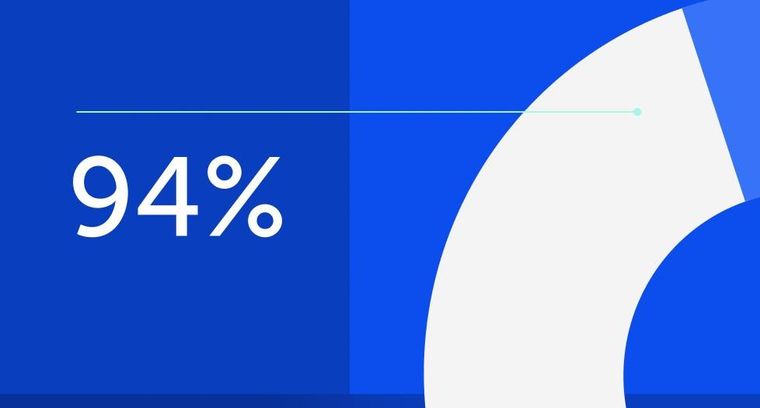
94% of researchers rate our articles as excellent or good
Learn more about the work of our research integrity team to safeguard the quality of each article we publish.
Find out more
REVIEW article
Front. Water, 09 March 2023
Sec. Water and Hydrocomplexity
Volume 5 - 2023 | https://doi.org/10.3389/frwa.2023.1084050
This article is part of the Research TopicHorizons in Water 2022View all 4 articles
Lakes and reservoirs are important for environmental anthropogenic functions in terms of agriculture and/or settlements. Here we present a first global overview of their chemistry by considering 1,508 water bodies, with data from 485 peer-reviewed publications from 1868 to 2020 and further five online databases. This work focusses on major ions (Ca2+, Mg2+, Na+, K+, &, Cl−, ) and investigates analogies as well as differences between lakes and reservoirs. We applied a Principal Component Analysis (PCA) to group both types of water bodies and to find differences and similarities. The PCA identified fewer variabilities for major ions in reservoirs than in lakes. Moreover, our analyses showed that lakes generally have more total dissolved solids (TDS). Such higher TDS loads in lakes could result from more diverse (and less controlled) inputs from larger catchments and from longer lasting interactions with thicker internal sediment layers. Global median geochemical compositions identified both reservoirs and lakes as calcium-bicarbonate-type waters. This first synthesis provides a basis for future studies and may serve as the start of a global database on these important water bodies.
During the last decades, scientific and public attention toward lakes and reservoirs increased mainly due to their role in water use for human consumption as well as for irrigation and recreation (Friese et al., 2014; Wentzky et al., 2018; Mi et al., 2020; Dordoni et al., 2022a,b). Other roles of reservoirs include flood mitigation and energy supply. Reservoirs and lakes also serve as unique reflectors of environmental change with their chemical, biological and hydrological responses to external drivers and potential changes in economic pressures in their catchments (Graf, 1999; Phyoe and Wang, 2019; Shi et al., 2019). Among these influences, anthropogenic land use is known as one of the most important drivers for water chemistry (Skoulikidis, 1993; Marmen et al., 2020). In particular, agriculture exerts strong influences on water quality via fertilizers. The release of such substances can also increase eutrophication (FAO/ECE, 1991; Galloway et al., 2017).
Different origins and purpose of use of lakes and reservoirs also sparked diverse scientific interests (Liu et al., 2016; Amundsen et al., 2018; Wang et al., 2019; Pu et al., 2020; Biskaborn et al., 2021). For example, studies on lakes often focus on biological communities and often do not consider surrounding landscapes and their catchments (Maberly et al., 2012). On the other hand, reservoir studies often deal with technical issues such dam integrity and water management for downstream section (Wheeler et al., 2020). In paleoclimatology, lake sediments serve as archives for centuries to millennia, whereas most reservoirs were created within the past 50 to 150 years. Because sediments in reservoirs can only provide information for several decades at best, their sedimentary records are only able to provide information on shorter-time scales (Lehner et al., 2011).
The World Commission on Dams (WCD) estimated that large dams and reservoirs contribute between 12 and 16% of the global food production. Dams and reservoirs are further estimated to provide about 19% of the global electricity supply in more than 150 countries (WCD, 2000). This is also reflected in the unprecedented acceleration of dam construction during the last six decades, especially in emerging economies, in response to rising water and energy demands (Berga et al., 2006; Klingensmith, 2007; Zarfl et al., 2015; Dillon et al., 2019; Shi et al., 2019). The consequences of these constructions include degradation of aquatic ecosystems and their biological communities due to flow regulation and fragmentation of habitats and reduced genetic exchange between populations (Jansson et al., 2000; Pringle et al., 2000; Freeman and Marcinek, 2006; Xie et al., 2007).
In terms of their limnological characteristics, reservoirs can show different properties when compared to natural lakes. Straskraba (1998) and Hayes et al. (2017) provided thorough analyses from a limnological perspective. One aspect to water quality is that reservoirs often have larger relative through-flow volumes and shorter residence times (Hayes et al., 2017). While well-flushed lakes may behave like reservoirs, most lakes with longer residence times of up to several decades are usually more affected by internal dynamics and sediment-water interactions (Findlay, 1995; Valett et al., 1996). These limnological differences between lakes and reservoirs raise the question whether they also show systematic differences in their chemical characteristics. Several studies have already addressed chemical differences between open- and closed-basins that host water bodies with or without inlets or outlets (Eugster and Hardie, 1978; Yan et al., 2002). However, it remains challenging to apply these findings to show chemical similarities and differences between lakes and reservoirs.
