ratio of 80:20 providing a carbon to nitrogen (COD/N) ratio of 4–5. The bacterial diversity increased at each stage throughout the system with dominating phyla Proteobacteria, Firmicutes, Planctomycetes, Bacteroidetes, Chloroflexi, Verrucomicrobia and Acidobacteria. Dominant bacteria at the genus level were Thiothrix, Planctomyces, Azonexus, Pseudoxanthomonas, Hydrogenophaga, Gemmobacter and other genera suggesting that N removal was accomplished via diverse metabolic pathways, including autotrophic nitrification, heterotrophic denitrification, autotrophic denitrification, and possibly anammox. This study shows benefits of step-feeding strategies in tidal flow constructed wetlands as a cost-effective solution for minimizing external carbon input to achieve effective N removal.
1. Introduction
Use of constructed wetlands (CWs) for wastewater treatment has increased rapidly in recent years, especially for small communities, rural areas, and villages (Chen, 2011; Wu et al., 2014; Garfí et al., 2017; Moreira and Dias, 2020; Li et al., 2021). CWs are engineered systems that are used worldwide for their low operation and maintenance costs, low energy and carbon footprints, and ease of operation (Wu et al., 2014; Wang et al., 2016; Tan et al., 2020). CWs have been successfully adopted to treat various types of wastewater including municipal, agricultural or industrial wastewater (Masi et al., 2018; Lekshmi et al., 2020). Beyond removing a wide range of contaminants from water, CWs also provide an array of social and environmental benefits such as a recreational zones, biodiverse habitats, or wildlife refuge and breeding grounds (Stefanakis et al., 2014; Dumax and Rozan, 2021). CWs utilize microbial mediated removal pathways to treat biodegradable contaminants such as nitrogen (N) or chemical oxygen demand (COD) (Kulshreshtha et al., 2022).
N removal in CWs is a complex process and is commonly accomplished by microbial nitrification-denitrification (Lu et al., 2020). CWs N removal potential can be further augmented with anaerobic ammonium oxidation (anammox) to overcome limitations of carbon availability encountered in denitrification processes (Negi et al., 2022). Complete nitrogen removal requires an efficient nitrification process to transform ammonium () under aerobic conditions using autotrophic aerobic bacteria. Followed by the elimination of nitrate () by denitrification using autotrophic and heterotrophic anaerobic bacteria with an adequate organic carbon source (Kadlec and Knight, 1996; Vymazal, 2007; Wang et al., 2016). Typically, the removal of total nitrogen (TN) in CWs ranges between 30–50% and thus does not always provide the required removal effectiveness, particularly for heavily polluted wastewater (Lee et al., 2009; Vymazal and Kröpfelová, 2009; Ruan et al., 2021; Negi et al., 2022). Nitrogen removal in CWs is often limited by the lack of readily available organic carbon sources for the denitrification process (Pelissari et al., 2014). Denitrification efficiency may be enhanced by the addition of external carbon sources such as biochar or agricultural by-products (Yu et al., 2019; Zheng et al., 2022) or iron addition as electron donor to ensure occurrence of both reductive and anoxic conditions (Zhuang et al., 2019). Besides external carbon loading, enhanced productivity of nitrifying/denitrifying communities in CWs can be achieved via control of oxygen supply to create suitable aerobic/anaerobic conditions. Possible solutions include artificial aeration, step-feeding, wastewater recirculation or hybrid designs that combining nitrification and denitrification advantages vertical and horizontal flow CWs (Vymazal, 2007; Ye and Li, 2009; Ávila et al., 2017; Ilyas and Masih, 2017; Jehawi et al., 2020). Nevertheless, most enhanced nitrogen removal modifications result in increased operational costs due to energy input (aeration) or carbon dosing, or require larger area footprints, e.g., for hybrid systems (Vymazal, 2013).
Tidal flow CWs (TFCWs) have been introduced as a compromise between artificial aeration and multi-stage hybrid systems (Saeed et al., 2020). TFCWs are vertical flow CWs (VFCWs) designed to operate under alternating water level conditions. The operation cycle includes wet phases during which the system is filled up with water and dry phases when the system is drained. During wet phases, the intruding water expels the air form the substrate matrix and creates temporarily anoxic/anaerobic conditions suitable for denitrification. Subsequently, dry phases create passively aerated aerobic conditions when lowering the water table drains the wetland and allows atmospheric air into the bed matrix. TFCWs have been achieving over 80% removal of TN operating effectively even in cold climates and under low carbon/nitrogen (C/N) conditions due to anammox microbial community that perform a low carbon nitrogen removal pathway (Hu et al., 2014; Pang et al., 2015; Ji et al., 2020). TFCWs are found to be less vulnerable to bioclogging due to the shear stress of the fluctuating water table that contains the growth of biofilm (Zhuang et al., 2019).
Despite benefits of the tidal flow operation mode, the implementation of TFCW is limited due to the complex management, as tidal phases need adjusting to the incoming wastewater quality to meet the required oxygen supply rate and provide a balanced carbon pool. Some of the limitations of TFCWs can be solved by an adjusted step-feeding strategy that enhances nitrification and effectively closes denitrification carbon demand. Stepwise introduction of the influent to already nitrified wastewater leads to more efficient use of the influent carbon source for the denitrification process (Tang et al., 2007; Hu et al., 2012) and has been previously tested in TFCWs. Limited literature compares different step-feeding ratios to assess the optimum operation for managing wastewater dosage and distribution points in the system. Therefore, the aim of this study was to investigate the operation of a multistage TFCW that allowed applying a step-feeding strategy. Multistage design divides CW into zones/stages that offers different treatment condition (i.e., oxygen levels, carbon pool) thus enabling treatment of different types of contaminants within same CW system. The TFCW investigated in this study is divided into 4 stages in series where first stage is designed for organic matter removal and the initiation of the nitrification step, the second stage is for the nitrification and denitrification steps, and the third stage and fourth stages (depending on the load) are incorporated to enable effective step-feeding distribution.
The study investigated a range of step-feeding ratios to assess optimum working conditions related to carbon source distribution in the system. The study was carried out at mesocosm TFCW fed with synthetic domestic wastewater (with elevated carbon and nitrogen concentration of approx. 700 mgCOD/l and 60 mg-N/l respectively) for 420 days to obtain a better control on the system and enable direct comparison between the applied step-feeding rations. A microbial analysis was carried out to identify the bacterial community structure and establish possible links with the observed CW performance. This study expands knowledge on TFCWs functions and operation and delivers results that will strengthen the position of TFCWs as an alternative for some of conventional wastewater treatment systems as well as hybrid CWs that characterize with higher area-footprint.
2. Materials and methods
2.1. System description and operation
The CW system consists of four identical stages made from gray PVC plastic with each stage being 100 cm in height and 10 cm in diameter with a total treatment surface area of 0.0.032 m2 (0.008 m2 each) with 80 liters influent tank filled with 60 liters to feed the system and 80 liters effluent tank to collect the final effluent (Figure 1). The experiment was constructed outdoors at the School of Engineering, Cardiff University. The experiment was run throughout the varying seasonal conditions with the daily mean air temperatures ranging from 5–25°C between winter and summer periods respectively. Water samples temperature fluctuated less significantly ranging from 10–20°C between winter and summer periods, respectively. Gravel was used as the main substrate in all four stages. In each stage, a depth of 10 cm in the bottom layer was filled with coarse gravel (20–25 mm). This served as the supporting and drainage layer; the following layer was filled with gravel (4–9 mm) as the main substrate layer with a depth of 50 cm; a 10 cm top layer of gravel (10–19 mm) was added to facilitate the dispersion and the distribution of wastewater and the growth of plants. The porosity of the gravel was 40% combined for the bottom and main substrate layer. Each stage was planted with Phragmites Australis at the beginning of the experiment, and good growth with lush vegetation was observed after 2 months by feeding the system with synthetic wastewater. Phragmites Australis were chosen as these are the most commonly used reference plant for CWs in Europe and are able to survive in most conditions (Brix, 1994; Sun et al., 2005; Kadlec and Wallace, 2008; Vymazal, 2010). Moreover, these plants provide a comparatively high oxygen transfer into the rhizosphere, which facilitates the aerobic degradation of pollutants (Barbera et al., 2009; Wang et al., 2012). The system was fed with synthetic domestic wastewater prepared freshly each week via the peristaltic pumps from the influent tank. About 60 liters of synthetic wastewater were required to feed the system for 1 week. The system was operated with three batch cycles per day, with each cycle entailing 2 h saturation and 6 h unsaturation giving a total of 8 h per cycle. The synthetic wastewater simulates typical domestic wastewater with a high concentration of organic carbon source and nitrogen to obtain approximately 700 mg/l of COD and 60 mg/l of -N. Two liters of room temperature (circa 20°C) synthetic wastewater were pumped into the system in each cycle, totaling 6 liters per day being actively pumped into the system. The synthetic wastewater was batch loaded to the first stage and sequentially passed through the other stages, generating alternate wet/dry periods in individual stages. Before starting the experiment and loading the synthetic wastewater to the CW, the system was inoculated with activated flocs obtained from the aeration basin of a local domestic wastewater treatment plant for about 2 weeks to provide seed microorganisms for the system.
