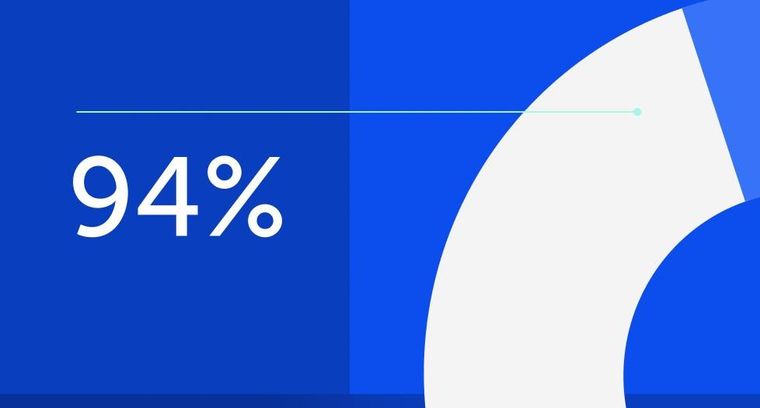
94% of researchers rate our articles as excellent or good
Learn more about the work of our research integrity team to safeguard the quality of each article we publish.
Find out more
METHODS article
Front. Water, 11 July 2022
Sec. Water and Hydrocomplexity
Volume 4 - 2022 | https://doi.org/10.3389/frwa.2022.925294
This article is part of the Research TopicAdvances and Emerging Methods in Tracer HydrogeologyView all 5 articles
Labeling groundwater by injecting an artificial tracer is a standard and widely used method to study groundwater flow systems. Noble gases dissolved in groundwater are potentially ideal artificial tracers, as they are not subject to biogeochemical transformations, do not adsorb onto the aquifer matrix, are colorless, and have no negative impact on the quality of groundwater resources. In addition, combining different noble-gas species in multi-tracer tests would allow direct analysis of the spatio-temporal heterogeneity of groundwater flow systems. However, while the handling of noble gases is safe and straightforward for injection into groundwater, conventional methods to analyse dissolved noble gases tend to be impractical for groundwater tracer tests. The sampling and subsequent lab-based analysis of dissolved noble gases are laborious, expensive and time intensive. Therefore, only researchers with access to specialized noble-gas labs have attempted such tracer tests. The recently developed gas-equilibrium membrane-inlet mass spectrometers (GE-MIMS) allow efficient on-site analysis of dissolved gases at high temporal resolution. The GE-MIMS instruments thereby eliminate most of the analytical and logistical constraints of conventional lab-based techniques and therefore provide new opportunities for groundwater tests using artificially injected gases. We used a GE-MIMS to systematically test the applicability of He, Kr, and Xe as artificial groundwater tracers. These gas species were injected into groundwater as Dirac-like pulses at three piezometers located at various locations upstream of a pumping well, where dissolved gas concentrations were continuously monitored with the GE-MIMS instrument. The groundwater travel times observed in these tracer tests ranged from a few hours to several weeks, and were consistent with the groundwater flow field at the experimental test site. Travel times determined from the noble gas tracer tests were also consistent with those obtained traditional dye tracers.
Labeling groundwater by injecting an artificial tracer is a standard and widely used method to study groundwater flow systems. Water flow and solute transport are studied by analysing the evolution of the tracer concentration at an observation point downstream of the tracer injection point. Commonly used tracer types used for groundwater tests are dissolved ions, heat, (biological) particles, stable, or radioactive isotopes, and fluorescent dyes. Also, dissolved gases are sporadically used as artificial tracers. An ideal tracer is conservative and is transported passively in the water without sorption on the aquifer matrix. For practical reasons, the tracer should be nontoxic, easily applied and quantified in the field, and its natural background concentrations must be much lower than those pertaining to the artificial injection. For reviews, see for example Davis et al. (1980, 1985), Solomon et al. (1998), Leibundgut and Seibert (2011), Maliva (2016), Brunner et al. (2017), Schilling et al. (2019), and Benischke (2021).
To study the spatio-temporal variability of the groundwater flow, different tracer species can be injected at different locations or at different points in time. Analysing the evolution of the specific tracer concentrations in groundwater allows disentangling of various features of the groundwater flow field. For example, injections of different fluorescent dyes can be combined, and their specific concentrations at the observation point(s) are analyzed on-site using a field fluorimeter, which can discriminate between different dye compounds based on their specific light-absorption spectra. However, dyes require careful handling, which can be challenging and restricting in field applications. Some dyes tend to adsorb onto the aquifer matrix or are subject to biogeochemical transformations in groundwater. The application of fluorescent dyes may also be subject to environmental regulations, and the colouration of the water may raise concerns with the general public or with consumers of the water resources (see reviews cited above).