Reservoirs also affect hydrological and biogeochemical functions at continental scales. For instance, reservoirs were estimated to have increased the total continental water surface area by about 7% in the last 150 years (Lehner et al., 2011). This has implications on continental evaporation, exchange of gases with the atmosphere and on flow regimes of rivers and streams as well as their dissolved load and sediment transport and storage (Martins and Nurudeen, 1988; Shiklomanov, 2000; Syvitski et al., 2005; Wang et al., 2011). One consequence is that reservoirs represent additional sources of methane and CO2 to the atmosphere that can be emitted through turbines and spillways as well as from sediments (Fearnside and Pueyo, 2012). In addition, temporary drying of sediments that result from management operations are known to enhance CO2 emissions (Keller et al., 2020). On the river basin scale, reservoirs act as sediment traps and substantially increase the retention of phosphorus (Maavara et al., 2015) and silica (Maavara et al., 2014) as well as the burial of carbon (Mendonca et al., 2017). Via these mechanisms of sediment trapping, the establishment of reservoirs also changed the aqueous chemistry of many rivers over part of their courses. Biogeochemical implications of these continental surface water changes include alterations of carbon fluxes, modification of nutrient budgets, changing oxygenation of water bodies and changes in their major ion chemistry (Groeger and Kimmel, 1984; Friedl, 2002; Kraus et al., 2011; Wang et al., 2014; Mendonca et al., 2017). To date, these processes have hardly been addressed on a global perspective and a good start is to summarize and compare their major ions.
With increasing numbers of reservoirs and increased global water demands that also concern lakes, a baseline study that outlines hydrochemical similarities and differences between these water bodies is useful to judge quality as an additional parameter to quantity. In the work presented here, we considered standing water bodies that differ in their geological backgrounds, salinities, depths, trophic levels, climate zones, hydrology and their degree of influences by land use. Our principal aims were to outline similarities and differences of lake and reservoirs via a Principal Component Analysis (PCA) and investigation of selected major ion groups, such as Ca2+& Mg2+ and dissolved inorganic carbon (DIC). With this, the presented outline could serve as a new basis to characterize these water bodies. This work may also supply a first tool for comparison to future studies and establish new connections to databases such as the Global River Chemistry database (GLORICH) (Hartmann et al., 2019) or data on groundwater (https://www.un-igrac.org/) for collaborative monitoring work on terrestrial water systems.
Data were retrieved from 485 peer-reviewed publications from 1868 to 2020. The locations of all water bodies used for aqueous chemistry investigations (663 lakes and 106 reservoirs) are provided in Figure 1. All peer-reviewed publications used in this study are listed in the Supplementary material. In addition, freely available online databases with data up to 2020 were researched (Table 1).
Table 1. List of online databases explored for this study with internet links. Last visited: 6 October 2022.
Because our intention was to describe lakes and reservoirs on the global scale, we gathered literature about water bodies in different geographical regions and with diverse physical and chemical characteristics. The lakes and reservoirs included in these considerations are located in North America, Asia, Africa, Europe, Australia, South America, and Antarctica. They cover an altitude range from 430 m below sea level (Dead Sea) to 5,140 m above sea level (Garig Co, China). With this, we considered catchments with different lithologies. Differences in the major ion compositions dissolved are to a large extent influenced by weathering of different rock types (Wang et al., 2020). Therefore, further in-depth studies that consider geology, climate, and land-use separately could make up follow-up work to this data set. Note that wetlands and lagoons were not included in this work.
Climate classes of the Updated Köppen-Geiger (UKG) classification were attributed to the selected water bodies following Peel et al. (2007). These climate classes were applied at a resolution of 0.1 degrees in both latitude and longitude. When a water body fell into two climatic classes, we considered it twice as a representative of both classes. Frequencies of occurrence and percentage of our data base with the respective climate classes are listed in Supplementary Table S1. Note that most surface water samples were collected during the warm season and during daytime, mostly close to the ouflow of the water bodies. Therefore, influences of seasonal or diel influences and stratification in standing water bodies cannot be interpreted by these data.
Moreover, in the work presented here we focused on major ion chemistry (Ca2+, Mg2+, Na+, K+, & , Cl−, ). These are among the most frequently reported variables in our database, and basic hydrochemical classifications of waters traditionally rely on these ions. Our analyses were based on data in mmol L−1. When ion concentrations were provided in other units, they were converted accordingly. When DIC concentrations were supplied as different variables than concentrations of hydrogencarbonate, they were converted into & . Data used for our investigations refer to selected analyses with errors of ion balances smaller than 10%. This corresponds to 247 lakes and 66 reservoirs. The threshold of a 10%-ion balance seems a reasonable quality indicator. This holds especially true when considering that most analyses were carried out with different methods including titrations, ion chromatography and spectrophotometry. We also considered total dissolved solids (TDS) in water. This parameter was often provided in the literature or else it was calculated based on cations and anions contents.