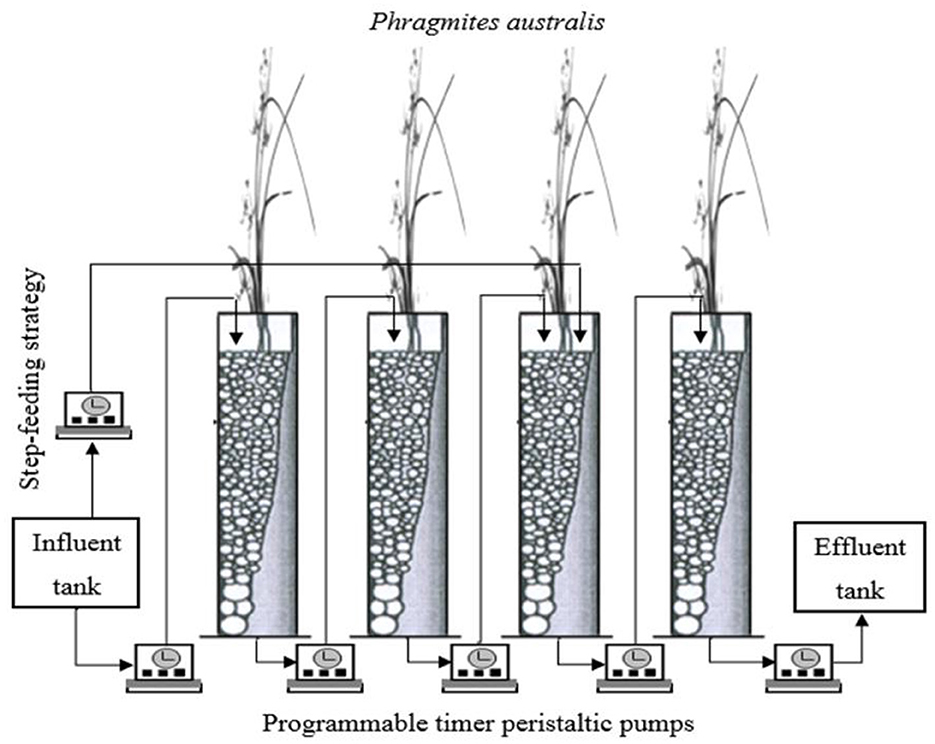
Figure 1. Schematic of the mesocosm constructed wetland system gray PVC plastic used in this study. About 80 liters influent tank was filled with 60 liters to feed the system for one week and 80 liters effluent tank to collect the final effluent from the system by using programmable timer peristaltic pumps.
The experiment was divided into four phases: Phase 1 with only tidal flow, Phase 2 with tidal flow and step-feeding ratio (80:20), Phase 3 with tidal flow and step-feeding ratio (70:30), and Phase 4 with tidal flow and step-feeding ratio (60:40). Step-feeding ratios during experimental Phase 2 to 4 were distributed from the influent reservoir into the third stage of the CW as shown in Figure 1. In these phases, a portion of the flow will be allowed into the first and second stage as normal, and the remainder will be added directly from the reservoir into the system once the synthetic wastewater finishes passing through from the first and second stages to reach the third stage. Samples were collected once a week from the influent tank and the effluent of each stage and analyzed directly in situ for pH and temperature using a pH/EC/TDS meter (HANNA HI 991301). Chemical oxygen demand (COD), nitrite-nitrogen (NO2−-N), nitrate-nitrogen (-N), ammonium-nitrogen (-N) and total nitrogen (TN) were analyzed using a Hach DR/3900 spectrophotometer and digester in the laboratory.
2.2. Tidal flow and step-feeding strategies
A tidal flow strategy was generated in each stage using peristaltic pumps, which were controlled by specific programmable timers (Williamson Pumps Ltd & Williamson Manufacturing Company Ltd.; model: CM type variable speed cased pump). This process repeatedly allowed the mesocosm CWs to be filled with synthetic wastewater until the main media layer fully submerged (wet saturated conditions) and subsequently drained after a desired time (dry unsaturated conditions). Whilst, the additional oxygen availability resulted in improved nitrification it creates less favorable conditions for the denitrification step (Vymazal and Kröpfelová, 2011; Li et al., 2015). Therefore, a step-feeding strategy was adopted at later stages to improve the TN reduction (denitrification step) by introducing the influent synthetic wastewater to the nitrified liquid. Such approaches may provide a more efficient use of the available carbon source that could enhance the denitrification step (Miyaji et al., 1980; Fillos et al., 1996; Puig et al., 2004; Hu et al., 2012).
2.3. DNA extraction and microbial community analysis
Gravel samples were taken from the lower (L) and upper (U) position of the main substrate from each of the four stages of the multistage CW system after 420 days (at the end of experimental Phase 4) and stored at −80°C. Genomic DNA was extracted from the gravel samples (1L, 1U, 2L, 2U, 3L, 3U, 4L, and 4U) using a Meta-G-Nome™ DNA Isolation Kit (Cambio Ltd). Gravel (10 g) was placed in a 20 ml sterile tube with 1 ml of 0.2% Tween 20 Wash Buffer (Cambio Ltd) and shaken for 10 min on a wrist action shaker at maximum speed (to remove the gravel microbial biofilm). The cell suspension was then transferred to a sterile 1.5 ml NoStick tube (Alpha Laboratories), centrifuged at 14,000 × g for 2 mins, and DNA extracted from the cell pellet according to the manufacturer's protocol. DNA was evaluated for quantity and size using a Qubit fluorometer (Qubit dsDNA BR Assay Kit; Invitrogen) and Agilent Tape Station (High Sensitivity D1000 ScreenTape and reagents; Agilent Technologies Inc) and stored at−20 °C until required for molecular analysis. Details for bacterial 16S rRNA gene PCR and Illumina sequencing are provided in Supplementary Text S1. Principal coordinates analysis (PCA) was used to visualize the difference in the microbial community calculated from the computed distance matrix and to visualize the performance characteristics of the system in terms of nutrient removal. R (v4.1.3) software was used to generate correlation and PCA analysis using packages corrplot (v0.92) and factoextra (v1.0.7) respectively (Alboukadel and Mundt, 2020; Wei and Simko, 2021).
3. Results and discussion
3.1. Overall treatment performance
The experiment was carried out over 420 days and evaluated the multistage CW system on nitrogen removal using both tidal flow (Phase 1) and tidal flow with step-feeding (Phases 2 to 4) as shown in Figure 2. COD removal rate was uniform across the experiments (ANOVA, p > 0.05) consistently reaching 90%, due to the system utilizing effective aerobic conditions induced by tidal flow operation (Figure 2A). However, a lower performance (70–75% COD removal) was observed in Phase 1 during a longer period with cold temperatures when water temperature approached 10°C. Significant COD removal obtained in all experiments (Phases 1 to 4) was predominantly due to enhanced oxygenation efficacy of the tidal flow system (Zhao et al., 2004; Hu et al., 2012; Chang et al., 2014) and the intensive microbial activities these conditions promote (Dušek et al., 2008). Previously, it has been reported that in most cases, insufficient oxygen supply is the main reason for poor biological COD removal in CW systems (Korkusuz et al., 2005; Ayaz et al., 2012; Wu et al., 2015). Nitrification is restricted even more than COD reduction because oxygen is utilized for carbon oxidization before nitrification due to the faster growth rate of heterotrophic organisms compared with that of nitrifiers (Wu et al., 2011; Bassin et al., 2015; Ge et al., 2015). However, well aerated systems provide unfavorable conditions for other process such as denitrification (Vymazal and Kröpfelová, 2011).
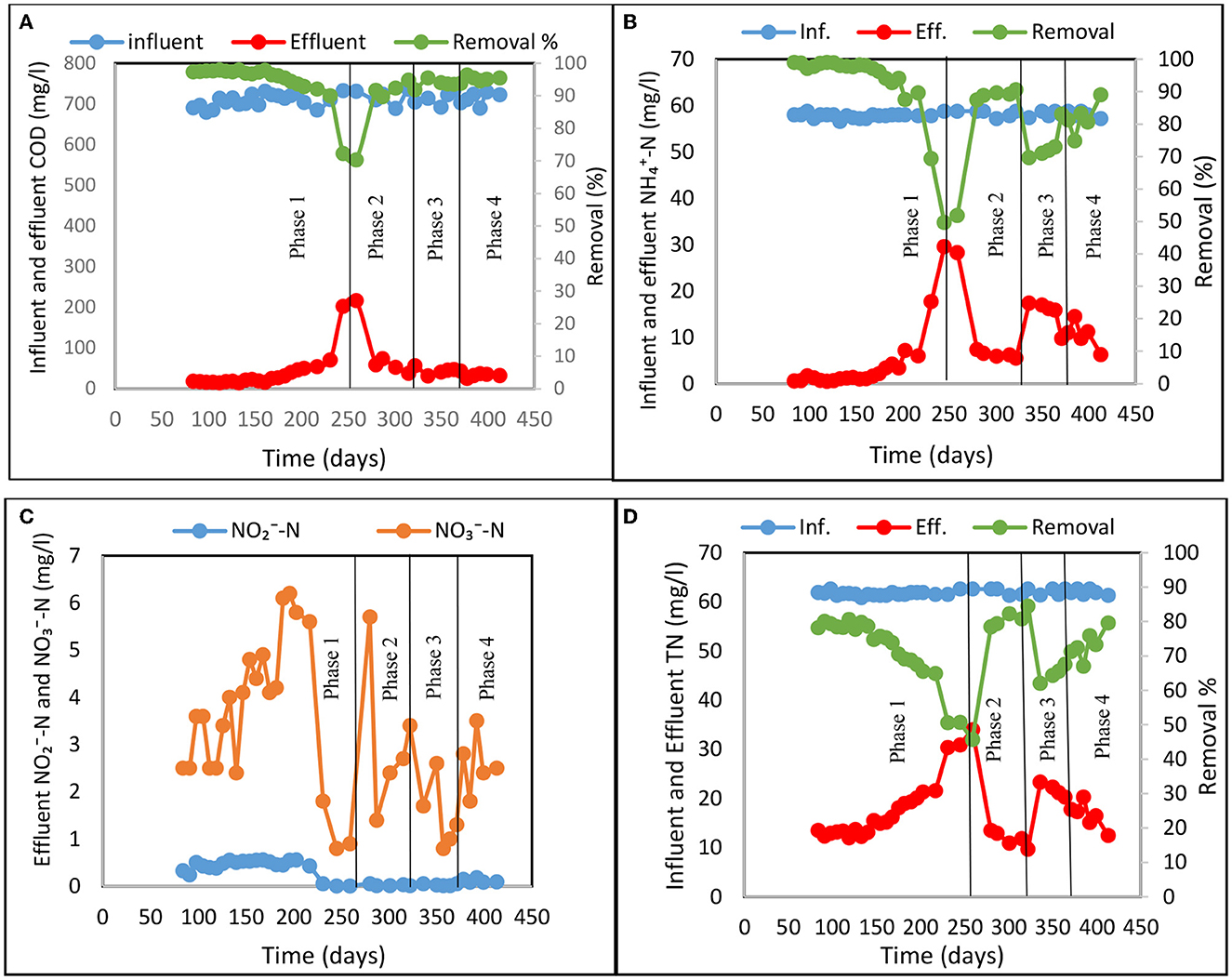
Figure 2. Treatment performance for Phases 1 to 4 in the multistage constructed wetland system: (A) Influent and effluent COD concentration and COD removal, (B) Influent and effluent -N concentration and -N removal, (C) Effluent NO2−-N and -N, and (D) Influent and effluent total nitrogen (TN) concentration and TN removal.