Noble gases are not subject to biogeochemical transformations, are not expected to adsorb on the aquifer matrix, are colorless, and have no negative impact on the quality of groundwater resources. Once a gas species is dissolved in groundwater at atmospheric pressure, it tends to remain dissolved in water due to the hydrostatic overpressure. The natural background concentrations of noble gases in groundwater are usually very low (with the exception of air-derived Ar, or He accumulating in old groundwaters due to its natural underground production; see e.g., Kipfer et al., 2002). For the typical applications of groundwater tests, the noble gases He, Ne, Kr, and Xe are therefore, in principle, ideal artificial tracers (Davis et al., 1980, 1985; Solomon et al., 1998; Maliva, 2016). In addition, as with fluorescent dyes, the combined application of different noble-gas species is expected to provide information on the spatio-temporal dynamics of the investigated system.
However, while the handling of noble gases is safe and straightforward for groundwater injection, the conventional methods to analyse dissolved noble gases tend to be impractical for groundwater tracer tests. The sampling of dissolved noble gases is laborious, and the subsequent laboratory-based analysis techniques are expensive and time-consuming. Therefore, only few such groundwater tests have been attempted by researchers with access to specialized noble-gas laboratories (Carter et al., 1959; Gupta et al., 1994; Sanford et al., 1996; Ekwurzel et al., 1999; Clark et al., 2005; Visser et al., 2014). While these studies underlined the scientific potential of noble gases as artificial groundwater tracers, the analytical, and logistical constraints prevented their wide-spread use in applied groundwater research.
The recent development of mass spectrometers for on-site analysis of dissolved gases (Mächler et al., 2012; Brennwald et al., 2016; Chatton et al., 2017) provides new opportunities for groundwater tests using artificially injected gases. The newly developed gas-equilibrium membrane-inlet mass spectrometry (GE-MIMS) method allows automated and simultaneous quantification of different dissolved gas species with a time resolution of a few minutes per measurement (see for example Mächler et al., 2013; Tyroller et al., 2014; Brennwald et al., 2016, 2020; Weber et al., 2018; Knapp et al., 2019; Popp et al., 2020; Roques et al., 2020; Schilling et al., 2021). The portable and autonomous GE-MIMS systems allow efficient on-site analysis of the full noble-gas breakthrough curves at observation points, and may therefore eliminate the analytical and logistical constraints for the use of noble gases as artificial tracers in groundwater tests.
Here, we present field experiments of He, Kr, and Xe as artificial groundwater tracers when used in combination with a GE-MIMS instrument. The choice of these gas species was based on their low natural background and their analytical convenience. The tracer tests were carried out at a groundwater test site in northeastern Switzerland. The gases were injected into groundwater as Dirac-like pulses, whereby the different tracer species were injected at different piezometers to label separate groundwater flow lines. The concentrations of He, Kr, and Xe dissolved in groundwater were monitored using the GE-MIMS at a pumping well downstream of the piezometers. The travel time of the tracer gases from the injection points to the pumping well ranged from a few hours to weeks, depending on the prevailing hydraulic conditions and the distances of the injection points to the pumping well. Breakthrough curves of both the noble-gas and dye tracers at the pumping well were compared by simultaneous injection of both tracer types. Based on these tests, we discuss and assess the utility of noble gases as a new class of tracer for groundwater tests in applied research.
We examined the utility and practicability of noble gases as artificial tracers at the RHESI groundwater test site (www.rhesi.org) located near the Alpine Rhine channel in northeastern Switzerland. Due to long-term colmatation of the channelized river bed, the river exhibits a low hydraulic connection to the underlying aquifer. The RHESI project aims to study the interplay between river restoration measures and riverbed permeability dynamics related to riverbed erosion events. To test the response of groundwater in the layered aquifer system to such erosion events, the riverbed was excavated from 15 to 20 April 2021 along a 50m section of the Alpine Rhine (Figure 1). The (partial) removal of the colmation layer by this simulated erosion event resulted in a temporary increase in infiltration of river water into the aquifer; infiltration rates approached nominal conditions in the subsequent months through natural re-clogging of the riverbed. This test site provided an ideal setting to assess dissolved noble gases as tracers for groundwater tests.