The selected major ion data were analyzed by the PCA. None of the major ions investigated followed a Gaussian distribution. They were therefore treated according to the optimal box-cox transformation (Box and Cox, 1964). The PCA was conducted according to Jolliffe (2011) and used major ions as independent variables with the aim to separate reservoirs and lakes from a hydrochemical point of view. In our models, we refused the null hypothesis of a t-test when the p-value was higher than 0.01. We always chose the models with the lowest Akaike Information Criterion (AIC) (Akaike, 1974). Subsequently, a Kruskal-Wallis test was performed in order to predict if lakes and reservoirs had the same distribution (Kruskal and Wallis, 1952; Table 2). The Bartlett test was also applied to evaluate the variance of each dataset (Table 3; Bartlett and Fowler, 1937).
Based on major ion compositions from the same suite of samples, we determined global average and median lake- and reservoir-compositions. For this purpose, we used the volume of each water body to calculate their weighted values. Volumes were either retrieved from the literature data or else they were evaluated by the areas of the respective water bodies multiplied by their average depth.
Our literature search yielded a total of 33 chemical variables (Supplementary Table S2). Several of these parameters were measured only occasionally. The most frequently observed variables include carbon species (DIC, DOC, and POC) and major ions (Ca2+, Mg2+, Na+, K+, , Cl−, and ). Chlorophyll was available for ~25% of the water bodies studied. We did not further consider nutrients, chlorophyll, organic carbon and dissolved gases because they were most frequently measured in lakes.
Note that the water bodies in our database are not equally distributed over climate zones, with continental and temperate climates accounting for ~75% of the whole dataset (Supplementary Table S1).
The investigated water bodies differ largely by volume, with the smallest one (Lake Blake Mere in the UK) being around ten orders of magnitude smaller than the biggest one (Caspian Sea). The median volume of the analyzed lakes is 0.015 km3. This shows that most of them are small to medium-sized water bodies. Reservoirs also show a strong variability in terms of volume, with seven orders of magnitude between the smallest (the Arligton Reservoir in Texas) and the biggest one (the Guri Reservoir in Venezuela). The median volume of all reservoirs analyzed is 0.16 km3.
Our PCA was performed on the first four components (PC I to PC IV). They covered 88% of the total variance of the dataset. This led to the following observations:
- The increase of PC I associates with increases of all variables
- The increase of PC II associates with increases of Ca2+ and Mg2+, while K+ decreases
- The increase of PC III associates with increases of K+, while & decreases
- The increase of PC IV associates with increases of K+ and & , while and Cl− decrease
All PCA loadings are outlined in Supplementary Table S3 and variances are available in Figure 2A. The Kruskal-Wallis test on the four components (Table 2) showed that lakes and reservoirs had different distributions over PCII and PCIII.
Figure 2. (A) Eigenvalues, (B) Plots of PCA loadings on PC II and PC III, (C) Output of the PCA on PC II and PC III. Lakes are marked in blue and reservoirs in red. The green circle marks the “RA96”-field that hosts most reservoirs.
Based on these results, we considered only PC II and PC III because of their low p-values and their best separation between reservoirs and lakes. Figures 2B, C present PC II and PC III. The Bartlett test suggests that reservoirs and lakes have different variances on PC II (Figure 2 and Table 3). Figure 2C shows that Ca2+, Mg2+, K+, and & can help to distinguish lakes from reservoirs. Here, increasing values on the x-axis correspond to elevated Ca2+ and Mg2+ contents, whereas increasing values on the y-axis indicate elevated K+ and decreasing & contents. This crossplot between the two principal components shows that reservoirs have much narrower geochemical distributions when compared to those of lakes. Within this diagram, we identified an area that hosts 96% of all analyzed reservoirs as marked by the circle in Figure 2C. We named this field “RA96.” The Kruskal-Wallis test also confirmed that the dataset of reservoirs and the one of lakes do not belong to the same population and have different distributions.
In order to confirm that reservoirs are more concentrated in the RA96-field of Figure 2C, we also performed different statistical tests under two hypotheses:
- The null hypothesis that assumes reservoirs and lakes to have the same distribution and variance
- The alternative hypothesis that assumes reservoirs to be more concentrated in the RA96-field rather than in the remaining area
The hypothesis was tested by a binomial analysis (Dodge, 2008). The p-value of the test is:
p (X ≥ 96 | X ~ Bin (N, p)) = 0.003
This result shows that the water bodies are unlikely to be randomly distributed within the plot. It confirms the assumption that reservoirs preferably concentrate in the RA96-field due to their hydrochemical characteristics. More details of the PCA are available in the Supplementary material.
Global average and mean compositions of lake and reservoir waters were weighted by their volumes (Table 4 and Figure 3). According to Piper (1944), the weighted average composition for lakes is a sodium-chloride-type water characterized by high contents in Cl− and Na+ & K+ and lower contents in & and Ca2+. In comparison, the weighted average for reservoirs falls on the boundary between sodium-chloride-types and calcium-sulfate waters. According to the global averages, lakes have a major anion concentration ranking with Cl− > > & . In terms of their cations, they rank with Na+ & K+ > Mg2+ > Ca2+. In comparison, the average chemical composition of reservoirs is lower in Cl− but has roughly equal average concentrations of & and . Additionally, cation contents are generally lower in reservoirs with Na+ & K+ > Ca2+ > Mg2+.
Table 4. Global volume-weighted compositions of lake and reservoir waters reported as averages and medians (the latter in brackets). Values (in mmol L−1) refer to analyses in our database with ion balances <10% (247 lakes, 66 reservoirs).
When considering global weighted median values, lakes and reservoirs are more similar and only differ in their cation contents. In lakes, the median cation composition assumes almost equal proportions of Na+ & K+, Ca2+ and Mg2+, whereas this distribution is Ca2+ > Na+ & K+ > Mg2+ in reservoirs. Moreover, TDS values are generally higher in lakes than in reservoirs.