The average -N removal was 91 ± 8%, 89 ± 1%, 74 ± 5%, and 82 ± 5% for experimental Phases 1 to 4, respectively as shown in Figure 2B. TN removal was limited by denitrification (Figures 2C, D) and showed significant variability across the experiments (ANOVA, p < 0.05). The average removal of TN in Phase 1, where only tidal flow was employed, was 71%. After applying the step-feeding strategy alongside tidal flow in Phases 2, 3 and 4 the average TN removal was 66–81% as shown in Figure 2D. The nitrogen removal rate for Phases 2 and 4 were 77 gN/m3.d and 70 gN/m3.d respectively, higher than observed in Phase 1 (67 gN/m3.d). This is due to the hydraulic loading rate (HLR) and application of step-feeding which re-introduced external carbon source to enhance the denitrification process. Interestingly, only 63 gN/m3.d nitrogen was removed in Phase 3. Figure 2 shows that each time the new feeding ratio was introduced (Phase 2–4) the nitrogen removal was dropping and then gradually increasing with the duration of the applied step-feeding phase. This could indicate microbial community response and adaptation to new conditions when the nutrient availability was altered. The step-feeding ratio of 70:30 occurs as breakthrough point for nutrient distribution and availability in the system as it is clearly visible that the following Phase 4 although also experiencing initial drop, shows much faster rebound and consequent increase in the N removal over the studied period.
3.2. Nitrogen removal performance in individual stages
The operational condition applied in Phase 1 showed that, stages 1 and 2 were responsible for a majority (>85%) of COD removal (Supplementary Figure 1), hence depleting the remaining part of the system (stages 3–4) from the required source of carbon to deliver effective denitrification as indicated by the high nitrate concentration in the final effluent. This shows a need to improve carbon pool distribution in the system. Therefore, a step-feeding strategy was introduced in Phases 2–4 with varying feeding ratios split between stages 1 and 3. It can be seen however that the internal addition of a carbon source did not result in locally (stage 3) elevated COD concentration (Figure 3). This might suggest that the added carbon load was efficiently processed and quickly entered various microbial metabolic pathways including nitrogen cycling.
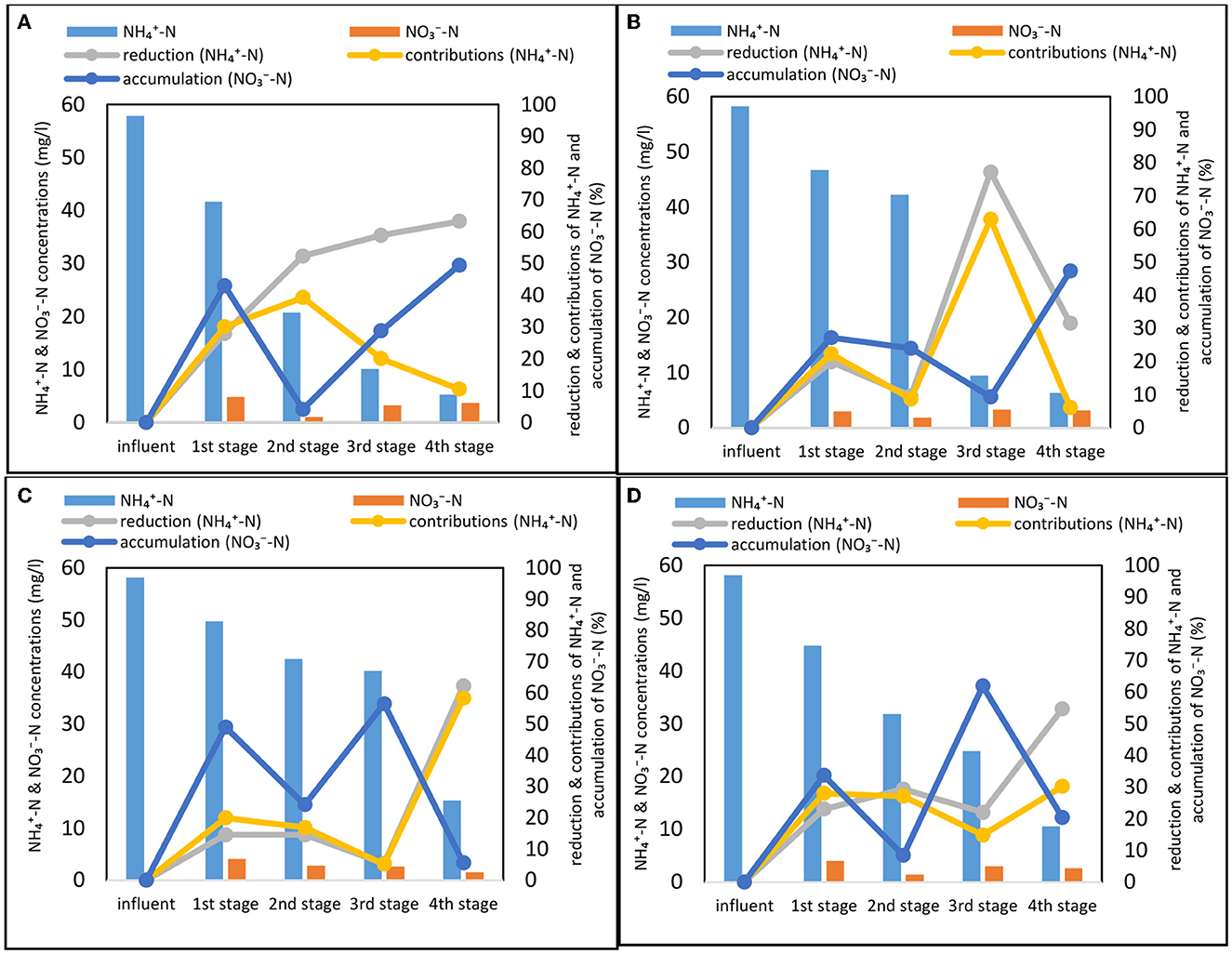
Figure 3. Nitrogen profile in individual stages of the constructed wetland for all phases (mean values of each stage) (A) Phase 1, (B) Phase 2, (C) Phase 3, and (D) Phase 4. Detailed statistical analysis (ANOVA, pairwise t-test) provided in Supplementary Tables S1–S4. Calculations on performance metrics (reduction, contributions, and accumulations) provided in Supplementary Text S3.
In Phase 1 (Figure 3A), the influent -N was reduced by 91% to 5.3 mg/l and the system showed significant (p < 0.05) increase of nitrification between stages 1–3 while plateauing and reaching similar (p > 0.05) levels at stages 3 and 4. The first two stages operated with 28 and 52% of nitrification rate respectively while reduction at stage 3 and 4 reached 59 and 63%, respectively. Nitrate accumulation varied between the stages reaching 49% at the stage 4 and only 4% at the stage 2. This shows great disproportion of denitrification potential across the treatment stages of the system and a stepwise exhaustion of carbon sources from inflow to effluent of the system.
Upon introduction of step-feeding, nitrogen dynamics were significantly (ANOVA, p < 0.05) affected by the tested step-feeding ratios displaying marked differences both between the phases but also within internal, stage-wise dynamics. The influent -N was reduced by 89, 74, and 82% with the bulk of reduction, a third of reduction and slightly more than half of the reduction occurring in the first three stages in Phase 2 (Figure 3B), Phase 3 (Figure 3C), and Phase 4 (Figure 3D), respectively. For all three phases, nitrate accumulation started from stage 1 indicating that denitrification became the limiting process for TN reduction due to the carbon deficiency. In the Phase 2 of the experiment, step-feeding introduction of carbon source activated denitrification potential at the carbon dosing point (stage 3) and showed lowest -N accumulation rate (9%) while at the same time delivering simultaneous very effective -N removal (77%) (Lai et al., 2020; Gupta et al., 2022). For step feeding ratios of 70:30 and 60:40 (Phases 3 and 4 respectively) internal introduction of carbon source showed to have initially inhibit nitrification rates (circa 15% -N reduction) and lead to increased nitrate accumulation (circa 60%) due to insufficient denitrification. This could be a sign of growing competition for carbon source between nitrogen cycling microbial consortia and the rest of bacterial community in the system as mentioned previously via TN and COD dynamics analysis. Overall, the differences in -N accumulation in the system displayed denitrification capabilities of the individual stages coupled with step-feeding. High nitrate accumulation rates (45–49%) observed for final effluent at the Phases 1 and 2 were due to low inflow -N levels (>10mg/L) and relatively high residual -N carried over from the previous stage (3 mg/L) in comparison to conditions observed at Phases 3 and 4 (25–40 mg -N /L). Nevertheless, despite different internal dynamics observed across all experiments (Phase 1–4) the differences in final effluent -N concentration were insignificant (p > 0.05).
Details for statistical analysis results for the comparison of the mean removal efficiencies between different step-feeding ratios are provided in Supplementary Text S2.
3.3. The effect of operational conditions
The invested multi-stage TFCW operated in step-feeding strategy. The wastewater inflow was distributed between stage 1 and stage 3 of the system according to the 3 step-feeding ratios of 80:20, 70:30, and 60:40. Nitrogen removal performance was significantly different between the applied step-feeding ratios (ANOVA, p < 0.01). The system operated at a minimum of 60% TN removal, reaching maximum effectiveness of over 80% when applying different step-feeding ratio thus, step-feeding ratio has been proven to be a differentiating factor in achieving effective TN removal in the study.