Figure 1. Schematic map of the test site showing the locations of the piezometers used for tracer injection (A, B, C), the pumping well (P), a qualitative illustration of the assumed hydraulic head (black contour lines) and groundwater flow (gray lines with arrows) before riverbed excavation, the river (blue), and the approximate area of the riverbed excavation (E).
The gas tracers were injected into the groundwater at three different piezometers drilled into the aquifer (see Section 2.2), which consists of fluvial gravels and sands. The piezometers are located at different distances and along different flow lines upstream of a pumping well (Figure 1). The pump was operated at a constant production rate of 8.2 L/s, which resulted in a water-level drawdown of 10cm at the pumping well. The dissolved-gas concentrations in the pumped water were continuously monitored with the GE-MIMS instrument (see Section 2.3). Some selected gas injections were combined with simultaneous injections of fluorescent dyes to compare both sets of tracer breakthrough curves at the pumping well.
The gas tracers used to label the groundwater (He, Kr, Xe) were acquired as pure gases (>99% purity) stored at high pressure in steel tanks. The gases were injected as Dirac-like pulses into the groundwater using a gas diffuser (Figure 2). The diffuser was connected to the gas tanks using adjustable pressure reducers and long, flexible copper tubing (6 mm outer diameter), with which the diffuser could be lowered to the bottom of the injection piezometers. The gas bubbles emitted from the diffuser partially dissolve in the groundwater before reaching the water surface. To optimize the efficiency of bubble dissolution into the groundwater, the gas diffuser was made of a piece of fine-pored limewood, which produced a large number of very small gas bubbles that tend to rise slowly and provide a large specific surface for gas/water exchange. The gas bubbles were injected for 5min, with the pressure regulators adjusted for a low gas consumption of approximately 100 LSTP per injection. By default, He was injected at piezometer A, Kr at piezometer B, and Xe at piezometer C. In some tests, however, a sequence of combined injections using different tracer species was injected at the same piezometer (see Sections 3.1, 3.2), whereby each tracer pulse was allowed to transfer from the piezometer into the aquifer for at least 1 h without interference from the other tracer injections. After completion of each of the gas injections, the piezometer headspace was purged with N2 from a pressurized gas tank or with ambient air (using a pump) in order to avoid any potential for re-dissolution of residual tracer gases into the water flowing through the piezometer.
The gas bubbles injected into the piezometer might get trapped inside or behind the screen of the piezometer. Such trapped tracer gas bubbles would dissolve slowly in the groundwater, possibly resulting in a smearing and tailing of the breakthrough peak observed at the pumping well. Since He exhibits the lowest solubility from the three tracer gases, the He bubbles would dissolve slowest, whereas the Xe bubbles would dissolve fastest (Holocher et al., 2003). The potential smearing and tailing of the gas breakthrough peaks would therefore be expected to be strongest for He, less for Kr, and least for Xe. To assess the importance of such artifacts, we compared the bubble-diffuser method with an injection of bubble-free water that contained a spike of dissolved He, Kr, and Xe tracers (see also Davis et al., 1985).
The tracer gases dissolved in the water pumped from the well were continuously quantified with a time resolution of approximately 5 min using a miniRUEDI portable mass spectrometer (Brennwald et al., 2016, Gasometrix GmbH, Switzerland). The pumped water was fed through a membrane module, where a gas/water equilibrium with a small gas headspace was established according to Henry's Law. This gas headspace was connected to the gas inlet of the miniRUEDI analyser to “sniff” the partial pressures of the gas species in the pumped water (GE-MIMS, Brennwald et al., 2016).
He, Kr, and Xe were quantified using the mass spectrometer from their ion-current intensities at m/z = 4, 84, and 129, respectively. While Ne would also be expected to be a suitable groundwater tracer, its quantification is complicated by mass-spectrometric interferences at m/z = 20 resulting from residual Ar and isotopically heavy water in the mass spectrometer. While reliable Ne quantification is technically feasible, the procedures to control the H2O interference require long dead-times for analysis of all other gases (Brennwald et al., 2020). To optimize the data quality for the He, Kr, and Xe measurements, we therefore did not analyse Ne in our tests.