Two groups were established for comparison of selected molar concentrations of ions of of Ca2+ & Mg2+ versus & :
- those with total dissolved solids (TDS) <100 mg L−1 and
- those with TDS >100 mg L−1.
With this grouping, Figure 4 shows correlations between Ca2+ & Mg2+ and & that also outline several fields. Field “1” shows an excess of & , field “2” marks samples with lower TDS and roughly equal amounts of & and Ca2+ & Mg2+, while field “3” shows excess Ca2+ & Mg2+ over & . Note that only lake waters occur in fields “1” and “3,” and also show higher TDS. Field “3” hosts lakes with the highest ion concentrations, 71% of which are located in regions for continental climate (type D according to Köppen). On the other hand, reservoir waters are found solely in field “2” and show lower TDS.
Figure 4. Geochemical relationships between Ca2+ and Mg2+ and and in lakes (blue; n = 247) and reservoirs (red; n = 66). Sizes of symbols vary with total dissolved solids (TDS) with bigger symbols for TDS >100 mg L−1 and smaller symbols for TDS <100 mg L−1. The black line marks the 1:1 ratio of ( and ):(Ca2+ and Mg2+). Field “1” shows excess of and , field “2” shows roughly balanced and and Ca2+ and Mg2+ and field “3” shows excess of Ca2+ and Mg2+ over and .
Our PCA outlined clear geochemical differences between lakes and reservoirs (Figure 2). With the sufficiently large sample populations, these differences are unlikely due to uneven numbers of lakes and reservoirs in the database. One explanation for lower variabilities and narrower ranges of major ion compositions in reservoirs may be their purpose for drinking water supply that foresees higher water quality standards. Additionally, reservoirs usually have more frequent water exchanges (Hayes et al., 2017). Such shorter residence times may contribute to a narrower distribution of reservoirs in our PCA analysis and may have caused the reservoir samples to fall within the RA96 field.
Among the major ions presented, K+ is a common component of commercial fertilizers and can also serve as a good reflector of agricultural influences (Scherer, 2005). Note that high K+concentrations in waters do not necessarily indicate fertilization and could also stem from cation exchange (Binner et al., 2017). Alternatively, K+ could also derive from the weathering of K-bearing minerals, such as biotite or orthoclase (Berner and Berner, 2015; Marx et al., 2017). However, because these minerals are part of rocks with low weathering rates, fertilizers likely assume a more dominant contribution to the water bodies of our study. Other studies confirm that agriculture can exert strong influences on the water chemistry of lakes (Marmen at al., 2020). Reservoirs are also often subject to more strict rules regarding anthropogenic land use changes. On the other hand, lakes are located in more variable environments in terms of land use. Usually higher agricultural and urban inputs, may explain why they have a higher potential to become enriched in K+ as shown by our PCA analyses (Figure 2C).
Geographical settings of reservoirs are usually designed to allow less diverse possibilities of chemical inputs (Hayes et al., 2017). This fact offers a good argument for the finding that reservoirs have lower TDS. In addition, most lakes were able to build up sedimentary records over hundreds to thousands of years, while reservoirs are younger environments that accumulated sediments for only several tens of years (Lehner et al., 2011). It seems plausible that at least for shallower lakes and temperate lakes with regular lake turnovers, exchanges between thicker sedimentary layers and the free water column could increase ion concentrations and variabilities of major ions. In addition, residence times are generally longer in lakes, and reservoirs are often deeper with respect to their surface area (Hayes et al., 2017). Deeper water bodies result in a smaller surface area with respect to their volume, which may cause less evaporation. In contrast, lakes with shallower water bodies are more affected by evaporation, which can influence TDS.
Regarding global chemical compositions, weighted averages are more influenced by larger water bodies, which make up only a small group of our database. We therefore consider the weighted medians as the better choice to represent global geochemical compositions of lakes and reservoirs. Weighted median values highlight & as the major anions in both lakes and reservoirs. This finding is consistent with that of Wetzel (1983). The strongest differences between both water bodies was found in their cation concentrations. In particular, K+ contents are smaller in reservoirs when compared to those of lakes. Again, this likely reflects less agricultural inputs that adhere to stricter rules for drinking water storage.
Next to the overall positive trend in Figure 4, the studied lakes showed higher TDS values. In field “1,” the majority of lakes analyzed have no surface outflows and are characterized by bicarbonate waters and/or high alkali contents (e.g., Lake Bogoria, Mono Lake, Alkali Lake and Borax Lake). This may be related to the fact that this field hosts lakes that are subject to strong evaporation with resulting higher TDS.
Field “2” in Figure 4 groups samples with low TDS that characterizes water bodies with moderate to low ion contents. These waters were likely subject to less evaporation. Note that field “2” hosts all reservoirs of our database. Here the data are also close to a 1:1 ratio between Ca2+ & Mg2+ and & . A plausible chemical evolution of these waters would be dominant hydrolysis of feldspar (Earle and Panchuk, 2019). A typical corresponding equation can be written as:
The ranking in weathering ability for this process is second in magnitude when compared to calcite and dolomite dissolution (Schnoor and Stumm, 1986). This suggests that most reservoirs and a large part of the studied lakes are located in regions that are influenced by this type of weathering. Variances may also be controlled by catchment size and residence times of waters in the subsurface.