However, the subsequently observed effectiveness variability between studied ratios was not expected and did not show a linear relationship (ratio vs. TN removal effectiveness). The minimum TN removal performance was obtained at 70:30 ration while both 80:20 and 60:40 ratios performed comparably well, with 60:40 ratio gradually reaching 80:20 ratio performance levels by the end of the monitoring period. A similar removal performance of 80% TN removal was achieved in a four-stage TFCW study optimizing step-feeding ratio and reported 80:20 step feeding ratio to outperform 90:10 and 85:10:5 dosing ratios (Hu et al., 2012). However, test in similar TFCWs showed only above 30% nitrification, including even lower TN removal at 80:20 step-feeding ratio (Yang et al., 2011). This indicates that in addition to step-feeding ratio optimization knowledge on carbon distribution and utilization in the system is required. Carbon to nitrogen (C/N) ratio is a key parameter in nitrogen cycle (Her and Huang, 1995; Ji et al., 2015). In wastewater treatment systems, carbon to nitrogen ratio is often reported in reference to carbon source using organic carbon fraction [i.e., biochemical oxygen demand (BOD), COD, total organic carbon (TOC) or dissolved organic carbon (DOC)] or total carbon fraction (TC). This study uses a COD parameter to represent the available carbon in the studied system. A complete denitrification requires a stoichiometric COD/N ratio equal 2.86 (Fu et al., 2009). However, practical considerations show that the effective nitrogen removal via denitrification occurs when the COD/N ratio reaches above 3. Nevertheless, operational conditions, quality of wastewater and carbon source composition requires denitrification process to be carried out at COD/N ratios often reaching 10 and above (Han et al., 2015; Pelaz et al., 2018; Deng and Shi, 2020). Meanwhile, anammox process becomes dominant and capable of delivering over 90% N removal when the COD/N ratio equals to 1 and lower (Wang et al., 2019; Sarvajith et al., 2020). In conventional CW designs (Vertical flow (VF)-, Horizontal flow (HF)-, Free water surface (FWS)-CW) operating with municipal wastewater, COD/N ratio ranging between 5–10 can deliver up to 50% nitrogen removal (Li et al., 2020; Rampuria et al., 2020; Zhu et al., 2021). High performing CWs specifically designed for N removal operate on higher COD/N ratios due to optimization of carbon dosage. Introduction of tidal-flow or spray aeration in CW can help to deliver up to 80% of nitrogen removal however a COD/N >6 is required (Zhi and Ji, 2014; Wang et al., 2020).
The investigated system showed a strong correlation (p < 0.05) between COD/N ratio and TN removal observed the final effluent at the stage 4 (Supplementary Figure S2). The highest performing Phase 2 (80:20 ratio) delivered >80% TN removal in the effluent, maintaining an average 4.9 COD/N ratio while Phase 3 and Phase 4 both showed C/N ratios varying between 2–3 indicating a potential shortage of carbon source in the system. Interestingly, the highest performing Phase 2 had least favorable thermal conditions, with water temperatures on average 6°C lower than Phase 4 (11.5°C and 18.1°C respectively). PCA analysis (Figure 4) reveals overlapping clusters between Phase 3 and 4 while Phase 2 performance data was grouped separately further indicating supreme performance of Phase 2. High performance effectiveness of 80:20 ratio is even more visible when considering variability of thermal conditions the system was exposed to Supplementary Figure S2 and which would affects kinetics of nitrogen removal (Pang et al., 2015; Myszograj and Bydałek, 2016). Nevertheless, the strong inverse correlation between water temperatures in the system (temp_out, Supplementary Figure S2) and COD/N ratio indicated that the inhibitive effect of low temperature can be overcome with higher carbon availability and conversely at higher temperatures, excessive carbon surplus is not required to sustain effective N removal in the investigated system.
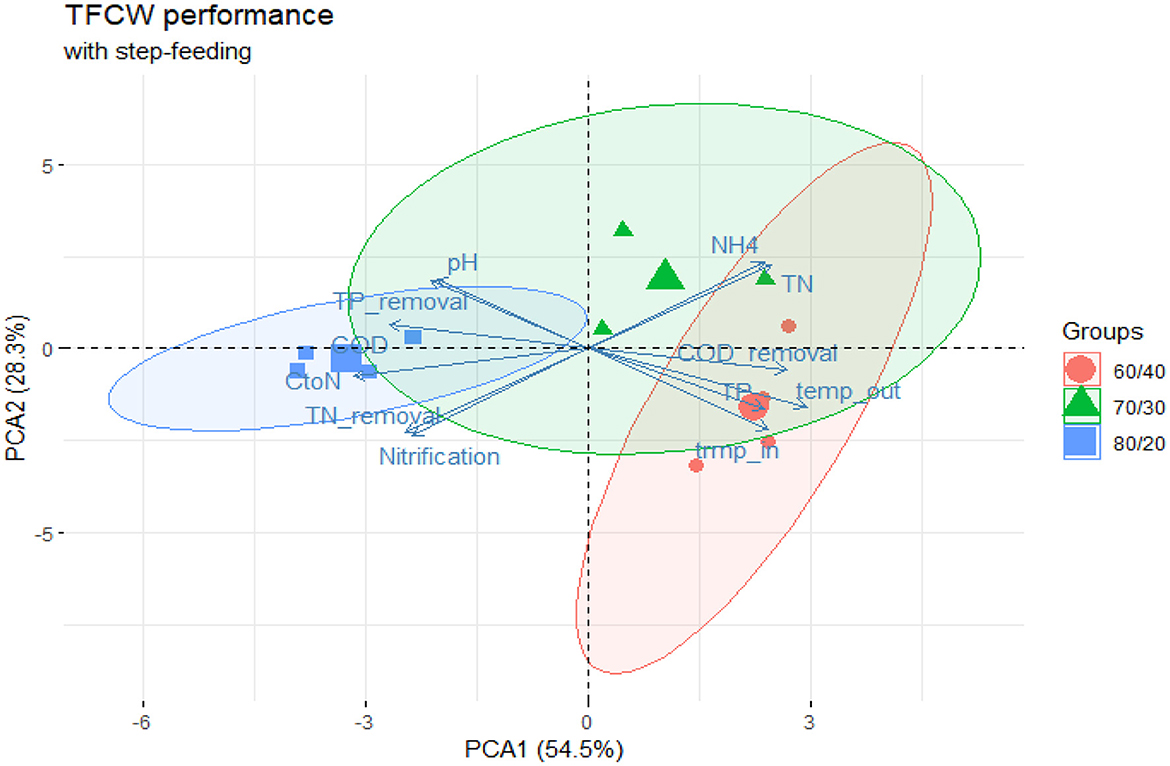
Figure 4. PCA plot capturing the performance of tidal flow CW operating with step-feeding strategy. Performance data points formed 3 distinctive groups separated primarily along the first coordinate axis with COD/N ratio and water temperature contributing most (38%) to the first component's variance. TN, COD, TP and are concentration values in the final effluent (stage 4) of the system; CtoN—COD to nitrogen ratio; temp_in/temp_out—water temperature in the inflow and outflow of the system.
3.4. Microbial community of the multistage CW system after 420 days (operational Phase 4)
A combined total of 1,079,014 16S rRNA gene sequence reads were obtained from the extracted DNA from the upper and lower positions of the gravel substrate from the four stages of the CW system after 420 days (Phase 4; 1L, 1U, 2L, 2U, 3L, 3U, 4L, and 4U). Read numbers ranged from 104,118 (3U) to 191,610 (1U) sequences per sample with an average read count of 134,877 reads. Rarefaction curves (Supplementary Figure S3) and Good's coverage statistics (Supplementary Table S5) indicate that 16S rRNA gene libraries for each gravel sample were sampled sufficiently to capture the majority of the bacterial diversity. Interestingly, both diversity (Shannon and Simpson diversity) and species richness (SChao1) indices (Hugerth and Andersson, 2017) increased in the CW system from stage 1 to stage 4 (Supplementary Table S5, Supplementary Figure S3), which demonstrates that bacterial diversity increased at each stage throughout the system. This agrees with other multi-stage CW systems that also showed an increase in bacterial diversity through the system from inlet to outlet (Babatunde et al., 2016; Rajan et al., 2019) and is corroborated by PCA UniFrac analysis (Supplementary Figure S5).
Bacterial 16S rRNA gene sequences were assigned taxonomy to identify the different bacterial communities at each stage and were classified from phylum to genus level. The relative abundance of a given bacterial group was set as the number of sequences affiliated with that group divided by the total number of reads per sample (Figures 5, 6; Supplementary Table S5, Supplementary Figure S4). In the CW system, a total of 14 bacterial phyla representing 62 assigned genera were identified at greater than 0.1% of the community. However, in all samples a large fraction of the sequences could not be assigned at the genus level (50 to 61.2%; Supplementary Figure S4a), and this presumably represents a large proportion of novel and unknown genera.