The raw ion-current data obtained from the mass spectrometer were compensated for instrumental drift with hourly measurements of air used a standard gas. This air standard was stored in a gas-tight bag (Cali-5-Bond, Calibrated Instruments) in order to avoid the potential for contamination with tracer gases released to the atmosphere during gas injection at the piezometers.
The noble-gas tracer methods were tested by 41 gas injections at the RHESI groundwater test site over the course of one year. Figure 3 shows an example of the He, Kr, and Xe tracer breakthrough curves observed at the pumping well in response to pulse injections at piezometers A, B, and C. The breakthrough curves show the typical delay and smearing of the Dirac-like injection due to groundwater advection, dispersion, and potentially also matrix diffusion and similar processes (see Section 4.1). The He injected at piezometer A appears at the pumping well first (peak maximum at 6.2 h after injection), followed by Xe from piezometer C (20.7 h) and Kr from piezometer B (192 h). This sequence of the tracer peaks is consistent with the groundwater flow system as illustrated in Figure 1. The asymmetric shape of the breakthrough peaks results from the progressing dispersive smearing of the peak as the breakthrough curve evolves at the pumping well (Leibundgut and Seibert, 2011), and is further enhanced by the convergent flow to the pumping well (Moench, 1989; Pedretti and Fiori, 2013; Pedretti et al., 2013).
Figure 3. Examples of breakthrough curves at the pumping well after injection of He, Kr, and Xe into the groundwater at piezometers A, B, and C using the bubble diffuser on 2021-09-07. Note the logarithmic scaling of the time axis.
Figure 4A shows a comparison of the breakthrough curves resulting from combined injections of He, Kr, and Xe using the bubble-diffuser method at piezometer C. The maxima of the breakthrough peaks occur at 15.4 h (Xe), 16.6 h (Kr), and 18.7 h (He). Overall, Xe shows the sharpest peak with the steepest slopes both before and after the peak maximum, whereas the He peak tends to show the most smearing and tailing. The shape of the Kr peak falls between those of He and Xe. The variation of the He, Kr, and Xe travel times (occurrence of the peak maxima) corresponds to a standard deviation of approximately 10% relative to their mean.
Figure 4. Comparison of the breakthrough curves of combined He, Kr, and Xe injections at piezometer C. (A) Gas injection using the down-hole bubble diffuser on 2022-03-03. (B) Injection of dissolved gas spike in bubble-free water on 2022-03-29.
Figure 4B shows a comparison of the breakthrough curves resulting from the injection test using bubble-free water containing a spike of dissolved He, Kr, and Xe at piezometer C. The breakthrough peaks of all three tracer gases are virtually identical, whereby a small delay of the He peak (16.9 h travel time) relative to the Kr and Xe peaks (15.5 h travel times) is observed.
Figure 5 shows a comparison of breakthrough curves from combined injections of noble gases and fluroescent tracers. He and sulforhodamine-B were injected at piezometer A, Xe, and uranine at piezometer C. The breakthrough curves of the noble gases and the fluorescent tracers are very similar during the phase of the increase of the tracer concentrations, and the peak maxima of the tracers injected at the same piezometer occur at approximately the same time. The initial phase of the concentration decrease immediately after the peak maxima is also similar for the noble gases and dye tracers, but at a later phase the peak tails tend to diverge. For piezometer A, the He peak shows less tailing than the sulforhodamine-B peak, whereas for piezometer C the Xe peak shows more tailing than the uranine peak.
Figure 5. Breakthrough curves from combined injections of noble gases and fluorescent dyes (He and sulforhodamine-B at piezometer A, Xe, and uranine at piezometer C).
Table 1 lists the injection tests that were carried out to study the variations of the tracer breakthrough peaks in relation to the riverbed excavation experiment. The “travel times” listed in the table correspond to times that elapsed between the tracer injection and the occurrence of the maximum of the breakthrough peak at the pumping well.
Table 1. List of the noble gas tracer injections at piezometers A, B, and C and travel times to the pumping well.
Before the excavation of the riverbed, the repeated injections in March 2021 resulted in travel times of 7.3 h to 7.4 h from piezometer A, 86 h from piezometer B, and 12.6 h to 12.9 h from piezometer C. Immediately after the excavation of the riverbed in mid-April 2021, the travel times from piezometer A decreased to 5.3 h or less with the injection tests carried during April–June; the tracers injected at piezometers B and C during this time were not observed at the pumping well. With the later injections carried out from September 2021 to March 2022, the travel times from piezometer A ranged from 6.2 h to 6.9 h, and the Kr and Xe tracers were observed again at the pumping well with travel times of 168 h to 334 h from piezometer B and 15.4 h to 20.7 h from piezometer C.