Field “3” in Figure 4 hosts lakes with high TDS. This field hosts many non-bicarbonate lakes located in arid areas or endorheic basins (e.g., Aral Sea, Dead Sea, Caspian Sea, and Lake Urmia). Many lakes in this region of the plot are influenced by weathering of evaporites, such as Qarhan Salt Lake (Fan et al., 2018). Another group of lakes often present in this field consists of amictic lakes that are covered by ice. They include for instance Lake Bonney in Antarctica, with a permanent ice-cover and proximity to the ocean that enrich waters in salts. Field “3” also comprises saline lakes with their chemistry driven by arid climate (e.g., Qinghai Lake, Gahai Lake). Anthropogenic factors include water withdrawal for agricultural purposes (e.g., Big Quill Lake). Such trends are confirmed by hydrological models by Eugster and Hardie (1978) that indicate ion enrichments in arid climates due to enhanced evaporation. Note that an excess of Ca2+& Mg2+ may also result from dissolution of Ca- and Mg-bearing minerals by carbon free acids, such as sulfuric or nitric acids from acid rain deposition (Berner and Berner, 2015).
Overall, our data show that geology, climate and human activities seem to be the main drivers that define the geochemical composition of the standing water bodies investigated. Similar dependencies were also found for groundwaters that are connected to surface water bodies (DeSimone et al., 2015).
In this study, we analyzed a total of 485 publications and five online databases on lakes and reservoirs. A PCA yielded an ion distribution plot that concentrates reservoirs in a narrower field than lakes. Moreover, the presented database also provides a first evaluation of global average and median water compositions for lakes and reservoirs as weighted by their water volumes. Weighted medians revealed calcium-bicarbonate type waters for both types of water bodies.
We generally recorded smaller values of total dissolved solids in reservoirs when compared to those of lakes. Associated larger variances of TDS in lakes likely reflect the fact that they have more diverse and less controlled inputs from larger catchments. This finding also implies that lakes may be more at risk to undergo environmental change by a larger variety of possible environmental influences.
For instance, agricultural activities play an important role in anthropogenic and land use influences. These were best reflected by K+ that serves as a good indicator of fertilizers. The data presented here reveal that reservoirs show lower K+ concentrations. This seems plausible, because they are often located in higher altitude areas with less pronounced agricultural activities. Further investigations on fertilizers and pesticides may reveal better relationships to agricultural influences and possible risks of eutrophication and algae blooms.
Our study offers a first global overview of chemical characteristics of lakes and reservoirs. It offers a platform for future global-scale biogeochemical studies, and may help to establish links to other global databases such as the Global Reservoir and Dam database (http://globaldamwatch.org/grand/) or the GLORICH database on rivers (https://www.geo.uni-hamburg.de/en/geologie/forschung/aquatische-geochemie/glorich).
Future considerations should also include other environmental parameters such as dissolved gases, nutrients or stable and radio isotopes that can act as useful tools to outline origins of water and its dissolved constituents. The presented database could additionally expand to closer investigation of climate, geology and land use. Future work could also include information on the water column and depth profiles, as they can provide seasonal insights linked to stratification. This approach would not only provide more information but would also help to further investigate observed differences between lakes and reservoirs.
MD performed the formal analysis and was responsible for investigation and data curation and visualization. MD and PZ arranged the conceptualization, methodology, and validation. MD and JB arranged the writing of the original draft and its editing. JB was responsible for project administration and acquisition of funding. All authors contributed to the article and approved the submitted version.
This work was supported by the Deutsche Forschungsgemeinschaft (DFG) [BA 2207/18-1 and RI 2040/4-1]. Part of this work was also funded in the framework of the project AquaKlif in the bayklif network for investigation of regional climate change funded by the Bavarian State Ministry of Science and the Arts.
We are indebted to Karsten Rinke and Michael Seewald for their advice and to all providers of data and literature that was used for this study.
The authors declare that the research was conducted in the absence of any commercial or financial relationships that could be construed as a potential conflict of interest.
All claims expressed in this article are solely those of the authors and do not necessarily represent those of their affiliated organizations, or those of the publisher, the editors and the reviewers. Any product that may be evaluated in this article, or claim that may be made by its manufacturer, is not guaranteed or endorsed by the publisher.
The Supplementary Material for this article can be found online at: https://www.frontiersin.org/articles/10.3389/frwa.2023.1084050/full#supplementary-material
Akaike, H. (1974). A new look at the statistical model identification. IEEE Trans. Autom. Control 19, 716–723. doi: 10.1109/TAC.1974.1100705
Amundsen, P. A., Primicerio, R., Smalås, A., Henriksen, E. H., Knudsen, R., Kristoffersen, R., et al. (2018). Long-term ecological studies in northern lakes—Challenges, experiences, and accomplishments. Limnol. Oceanogr. 591, 5590. doi: 10.1002/lno.10951
Bartlett, M. S., and Fowler, R. H. (1937). Properties of sufficiency and statistical tests. Proc. R. Soc. London, Ser. A 160, 268–282. doi: 10.1098/rspa.1937.0109
Berga, L., Buil, J. M., Bofill, E., De Cea, J. C., Garcia Perez, J. A., Mañueco., et al. (2006). Dams and Reservoirs, Societies and Environment in the 21st Century. Barcelona: Taylor and Francis.