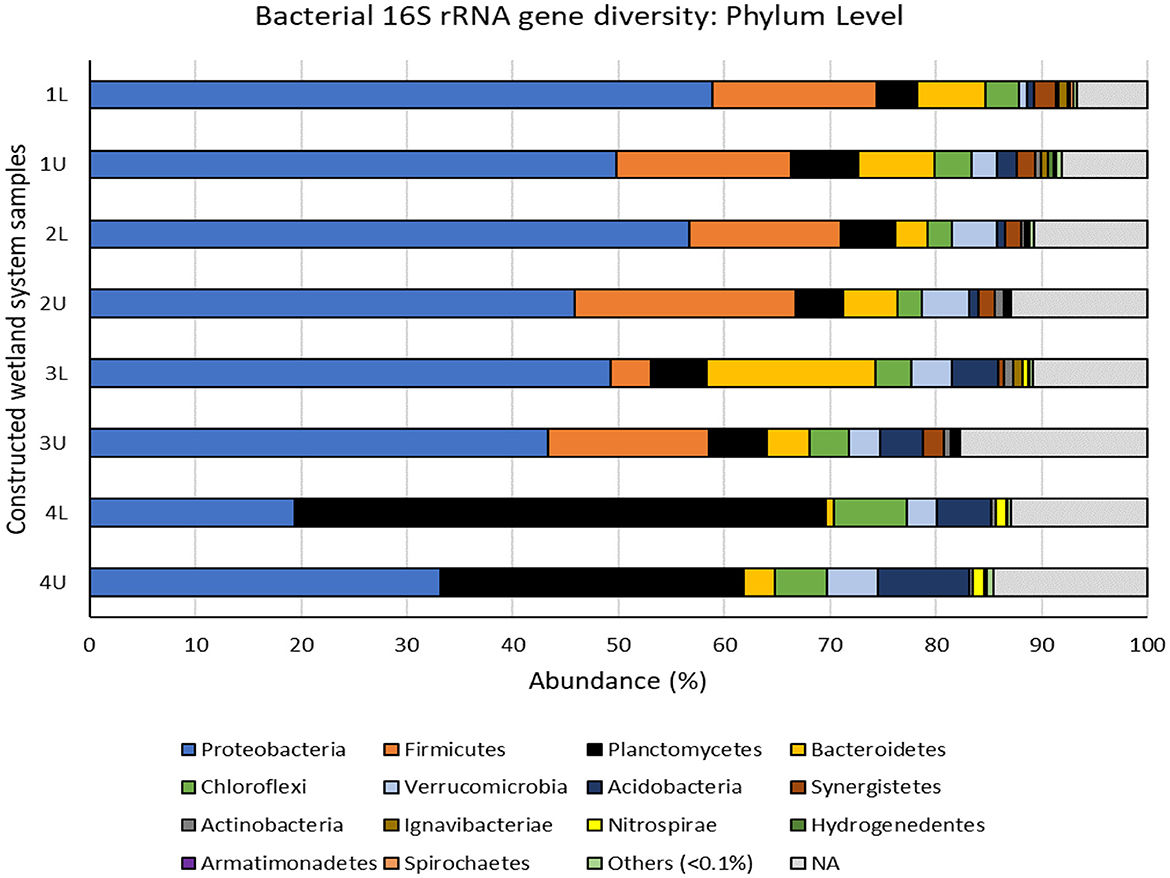
Figure 5. Bacterial 16S rRNA gene diversity in the multistage constructed wetland system at the end of Phase 4 assigned at the phylum level. 1L, stage 1 lower position; 1U, stage 1 upper position; 2L, stage 2 lower position; 2U, stage 2 upper position; 3L, stage 3 lower position; 3U, stage 3 upper position; 4L, stage 4 lower position; 4U, stage 4 upper position. Others (<0.1%) represents bacterial phyla with less than 0.1% abundance in each sample (Gemmatimonadetes, Cloacimonetes, Chlamydiae, Latescibacteria, Lentisphaerae, Parcubacteria, Elusimicrobia, Deinococcus-Thermus and candidate phyla WPS-1, WPS-2, SR1, and BRC1). NA represents bacterial OTUs that were unassigned at the phylum level.
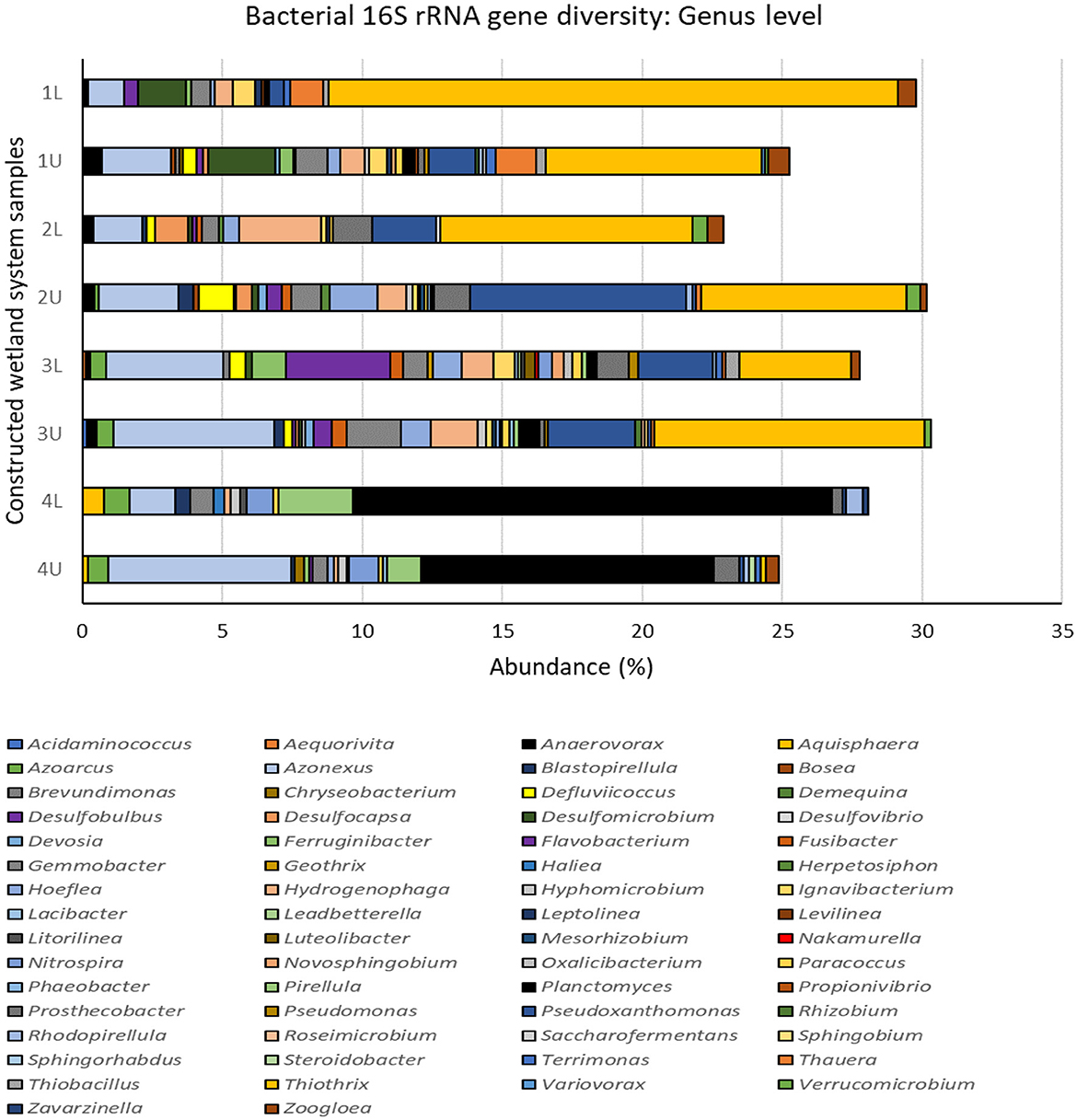
Figure 6. Bacterial 16S rRNA gene diversity in the multistage constructed wetland system at the end of Phase 4 assigned at the genus level. 1L, stage 1 lower position; 1U, stage 1 upper position; 2L, stage 2 lower position; 2U, stage 2 upper position; 3L, stage 3 lower position; 3U, stage 3 upper position; 4L, stage 4 lower position; 4U, stage 4 upper position. Data excludes all unassigned OTUs, see Supplementary Figure 4.
Overall, the CW system was dominated by members of the phyla: Proteobacteria (44.5%), Firmicutes (10.9%), Planctomycetes (13.6), Bacteroidetes (5.7%), Chloroflexi (3.8%), Verrucomicrobia (3.3%), and Acidobacteria (3.3%), and lesser proportions of Synergistetes (1.2%), Actinobacteria (0.53%), Ignavibacterae (0.36%), Nitrospirae (0.35%), Hydrogenedentes (0.12%), Armatimonadetes (0.11%), and Spirochaetes (0.10%), although there were some clear differences between phyla at different stages (Figure 5). The five most commonly found phyla in our study have been reported previously in CW systems and are thought to be key phyla in the successful operation of CW systems (Verduzo Garibay et al., 2021). The most abundant phylum Proteobacteria showed clear differences at the class level, with higher numbers of Gammaproteobacteria being found in stages 1 and 2 and Betaproteobacteria becoming more prevalent at stages 3 and 4 (Supplementary Figure S4b). Alphaproteobacteria and Deltaproteobacteria were found consistently throughout, while unclassified Proteobacteria were abundant in stage 1 but rapidly declined after stage 2.
Changes in bacterial diversity and community structure with stage and position may be linked to the different processes and rates of N removal occurring at each stage, coupled with the introduction of fresh carbon sources at stage 3. This is evident from the dominant assigned bacterial genera (Figure 6) and the top 20 16S rRNA gene operational taxonomic units (OTUs) (Supplementary Figure S6) identified as proxy for bacterial species. The dominant bacterial genera observed (Figure 6) in the whole CW system (representing >1% abundance) were Thiothrix, Planctomyces, Azonexus, Pseudoxanthomonas, Hydrogenophaga, and Gemmobacter, and all varied in abundance depending on CW stage. For example, the top three genera, Thiothrix ranged from 3.9–20.3% in stages 1 to 3 and but were <0.2% at stage 4. Conversely, Planctomyces were <0.7% in stages 1 to 3 but ranged from 10.4–17.1% at stage 4, while Azonexus steadily increased from 1.3–2.4% at stage 1 to 1.6–6.5% at stage 4. All dominant genera were represented in the top 20 OTUs, although the most common OTU (or bacterial species) was OTU0009, which was unassigned at the genus level but could be assigned at the family level and belonged to members of the Veillonellaceae.
Operational taxonomic unit OTU0009 was particularly prevalent at stages 1 to 3 and nearly absent at stage 4. The increased abundance of OTU008 in stage 3 and the upper portion of stage 4 correlates with the addition of the carbon source supplement as denitrification in CW systems by these organisms is known to be associated with high TOC (Wu et al., 2016). Pseudoxanthomonas are also known heterotrophic denitrifiers and OTU0045 was found throughout the system but particularly within stage 2. Other abundant denitrifiers found throughout included Gemmobacter (OTU0014) which can denitrify mixotrophically (Du et al., 2020) and Hydrogenophaga (OTU0056) which can utilize hydrogen (Willems et al., 1989).
Stage 4 was dominated by members of the Planctomycetes (Figure 5), including the genera Planctomyces and Pirellula (Figure 6), and other uncultured genera (Supplementary Figure S6). Many Planctomycetes conduct anammox metabolism, a process in which ammonia is oxidized by nitrite to nitrogen gas. Planctomyces OTU0059, and Planctomycetaceae OTUs (OTU0062, OTU0065, and OTU0080; Supplementary Figure S6) were well represented in the top 20 OTUs in high numbers at stage 4. Presumably very low oxygen (or anoxic) conditions and low concentrations of organic matter in the fourth stage allowed for these bacteria to proliferate and outcompete denitrifying Proteobacteria (Jin et al., 2012; Mosley et al., 2022).