Berner, E. K., and Berner, R. A. (2015). Global Environment: Water, Air, and Geochemical Cycles. Princeton, NJ: Princeton University Press. doi: 10.1080/00207233.2012.753739
Binner, I., Dultz, S., Schellhorn, M., and Schenk, M. K. (2017). Potassium adsorption and release properties of clays in peat-based horticultural substrates for increasing the cultivation safety of plants. Appl. Clay Sci. 145, 28–36. doi: 10.1016/j.clay.2017.05.013
Biskaborn, B. K., Nazarova, L., Kröger, T., Pestryakova, L. A., Syrykh, L., Pfalz, G., et al. (2021). Late quaternary climate reconstruction and lead-lag relationships of biotic and sediment-geochemical indicators at Lake Bolshoe Toko, Siberia. Front. Earth Sci. 9, 7353. doi: 10.3389/feart.2021.737353
Box, G. E. P., and Cox, D. R. (1964). An analysis of transformations. J. R. Stat. Soc. Series B Stat. Methodol. 26, 211–252.
DeSimone, L., McMahon, P. B., and Rosen, M. R. (2015). The Quality of our Nation's Waters: Water Quality in Principal Aquifers of the United States, 1991–2010. USGS Report. doi: 10.3133/cir1360
Dillon, P., Stuyfzand, P., Grischek, T., Lluria, M., Pyne, R. D. G., Jain, R. C., et al. (2019). 60 years of global progress in managed aquifer recharge. Hydrogeol. J. 27, 1–30. doi: 10.1007/s10040-018-1841-z
Dodge, Y. (2008). Binomial Test, The Concise Encyclopedia of Statistics. New York: Springer New York.
Dordoni, M., Seewald, M., Rinke, K., Schmidmeier, J., and Barth, J. A. C. (2022a). Novel evaluations of sources and sinks of dissolved oxygen via stable isotopes in lentic water bodies. Sci. Total Environ. 838, 156541.doi: 10.1016/j.scitotenv.2022.156541
Dordoni, M., Seewald, M., Rinke, K., van Geldern, R., Schmidmeier, J., and Barth, J. A. C. (2022b). Mineralization of autochthonous particulate organic carbon is a fast channel of organic matter turnover in Germany's largest drinking water reservoir. Biogeosciences 19, 5343–5355. doi: 10.5194/bg-19-5343-2022
Eugster, H. P., and Hardie, L. A. (1978). “Saline Lakes” in Lakes, eds A. Lerman. New York: Springer New York. doi: 10.1007/978-1-4757-1152-3_8
Fan, Q. S., Lowenstein, T. K., Wei, H. C., Yuan, Q., Qin, Z. J., Shan, F. S., et al (2018). Sr isotope and major ion compositional evidence for formation of Qarhan Salt Lake, Western China. Chem. Geol. 497, 128–145. doi: 10.1016/j.chemgeo.2018.09.001
FAO/ECE (1991). Legislation and Measures for the Solving of Environmental Problems Resulting from Agricultural Practices (With Particular Reference to Soil, Air and Water), Their Economic Consequences and Impact on Agrarian Structures and Farm Rationalization. Geneva. United Nations Economic Commission for Europe (UNECE) and FAO, Agri/Agrarian Structures and Farm Rationalization Report No. 7. United Nations.
Fearnside, P. M., and Pueyo, S. (2012). Greenhouse-gas emissions from tropical dams. Nat. Clim. Change 2, 382–384. doi: 10.1038/nclimate1540
Findlay, S. (1995). Importance of surface-subsurface exchange in stream ecosystems: the hyporheic zone. Limnol. Oceanogr. 40, 159–164. doi: 10.4319/LO.1995.40.1.0159
Freeman, M. C., and Marcinek, P. A. (2006). Fish assemblage responses to water withdrawals and water supply reservoirs in piedmont streams. Environ. Manage. 38, 435–450. doi: 10.1007/s00267-005-0169-3
Friedl, W. (2002). Disrupting biogeochemical cycles—Consequences of damming. Aquat. Sci. 64, 55–65. doi: 10.1007/s00027-002-8054-0
Friese, K., Schultze, M., Boehrer, B., Buttner, O., Herzsprung, P., Koschorreck, M., et al. (2014). Ecological response of two hydro-morphological similar pre-dams to contrasting land-use in the Rappbode reservoirs system (Germany). Int. Rev. Hydrobiol., 99, 335–349. doi: 10.1002/iroh.201301672
Galloway, J. N., Leach, A. M., Erisman, J. W., and Bleeker, A. (2017). Nitrogen: the historical progression from ignorance to knowledge, with a view to future solutions. Soil Res 55, 417–424. doi: 10.1071/SR16334
Graf, W. L. (1999). Dam nation: a geographic census of american dams and their large-scale hydrologic impacts. Water Resour. Res. 35, 1305–1311. doi: 10.1029/1999WR900016
Groeger, A. W., and Kimmel, B. L. (1984). Organic matter supply and processing in lakes and reservoirs. Lake Reservoir Manage 1, 282–285. doi: 10.1080/07438148409354525
Hartmann, J., Lauerwald, R., and Moosdorf, N. (2019). GLORICH - Global river chemistry database, Supplement to: Hartmann, J. 2014.: A brief overview of the global river chemistry database, gloricH. Procedia Earth Planet. Sci. 10, 23–27. doi: 10.1016/j.proeps.2014.08.005
Hayes, N. M., Deemer, B. R., Corman, J. R., Razavi, N. R., and Strock, K. E. (2017). Key differences between lakes and reservoirs modify climate signals: a case for a new conceptual model. Limnol. Oceanogr. Lett. 2, 47–62. doi: 10.1002/lol2.10036
Jansson, R., Nilsson, C., and Renöfält, B. (2000). Fragmentation of riparian floras in rivers with multiple dams. Ecology 81, 899–903. doi: 10.1890/0012-9658(2000)081(0899:FORFIR)2.0.CO;2
Jolliffe, I. (2011). Principal Component Analysis. Berlin, Heidelberg: Springer. doi: 10.1007/978-3-642-04898-2_455
Keller, P. S., Catalán, N., von Schiller, D., Grossart, H.-P., Koschorreck, M., Karakaya, N., et al. (2020). Global CO2 emissions from dry inland waters share common drivers across ecosystems. Nat. Commun. 11. doi: 10.1038/s41467-020-15929-y
Klingensmith, D. (2007). “One Valley and a Thousand”: Dams, Nationalism, and Development. New DelhiOxford University Press.