However, further investigations are necessary to confirm the taxonomic identity of the putative anammox bacteria through further sequencing (16S rRNA and hydrazine synthase genes; Mosley et al., 2022) or anammox-specific probes (Tal et al., 2006).
3.5. Nitrogen cycling bacteria
Abundance of denitrifying Proteobacteria (e.g., Thiothrix, Thauera, Pseudoxanthomonas and Hydrogenophaga) in the CW system in stages 1 to 3 suggests that this is the major N removal process with both heterotrophic and autotrophic denitrification occurring. Evidence of autotrophic ammonia-oxidizing bacteria (Nitrosomonas and Nitrosospira) and autotrophic nitrite-oxidizing bacteria (Nitrospira and Nitrolancea) occurred throughout the system, although at very low abundance (<1.0%; Supplementary Figure S7) demonstrates that ammonia was continually oxidized to nitrate. The high abundance of Planctomycetes and the potential for anammox bacteria suggests that the anammox process was an alternatively occurring pathway for nitrogen removal, especially at stage 4 of Phase 4 when conditions were suitable. Clearly, nitrogen removal in the CW system was accomplished via diverse pathways, including autotrophic nitrification, heterotrophic denitrification, autotrophic denitrification, and possibly anammox. Similar collaborative microbial pathways for N removal have been found routinely in constructed wetlands (Wei et al., 2021; Zhang et al., 2021) and it is the abundance, consortia and distribution of these organisms that is key to the performance of a CW system (Zhang et al., 2021).
4. Conclusion
The multistage vertical flow constructed wetland applied a combination of tidal flow and step-feeding strategies with 2 h saturation (wet) and 6 h unsaturation (dry), giving a total of 8 h per cycle. The results indicate that the proposed system was able to deal with high concentration levels of organics (expressed as COD) and nitrogen and efficiently remove them. The overall removal efficiency during the experimental period for COD, -N and TN was up to 95.4, 90.9, and 81.1%, respectively. The improvement of removal efficiency was attributed to the tidal flow strategy as well as the prolonged unsaturated time that enhanced the oxygen transfer to the system. It was possible to use the step-feeding strategy to treat the synthetic domestic wastewater with high influent concentrations of organic matter and nitrogen to enhance the TN removal performance efficiency. Consequently, sufficient bed resting time (6 h) and the addition of a carbon source at the third stage of the system were key factors to preserve the efficient nitrification process and support the denitrification process. Statistical analysis showed that the step-feeding ratio has a significant impact on organic matter and nitrogen removal and identified the 80:20 step feeding ratio to provide best overall performance for COD and N removal. The bacterial diversity increased at each stage throughout the system and was composed of bacterial phyla consistently found in CW systems (e.g., Proteobacteria, Firmicutes, Planctomycetes, Bacteroidetes, Chloroflexi, Verrucomicrobia, and Acidobacteria), and dominant genera were representative of nitrogen cycling bacteria undertaking N removal via diverse metabolic pathways, including autotrophic nitrification, heterotrophic denitrification, autotrophic denitrification, and possibly anammox.
Data availability statement
All relevant data is contained within the article: The original contributions presented in the study are included in the article/Supplementary material, further inquiries can be directed to the corresponding author/s.
Author contributions
MK: conceptualization, methodology, investigation, resources, writing—original draft, and funding acquisition. FB: validation, formal analysis, writing—review and editing, visualization, and funding acquisition. AB: supervision. AA-M: data curation. JW: writing—review and editing and visualization. GW: validation, formal analysis, and writing—original draft, visualization. All authors contributed to the article and approved the submitted version.
Funding
FB was supported by a Natural Environment Research Council (NERC) PhD studentship at the GW4 Center for Doctoral Training in Freshwater Biosciences and Sustainability (GW4 FRESH CDT, Grant No. NE/RO11524/1).
Acknowledgments
The authors would like to thank Kuwait Institute for Scientific Research (KISR) for their financial support of this study. Also, special thanks to the technical staff at the Cardiff University School of Engineering and the School of Biosciences Genomics Research Hub.
Conflict of interest
MK reports financial support was provided by Kuwait Institute for Scientific Research. FB reports financial support was provided by UK Research and Innovation.
The remaining authors declare that the research was conducted in the absence of any commercial or financial relationships that could be construed as a potential conflict of interest.
Publisher's note
All claims expressed in this article are solely those of the authors and do not necessarily represent those of their affiliated organizations, or those of the publisher, the editors and the reviewers. Any product that may be evaluated in this article, or claim that may be made by its manufacturer, is not guaranteed or endorsed by the publisher.
Supplementary material
The Supplementary Material for this article can be found online at: https://www.frontiersin.org/articles/10.3389/frwa.2023.1128901/full#supplementary-material
Abbreviations
-N, Ammonium-nitrogen; anammox, Anaerobic ammonium oxidation; BOD, Biochemical oxygen demand; COD/N, Carbon/nitrogen; COD, Chemical oxygen demand; CWs, Constructed wetlands; DOC, Dissolved organic carbon; FWSCW, Free water surface constructed wetlands; HFCW, Horizontal flow constructed wetlands; HLR, Hydraulic loading rate; L, Lower; N, Nitrate-nitrogen; NO2−-N, Nitrite-nitrogen; N, Nitrogen; OUT, Operational taxonomic units; PCA, Principal coordinates analysis; SND, Simultaneous nitrification and denitrification; TFCWs, Tidal flow CWs; TC, Total carbon; TN, Total nitrogen; TOC, Total organic carbon; U, Upper; VFCWs, Vertical flow CWs.
References
Alboukadel, K., and Mundt, F. (2020). Factoextra: extract and visualize the results of multivariate data analyses. R Package Version 1, 337–54. Available online at: https://cran.r-project.org/web/packages/factoextra/index.html
Ávila, C., Pelissari, C., Sezerino, P. H., Sgroi, M., Roccaro, P., and García, J. (2017). Enhancement of total nitrogen removal through effluent recirculation and fate of PPCPs in a hybrid constructed wetland system treating urban wastewater. Sci. Total Environ. 584, 414–425. doi: 10.1016/j.scitotenv.2017.01.024
Ayaz, S. Ç., Aktaş, Ö., Findik, N., Akça, L., and Kinaci, C. (2012). Effect of recirculation on nitrogen removal in a hybrid constructed wetland system. Ecol. Eng. 40, 1–5. doi: 10.1016/j.ecoleng.2011.12.028
Babatunde, A., Miranda-CasoLuengo, R., Imtiaz, M., Zhao, Y., and Meijer, W. G. (2016). Performance assessment and microbial diversity of two pilot scale multi-stage sub-surface flow constructed wetland systems. J. Environ. Sci. 46, 38–46. doi: 10.1016/j.jes.2015.02.018
Barbera, A. C., Cirelli, G. L., Cavallaro, V., Di Silvestro, I., Pacifici, P., Castiglione, V., et al. (2009). Growth and biomass production of different plant species in two different constructed wetland systems in Sicily. Desalination 246, 129–136. doi: 10.1016/j.desal.2008.03.046
Bassin, J., Abbas, B., Vilela, C., Kleerebezem, R., Muyzer, G., Rosado, A., et al. (2015). Tracking the dynamics of heterotrophs and nitrifiers in moving-bed biofilm reactors operated at different COD/N ratios. Biores. Technol. 192, 131–141. doi: 10.1016/j.biortech.2015.05.051
Brix, H. (1994). Functions of macrophytes in constructed wetlands. Water Sci. Technol. 29, 71–78. doi: 10.2166/wst.1994.0160
Chang, Y., Wu, S., Zhang, T., Mazur, R., Pang, C., and Dong, R. (2014). Dynamics of nitrogen transformation depending on different operational strategies in laboratory-scale tidal flow constructed wetlands. Sci. Total Environ. 487, 49–56. doi: 10.1016/j.scitotenv.2014.03.114
Chen, H. (2011). Surface-flow constructed treatment wetlands for pollutant removal: applications and perspectives. Wetlands 31, 805–814. doi: 10.1007/s13157-011-0186-3
Deng, G., and Shi, X. (2020). The effects of carbon source and COD/N ratio on simultaneous denitrification and methanogenesis in an upflow anaerobic sludge blanket reactor. Renew. Energy 157, 867–873. doi: 10.1016/j.renene.2020.05.105
Du, S., Ya, T., Zhang, M., Zhu, M., Li, N., Liu, S., et al. (2020). Distinct microbial communities and their networks in an anammox coupled with sulfur autotrophic/mixotrophic denitrification system. Environ. Pollut. 262, 114190. doi: 10.1016/j.envpol.2020.114190
Dumax, N., and Rozan, A. (2021). Valuation of the environmental benefits induced by a constructed wetland. Wetlands Ecol. Manage. 29, 809–822. doi: 10.1007/s11273-021-09811-x
Dušek, J., Picek, T., and CíŽková, H. (2008). Redox potential dynamics in a horizontal subsurface flow constructed wetland for wastewater treatment: Diel, seasonal and spatial fluctuations. Ecol. Eng. 34, 223–232. doi: 10.1016/j.ecoleng.2008.08.008