Kraus, T. E. C., Bergamaschi, B. A., Hernes, P. J., Doctor, D., Kendall, C., Downing, B. D., et al. (2011). How reservoirs alter drinking water quality: organic matter sources, sinks, and transformations. Lake Reservoir Manage. 27, 205–219. doi: 10.1080/07438141.2011.597283
Kruskal, W. H., and Wallis, W. A. (1952). Use of ranks in one-criterion variance analysis. J. Am. Stat. Assoc. 47, 583–621. doi: 10.2307/2280779
Lehner, B., Reidy Liermann, C., Revenga, C., Vörösmarty, C., Fekete, B., Crouzet, P., et al. (2011). High-resolution mapping of the world's reservoirs and dams for sustainable river-flow management. Front. Ecol. Environ. 9, 494–502. doi: 10.1890/100125
Liu, M., Xu, X., Wang, D., Sun, A. Y., and Wang, K. (2016). Karst catchments exhibited higher degradation stress from climate change than the non-karst catchments in southwest China: an ecohydrological perspective. J. Hydrol. 535, 173–180. doi: 10.1016/j.jhydrol.2016.01.033
Maavara, T., Dürr, H. H., and Van Cappellen, P. (2014). Worldwide retention of nutrient silicon by river damming: from sparse data set to global estimate. Global Biogeochem. Cycles 28, 842–855. doi: 10.1002/2014GB004875
Maavara, T., Parsons, C., Ridenour, C., Stojanovic, S., Dürr, H., Powley, H., et al. (2015). Global phosphorus retention by river damming. Proc. Natl. Acad. Sci. 112, 15603–15608. doi: 10.1073/pnas.1511797112
Maberly, S. C., Barker, P. A., Stott, A. W., and De Ville, M. M. (2012). Catchment productivity controls CO2 emissions from lakes. Nat. Clim. Change 3, 391–394. doi: 10.1038/nclimate1748
Marmen, S., Blank, L., Al-Ashhab, A., Malik, A., Ganzert, L., Lalzar, M., et al. (2020). The role of land use types and water chemical properties in structuring the microbiomes of a connected lake system. Front. Microbiol. 11, 89. doi: 10.3389/fmicb.2020.00089
Martins, O., and Nurudeen, S. I. (1988). “Hydrology and geochemistry of Kainji Lake - A Reappraisal” in Transport of Carbon and Minerals in Major World Rivers, Lakes and Estuaries, eds E.T. Degens, S. Kempe, and A.S. Naidu (Mittl. Geol.-Paläont. Inst. Univ. Hamb).
Marx, A., Dusek, J., Jankovec, J., Sanda, M., Vogel, T., van Geldern, R., et al. (2017). A review of CO2 and associated carbon dynamics in headwater streams: a global perspective. Rev. Geophys. 55, 560–585. doi: 10.1002/2016RG000547
Mendonca, R., Muller, R. A., Clow, D., Verpoorter, C., Raymond, P., Tranvik, L. J., et al. (2017). Organic carbon burial in global lakes and reservoirs. Nat. Commun. 8, 1789. doi: 10.1038/s41467-017-01789-6
Mi, C., Shatwell, T., Ma, J., Wentzky, V. C., Boehrer, B., Xu, Y., et al. (2020). The formation of a metalimnetic oxygen minimum exemplifies how ecosystem dynamics shape biogeochemical processes: a modelling study. Water Res. 3, 115701. doi: 10.1016/j.watres.2020.115701
Peel, M. C., Finlayson, B. L., and McMahon, T. A. (2007). Updated world map of the Köppen-Geiger climate classification. Hydrol. Earth Syst. Sci. 11, 1633–1644. doi: 10.5194/hess-11-1633-2007
Phyoe, W. W., and Wang, F. (2019). A review of carbon sink or source effect on artificial reservoirs. Int. J. Environ. Sci. Technol. 16, 2161–2174. doi: 10.1007/s13762-019-02237-2
Piper, A. M. (1944). A graphic procedure in the geochemical interpretation of water-analyses. Eos 25, 914–928. doi: 10.1029/TR025i006p00914
Pringle, C. M., Freeman, M. C., and Freeman, B. J. (2000). Regional effects of hydrologic alterations on riverine macrobiota in the new world: tropical-temperate comparisons: Bioscience 50, 807–823. doi: 10.1641/0006-3568(2000)050(0807:REOHAO)2.0.CO;2
Pu, J., Li, J., Zhang, T., Martin, J. B., and Yuan, D. (2020). Varying thermal structure controls the dynamics of CO2 emissions from a subtropical reservoir, south China. Water Res. 178, 115831. doi: 10.1016/j.watres.2020.115831
Scherer, H. W. (2005). “Fertilizers and Fertilization” in: Encyclopedia of Soils in the Environment, eds Hillel D (Science Direct).