Fillos, J., Diyamandoglu, V., Carrio, L. A., and Robinson, L. (1996). Full-scale evaluation of biological nitrogen removal in the step-feed activated sludge process. Water Environ. Res. 68, 132–142. doi: 10.2175/106143096X127325
Fu, Z., Yang, F., Zhou, F., and Xue, Y. (2009). Control of COD/N ratio for nutrient removal in a modified membrane bioreactor (MBR) treating high strength wastewater. Bioresour. Technol. 100, 136–141. doi: 10.1016/j.biortech.2008.06.006
Garfí, M., Flores, L., and Ferrer, I. (2017). Life cycle assessment of wastewater treatment systems for small communities: Activated sludge, constructed wetlands and high rate algal ponds. J. Cleaner Product. 161, 211–219. doi: 10.1016/j.jclepro.2017.05.116
Ge, S., Wang, S., Yang, X., Qiu, S., Li, B., and Peng, Y. (2015). Detection of nitrifiers and evaluation of partial nitrification for wastewater treatment: a review. Chemosphere 140, 85–98. doi: 10.1016/j.chemosphere.2015.02.004
Gupta, R. K., Poddar, B. J., Nakhate, S. P., Chavan, A. R., Singh, A. K., Purohit, H. J., et al. (2022). Role of heterotrophic nitrifiers and aerobic denitrifiers in simultaneous nitrification and denitrification process: a nonconventional nitrogen removal pathway in wastewater treatment. Lett. Appl. Microbiol. 74, 159–184. doi: 10.1111/lam.13553
Han, X., Wang, Z., Ma, J., Zhu, C., Li, Y., and Wu, Z. (2015). Membrane bioreactors fed with different COD/N ratio wastewater: impacts on microbial community, microbial products, and membrane fouling. Environ. Sci. Pollut. Res. 22, 11436–11445. doi: 10.1007/s11356-015-4376-z
Her, J.-J., and Huang, J.-S. (1995). Influences of carbon source and C/N ratio on nitrate/nitrite denitrification and carbon breakthrough. Biores. Technol. 54, 45–51. doi: 10.1016/0960-8524(95)00113-1
Hu, Y., Zhao, Y., and Rymszewicz, A. (2014). Robust biological nitrogen removal by creating multiple tides in a single bed tidal flow constructed wetland. Sci. Total Environ. 470, 1197–1204. doi: 10.1016/j.scitotenv.2013.10.100
Hu, Y. S., Zhao, Y. Q., Zhao, X. H., and Kumar, J. L. (2012). Comprehensive analysis of step-feeding strategy to enhance biological nitrogen removal in alum sludge-based tidal flow constructed wetlands. Bioresour. Technol. 111, 27–35. doi: 10.1016/j.biortech.2012.01.165
Hugerth, L. W., and Andersson, A. F. (2017). Analysing microbial community composition through amplicon sequencing: from sampling to hypothesis testing. Front. Microbiol. 8, 1561. doi: 10.3389/fmicb.2017.01561
Ilyas, H., and Masih, I. (2017). The performance of the intensified constructed wetlands for organic matter and nitrogen removal: A review. J. Environ. Manage. 198, 372–383. doi: 10.1016/j.jenvman.2017.04.098
Jehawi, O. H., Abdullah, S. R. S., Kurniawan, S. B., Ismail, N. I., Idris, M., Al Sbani, N. H., et al. (2020). Performance of pilot Hybrid Reed Bed constructed wetland with aeration system on nutrient removal for domestic wastewater treatment. Environ. Technol. Innov. 19, 100891. doi: 10.1016/j.eti.2020.100891
Ji, B., Yang, K., Zhu, L., Jiang, Y., Wang, H., Zhou, J., et al. (2015). Aerobic denitrification: a review of important advances of the last 30 years. Biotechnol. Biopr. Eng. 20, 643–651. doi: 10.1007/s12257-015-0009-0
Ji, B., Zhao, Y., Vymazal, J., Qiao, S., Wei, T., Li, J., et al. (2020). Can subsurface flow constructed wetlands be applied in cold climate regions? A review of the current knowledge. Ecol. Eng. 157, 105992. doi: 10.1016/j.ecoleng.2020.105992
Jin, R.-C., Yang, G.-F., Yu, J.-J., and Zheng, P. (2012). The inhibition of the Anammox process: A review. Chem. Eng. J. 197, 67–79. doi: 10.1016/j.cej.2012.05.014
Kadlec, R., and Knight, R. (1996). Treatment Wetlands. Boca Raton, Florida, USA: Chemical Rubber Company Press. Boca Raton, FL: Lewis Publishers.
Kadlec, R. H., and Wallace, S. (2008). Treatment Wetlands. 2nd ed. Florida, USA: CRC press. doi: 10.1201/9781420012514
Korkusuz, E. A., Beklioglu, M., and Demirer, G. N. (2005). Comparison of the treatment performances of blast furnace slag-based and gravel-based vertical flow wetlands operated identically for domestic wastewater treatment in Turkey. Ecol. Eng. 24, 185–198. doi: 10.1016/j.ecoleng.2004.10.002
Kulshreshtha, N. M., Verma, V., Soti, A., Brighu, U., and Gupta, A. B. (2022). Exploring the contribution of plant species in the performance of constructed wetlands for domestic wastewater treatment. Biores. Technol. Reports, 18, 101038. doi: 10.1016/j.biteb.2022.101038
Lai, C., Guo, Y., Cai, Q., and Yang, P. (2020). Enhanced nitrogen removal by simultaneous nitrification-denitrification and further denitrification (SND-DN) in a moving bed and constructed wetland (MBCW) integrated bioreactor. Chemosphere 261, 127744. doi: 10.1016/j.chemosphere.2020.127744
Lee, C. G., Fletcher, T. D., and Sun, G. (2009). Nitrogen removal in constructed wetland systems. Eng. Life Sci. 9, 11–22. doi: 10.1002/elsc.200800049
Lekshmi, B., Sharma, S., Sutar, R. S., Parikh, Y. J., Ranade, D. R., and Asolekar, S. R. (2020). “Circular economy approach to women empowerment through reusing treated rural wastewater using constructed wetlands,” in Waste Management as Economic Industry Towards Circular Economy (Springer) 1–10. doi: 10.1007/978-981-15-1620-7_1
Li, L., He, C., Ji, G., Zhi, W., and Sheng, L. (2015). Nitrogen removal pathways in a tidal flow constructed wetland under flooded time constraints. Ecol. Eng. 81, 266–271. doi: 10.1016/j.ecoleng.2015.04.073
Li, Y., Zhang, H., Zhu, L., Chen, H., Du, G., Gao, X., et al. (2020). Evaluation of the long-term performance in a large-scale integrated surface flow constructed wetland–pond system: A case study. Biores. Technol. 309, 123310. doi: 10.1016/j.biortech.2020.123310
Li, Y., Zhu, S., Zhang, Y., Lv, M., Kinhoun, J. J. R., Qian, T., et al. (2021). Constructed wetland treatment of source separated washing wastewater in rural areas of southern China. Separ. Purific. Technol. 272, 118725. doi: 10.1016/j.seppur.2021.118725
Lu, J., Guo, Z., Kang, Y., Fan, J., and Zhang, J. (2020). Recent advances in the enhanced nitrogen removal by oxygen-increasing technology in constructed wetlands. Ecotoxicol. Environ. Safety 205, 111330. doi: 10.1016/j.ecoenv.2020.111330
Masi, F., Rizzo, A., and Regelsberger, M. (2018). The role of constructed wetlands in a new circular economy, resource oriented, and ecosystem services paradigm. J. Environ. Manag. 216, 275–284. doi: 10.1016/j.jenvman.2017.11.086
Miyaji, Y., Iwasaki, M., and Sekigawa, Y. (1980). “Biological nitrogen removal by step-feed process,” in Water Pollution Research and Development (Pergamon) 193–202. doi: 10.1016/B978-1-4832-8438-5.50018-2
Moreira, F. D., and Dias, E. H. O. (2020). Constructed wetlands applied in rural sanitation: A review. Environ. Res. 190, 110016. doi: 10.1016/j.envres.2020.110016
Mosley, O. E., Gios, E., Weaver, L., Close, M., Daughney, C., van der Raaij, R., et al. (2022). Metabolic diversity and aero-tolerance in anammox bacteria from geochemically distinct aquifers. Msystems. 7, e01255–21. doi: 10.1128/msystems.01255-21
Myszograj, S., and Bydałek, F. A. (2016). Temperature impact of nitrogen transformation in technological system: vertical flow constructed wetland and polishing pond. Civil Environ. Eng. Rep. 23, 125–136. doi: 10.1515/ceer-2016-0057
Negi, D., Verma, S., Singh, S., Daverey, A., and Lin, J.-G. (2022). Nitrogen Removal via Anammox process in Constructed Wetland-A comprehensive review. Chem. Eng. J. 437, 135434. doi: 10.1016/j.cej.2022.135434
Pang, Y., Zhang, Y., Yan, X., and Ji, G. (2015). Cold temperature effects on long-term nitrogen transformation pathway in a tidal flow constructed wetland. Environ. Sci. Technol. 49, 13550–13557. doi: 10.1021/acs.est.5b04002
Pelaz, L., Gómez, A., Letona, A., Garralón, G., and Fdz-Polanco, M. (2018). Nitrogen removal in domestic wastewater. Effect of nitrate recycling and COD/N ratio. Chemosphere 212, 8–14. doi: 10.1016/j.chemosphere.2018.08.052
Pelissari, C., Sezerino, P. H., Decezaro, S. T., Wolff, D. B., Bento, A. P., de Carvalho Junior, O., et al. (2014). Nitrogen transformation in horizontal and vertical flow constructed wetlands applied for dairy cattle wastewater treatment in southern Brazil. Ecol. Eng. 73, 307–310. doi: 10.1016/j.ecoleng.2014.09.085
Puig, S., Vives, M., Corominas, L., Balaguer, M., and Colprim, J. (2004). Wastewater nitrogen removal in SBRs, applying a step-feed strategy: from lab-scale to pilot-plant operation. Water Sci. Technol. 50, 89–96. doi: 10.2166/wst.2004.0617
Rajan, R. J., Sudarsan, J., and Nithiyanantham, S. (2019). Microbial population dynamics in constructed wetlands: Review of recent advancements for wastewater treatment. Environ. Eng. Res. 24, 181–190. doi: 10.4491/eer.2018.127
Rampuria, A., Gupta, A. B., and Brighu, U. (2020). Nitrogen transformation processes and mass balance in deep constructed wetlands treating sewage, exploring the anammox contribution. Biores. Technol. 314, 123737. doi: 10.1016/j.biortech.2020.123737
Ruan, W., Cai, H., Xu, X., Man, Y., Wang, R., Tai, Y., et al. (2021). Efficiency and plant indication of nitrogen and phosphorus removal in constructed wetlands: A field-scale study in a frost-free area. Sci. Total Environ. 799, 149301. doi: 10.1016/j.scitotenv.2021.149301