Schnoor, J. L., and Stumm, W. (1986). Role of chemical weathering in the neutralization of acidic deposition. Schweiz. Z. Hydrol. 48, 171–195. doi: 10.1007/BF02560197
Shi, H., Chen, J., Liu, S., and Sivakumar, B. (2019). The role of large dams in promoting economic development under the pressure of population growth. Sustainability 11, 2965. doi: 10.3390/su11102965
Shiklomanov, I. A. (2000). Appraisal and assessment of world water resources. Water Int. 25, 11–32. doi: 10.1080/02508060008686794
Skoulikidis, N. T. (1993). Significance evaluation of factors controlling river water composition. Environ. Geol. 22, 178–185. doi: 10.1007/BF00789329
Straskraba, M. (1998). Limnological differences between deep valley reservoirs and deep lakes. Int. Rev. Hydrobiol. 83, 1–12.
Syvitski, J. P. M., Vörösmarty, C. J., Kettner, A. J., and Green, P. (2005). Impact of humans on the flux of terrestrial sediment to the global coastal ocean. Science 308, 376–380. doi: 10.1126/science.1109454
Valett, H. M., Morrice, J. A., Dahm, C. N., and Campana, M. E. (1996). Parent lithology, surface-groundwater exchange, and nitrate retention in headwater streams. Limnol. Oceanogr. 41, 333–345. doi: 10.4319/lo.1996.41.2.0333
Wang, B., Zhang, H., Liang, X., Li, X., and Wang, F. (2019). Cumulative effects of cascade dams on river water cycle: evidence from hydrogen and oxygen isotopes. J. Hydrol. 568, 604–610. doi: 10.1016/j.jhydrol.2018.11.016
Wang, W., Wang, X. G., and Zhou, X. (2011). Impacts of Californian dams on flow regime and maximum/minimum flow probability distribution. Hydrol. Res. 42, 275–289. doi: 10.2166/nh.2011.137
Wang, W., Yi, Y., Zhong, J., Kumar, A., and Li, S. L. (2020). Carbon biogeochemical processes in a subtropical karst river–reservoir system. J. Hydrol. 591, 125590. doi: 10.1016/j.jhydrol.2020.125590
Wang, Y. F., Chen, L. D., Gao, Y., Wang, S., Lu, Y. H., and Fu, B. J. (2014). Carbon sequestration function of check-dams: a case study of the loess plateau in China. Ambio 43, 926–931. doi: 10.1007/s13280-014-0518-7
WCD (2000). Dams and Development: A New Framework for Decision-making: the Report of the World Commission on Dams.
Wentzky, V., Tittel, J., Jäger, C. G., and Rinke, K. (2018). Mechanisms preventing a decrease in phytoplankton biomass after phosphorus reductions in a German drinking water reservoir—results from more than 50 years of observation. Freshwater Biol. 63, 1063–1076. doi: 10.1111/fwb.13116
Wheeler, K. G., Jeuland, M., Hall, J. W., Zagona, E., and Whittington, D. (2020). Understanding and managing new risks on the Nile with the Grand Ethiopian Renaissance Dam. Nat. Commun 11, 5222. doi: 10.1038/s41467-020-19089-x
Xie, S., Li, Z., Liu, J., Xie, S., Wang, H., and Murphy, B. R. (2007). Fisheries of the yangtze river show immediate impacts of the three gorges dam. Fisheries 32, 343–344.
Yan, J., Hinderer, M., and Einsele, G. (2002). Geochemical evolution of closed-basin lakes: general model and application to Lakes Qinghai and Turkana. Sediment. Geol. 148, 105–122. doi: 10.1016/S0037-0738(01)00212-3
Keywords: lakes, reservoirs, major ions, geochemistry, principal component analysis-PCA
Citation: Dordoni M, Zappalà P and Barth JAC (2023) A preliminary global hydrochemical comparison of lakes and reservoirs. Front. Water 5:1084050. doi: 10.3389/frwa.2023.1084050
Received: 29 October 2022; Accepted: 17 February 2023;
Published: 09 March 2023.
Edited by:
Richard Graham Taylor, University College London, United KingdomReviewed by:
Si-Liang Li, Tianjin University, ChinaCopyright © 2023 Dordoni, Zappalà and Barth. This is an open-access article distributed under the terms of the Creative Commons Attribution License (CC BY). The use, distribution or reproduction in other forums is permitted, provided the original author(s) and the copyright owner(s) are credited and that the original publication in this journal is cited, in accordance with accepted academic practice. No use, distribution or reproduction is permitted which does not comply with these terms.
*Correspondence: Marlene Dordoni, bXZkb3Jkb25pQGdtYWlsLmNvbQ==
Disclaimer: All claims expressed in this article are solely those of the authors and do not necessarily represent those of their affiliated organizations, or those of the publisher, the editors and the reviewers. Any product that may be evaluated in this article or claim that may be made by its manufacturer is not guaranteed or endorsed by the publisher.
Research integrity at Frontiers
Learn more about the work of our research integrity team to safeguard the quality of each article we publish.