Saeed, T., Miah, M. J., Khan, T., and Ove, A. (2020). Pollutant removal employing tidal flow constructed wetlands: media and feeding strategies. Chem. Eng. J. 382, 122874. doi: 10.1016/j.cej.2019.122874
Sarvajith, M., Reddy, G. K. K., and Nancharaiah, Y. V. (2020). Aerobic granular sludge for high-strength ammonium wastewater treatment: Effect of COD/N ratios, long-term stability and nitrogen removal pathways. Biores. Technol. 306, 123150. doi: 10.1016/j.biortech.2020.123150
Stefanakis, A., Akratos, C. S., and Tsihrintzis, V. A. (2014). Vertical Flow Constructed Wetlands: Eco-Engineering Systems for Wastewater and Sludge Treatment. Oxford, UK: Newnes. doi: 10.1016/B978-0-12-404612-2.00008-8
Sun, G., Zhao, Y., and Allen, S. (2005). Enhanced removal of organic matter and ammoniacal-nitrogen in a column experiment of tidal flow constructed wetland system. J. Biotechnol. 115, 189–197. doi: 10.1016/j.jbiotec.2004.08.009
Tal, Y., Watts, J. E., and Schreier, H. J. (2006). Anaerobic ammonium-oxidizing (anammox) bacteria and associated activity in fixed-film biofilters of a marine recirculating aquaculture system. Appl. Environ. Microbiol. 72, 2896–2904. doi: 10.1128/AEM.72.4.2896-2904.2006
Tan, X., Yang, Y.-L., Li, X., Zhou, Z.-W., Liu, C.-J., Liu, Y.-W., et al. (2020). Intensified nitrogen removal by heterotrophic nitrification aerobic denitrification bacteria in two pilot-scale tidal flow constructed wetlands: influence of influent C/N ratios and tidal strategies. Bioresour. Technol. 302, 122803. doi: 10.1016/j.biortech.2020.122803
Tang, C.-C., Kuo, J., and Weiss, J. S. (2007). Maximum nitrogen removal in the step-feed activated sludge process. Water Environ. Res. 79, 367–374. doi: 10.2175/106143006X111808
Verduzo Garibay, M., Fernández del Castillo, A., de Anda, J., Senés-Guerrero, C., and Gradilla-Hernández, M. (2021). Structure and activity of microbial communities in response to environmental, operational, and design factors in constructed wetlands. Int. J. Environ. Sci. Technol. 1–26. doi: 10.1007/s13762-021-03719-y
Vymazal, J. (2007). Removal of nutrients in various types of constructed wetlands. Sci. Total Environ. 380, 48–65. doi: 10.1016/j.scitotenv.2006.09.014
Vymazal, J. (2010). Constructed wetlands for wastewater treatment. Water 2, 530–549. doi: 10.3390/w2030530
Vymazal, J. (2013). The use of hybrid constructed wetlands for wastewater treatment with special attention to nitrogen removal: a review of a recent development. Water Res. 47, 4795–4811. doi: 10.1016/j.watres.2013.05.029
Vymazal, J., and Kröpfelová, L. (2009). Removal of nitrogen in constructed wetlands with horizontal sub-sureface flow: a review. Wetlands 29, 1114–1124. doi: 10.1672/08-216.1
Vymazal, J., and Kröpfelová, L. (2011). A three-stage experimental constructed wetland for treatment of domestic sewage: first 2 years of operation. Ecol. Eng. 37, 90–98. doi: 10.1016/j.ecoleng.2010.03.004
Wang, R., Baldy, V., Périssol, C., and Korboulewsky, N. (2012). Influence of plants on microbial activity in a vertical-downflow wetland system treating waste activated sludge with high organic matter concentrations. J. Environ. Manag. 95, S158–S164. doi: 10.1016/j.jenvman.2011.03.021
Wang, W., Ding, Y., Wang, Y., Song, X., Ambrose, R. F., and Ullman, J. L. (2016). Intensified nitrogen removal in immobilized nitrifier enhanced constructed wetlands with external carbon addition. Biores. Technol. 218, 1261–1265. doi: 10.1016/j.biortech.2016.06.135
Wang, W., Song, X., Li, F., Ji, X., and Hou, M. (2020). Intensified nitrogen removal in constructed wetlands by novel spray aeration system and different influent COD/N ratios. Biores. Technol. 306, 123008. doi: 10.1016/j.biortech.2020.123008
Wang, X., Yang, R., Guo, Y., Zhang, Z., Kao, C. M., and Chen, S. (2019). Investigation of COD and COD/N ratio for the dominance of anammox pathway for nitrogen removal via isotope labelling technique and the relevant bacteria. J. Hazard. Mater. 366, 606–614. doi: 10.1016/j.jhazmat.2018.12.036
Wei, J. M., Cui, L. J., Li, W., Ping, Y. M., and Li, W. (2021). Denitrifying bacterial communities in surface-flow constructed wetlands during different seasons: characteristics and relationships with environment factors. Sci. Rep. 11, 1–9. doi: 10.1038/s41598-021-82438-3
Wei, T., and Simko, V. (2021). R package “corrplot”: Visualization of a Correlation Matrix (Version 0.92). Vienna.
Willems, A., Busse, J., Goor, M., Pot, B., Falsen, E., Jantzen, E., et al. (1989). Hydrogenophaga, a new genus of hydrogen-oxidizing bacteria that includes Hydrogenophaga flava comb. nov. (formerly Pseudomonas flava), Hydrogenophaga palleronii (formerly Pseudomonas palleronii), Hydrogenophaga pseudoflava (formerly Pseudomonas pseudoflava and “Pseudomonas carboxydoflava”), and Hydrogenophaga taeniospiralis (formerly Pseudomonas taeniospiralis). Int. J. System. Evolution. Microbiol. 39, 319–333. doi: 10.1099/00207713-39-3-319
Wu, H., Fan, J., Zhang, J., Ngo, H. H., Guo, W., Hu, Z., et al. (2015). Decentralized domestic wastewater treatment using intermittently aerated vertical flow constructed wetlands: impact of influent strengths. Biores. Technol. 176, 163–168. doi: 10.1016/j.biortech.2014.11.041
Wu, S., Kuschk, P., Brix, H., Vymazal, J., and Dong, R. (2014). Development of constructed wetlands in performance intensifications for wastewater treatment: a nitrogen and organic matter targeted review. Water Res. 57, 40–55. doi: 10.1016/j.watres.2014.03.020
Wu, S., Zhang, D., Austin, D., Dong, R., and Pang, C. (2011). Evaluation of a lab-scale tidal flow constructed wetland performance: Oxygen transfer capacity, organic matter and ammonium removal. Ecol. Eng. 37, 1789–1795. doi: 10.1016/j.ecoleng.2011.06.026
Wu, Y., Han, R., Yang, X., Fang, X., Chen, X., Yang, D., et al. (2016). Correlating microbial community with physicochemical indices and structures of a full-scale integrated constructed wetland system. Appl. Microbiol. Biotechnol. 100, 6917–6926. doi: 10.1007/s00253-016-7526-4
Yang, Y., Zhao, Y., Wang, S., Guo, X., Ren, Y., Wang, L., et al. (2011). A promising approach of reject water treatment using a tidal flow constructed wetland system employing alum sludge as main substrate. Water Sci. Technol. 63, 2367–2373. doi: 10.2166/wst.2011.575
Ye, F., and Li, Y. (2009). Enhancement of nitrogen removal in towery hybrid constructed wetland to treat domestic wastewater for small rural communities. Ecol. Eng. 35, 1043–1050. doi: 10.1016/j.ecoleng.2009.03.009
Yu, G., Peng, H., Fu, Y., Yan, X., Du, C., and Chen, H. (2019). Enhanced nitrogen removal of low C/N wastewater in constructed wetlands with co-immobilizing solid carbon source and denitrifying bacteria. Biores. Technol. 280, 337–344. doi: 10.1016/j.biortech.2019.02.043
Zhang, M., Huang, J. C., Sun, S., Rehman, M. M. U., He, S., and Zhou, W. (2021). Nitrogen removal through collaborative microbial pathways in tidal flow constructed wetlands. Sci. Total Environ. 758, 143594. doi: 10.1016/j.scitotenv.2020.143594
Zhao, Y., Sun, G., Lafferty, C., and Allen, S. (2004). Optimising the performance of a lab-scale tidal flow reed bed system treating agricultural wastewater. Water Sci. Technol. 50, 65–72. doi: 10.2166/wst.2004.0490
Zheng, F., Fang, J., Guo, F., Yang, X., Liu, T., Chen, M., et al. (2022). Biochar based constructed wetland for secondary effluent treatment: Waste resource utilization. Chem. Eng. J. 432, 134377. doi: 10.1016/j.cej.2021.134377
Zhi, W., and Ji, G. (2014). Quantitative response relationships between nitrogen transformation rates and nitrogen functional genes in a tidal flow constructed wetland under C/N ratio constraints. Water Res. 64, 32–41. doi: 10.1016/j.watres.2014.06.035
Zhu, T., Gao, J., Huang, Z., Shang, N., Gao, J., Zhang, J., et al. (2021). Comparison of performance of two large-scale vertical-flow constructed wetlands treating wastewater treatment plant tail-water: Contaminants removal and associated microbial community. J. Environ. Manag. 278, 111564. doi: 10.1016/j.jenvman.2020.111564
Keywords: biological treatment, carbon dosage, microbial structure, nature-based solutions, nitrification-denitrification, nutrients removal
Citation: Khajah M, Bydalek F, Babatunde AO, Al-Matouq A, Wenk J and Webster G (2023) Nitrogen removal performance and bacterial community analysis of a multistage step-feeding tidal flow constructed wetland. Front. Water 5:1128901. doi: 10.3389/frwa.2023.1128901
Received: 21 December 2022; Accepted: 06 February 2023;
Published: 23 February 2023.
Edited by:
Cristina Matos, University of Trás-os-Montes and Alto Douro, PortugalReviewed by:
Yujie He, Nanjing University, ChinaYuriy Litti, Federal Center Research Fundamentals of Biotechnology (RAS), Russia
Copyright © 2023 Khajah, Bydalek, Babatunde, Al-Matouq, Wenk and Webster. This is an open-access article distributed under the terms of the Creative Commons Attribution License (CC BY). The use, distribution or reproduction in other forums is permitted, provided the original author(s) and the copyright owner(s) are credited and that the original publication in this journal is cited, in accordance with accepted academic practice. No use, distribution or reproduction is permitted which does not comply with these terms.
*Correspondence: Mishari Khajah, bWtoYWphaEBraXNyLmVkdS5rdw==