The breakthrough curves observed from simultaneous injections of He, Kr, and Xe using the bubble-diffuser at piezometer C show slight yet systematic differences (Section 3.1). Xe shows the sharpest breakthrough peak with the lowest travel time, whereas the He breakthrough peak exhibits the strongest smearing and a slightly higher travel time. The Kr breakthrough curve falls in between those of He and Xe (Figure 4A). This systematic, gas-specific smearing and tailing of the breakthrough peaks is not apparent with the test injection using bubble-free water and is therefore attributed to the injection of tracer-gas bubbles into the piezometer. The trapping of gas bubbles in the piezometer screen may indeed cause a certain inconsistency between the breakthrough curves of He, Kr, and Xe. Nevertheless, even if the tracer-gas bubbles do get trapped in the piezometer screen, the travel times determined from the three gas species were found to be consistent within approximately 10%. Since the use of the downhole bubble-diffuser is very simple during field work, we therefore used the diffuser-method for all further tests and experiments within the scope of this work.
Note that the He breakthrough of the bubble-free tracer injection still shows a small delay relative to the Kr and Xe peaks. This delay must be due to a He-specific retardation process during transport of the dissolved tracers in the groundwater, possibly because He is much smaller and more mobile than Kr and Xe. For example, if a tracer-gas pulse in the groundwater flow passes near a lens of stagnant water, the dissolved gases will be exchanged between the flowing and the stagnant water by molecular diffusion. This “matrix diffusion” process would result in a retardation of the tracer breakthrough curve (Małoszewski and Zuber, 1985; Sanford and Solomon, 1998; Jardine et al., 1999; Małoszewski et al., 1999; Hauns et al., 2001; McNeill et al., 2001; Liedl and Ptak, 2003; Rezanezhad et al., 2012). Since the rate of molecular diffusion of He is 4.5 times higher than that of Kr and 5.6 times higher than that of Xe (Jähne et al., 1987), the corresponding retardation is expected to be systematically higher for He than for Kr and Xe.
The breakthrough curves observed from simultaneous injections of noble gases and fluorescent dyes (Section 3.2 and Figure 5) show very similar behavior for both sets of tracers until the onset of the concentration decrease after the peak maxima, with similar travel times between the two tracer types. However, the different behaviors of the breakthrough tailing hint at slightly different behaviors of the different tracer species.
In case of the simultaneous injection of He and sulforhodamine-B at piezometer A, the stronger tailing of the fluorescent dye is attributed to its tendency to adsorb on the aquifer matrix (Leibundgut and Seibert, 2011). The elution of the adsorbed sulforhodamine-B results in the extended tailing of the dye breakthrough peak. The opposite behavior is observed in case of the simultaneous injection of Xe and uranine in piezometer C, where the tailing is larger for the noble gas tracer. Adsorption is unlikely to play a role here, as uranine has a low tendency to adsorb on aquifer materials (Leibundgut and Seibert, 2011) and noble gases are generally considered to be non-sorbing in groundwater systems. The enhanced tailing of the Xe breakthrough peak may be related to delayed dissolution of trapped Xe gas bubbles after injection in the piezometer (Section 4.1).
The observed noble-gas travel times from piezometers A, B, and C to the pumping well show a systematic evolution in response to variations of groundwater-flow dynamics during the riverbed excavation experiment (Section 3.3 and Table 1). Before excavation of the riverbed, all three piezometers A, B, and C were considered to exhibit a groundwater-flow connection to the pumping well. Correspondingly, the He, Kr, and Xe pulses injected at these piezometers in March 2021 were observed at the pumping well. Immediately after the excavation of the riverbed, the groundwater flow toward the pumping well was focused near piezometer A, with poor flow connections between the pumping well and piezometers B and C. This is reflected in the results from noble gas the injections from April to June. The He travel times from piezometer A to the pumping well showed a marked decrease of approximately 35% relative to the pre-excavation value, whereas no significant amounts of Kr and Xe injected at piezometers B and C reached the pumping well. In a later phase, the groundwater flow-field is expected to gradually return to pre-excavation conditions due to the progressive re-clogging of the riverbed. Accordingly, travel times from piezometer A to the pumping well observed with the He injections from September 2021 to March 2022 lie between those immediately after the excavation and the pre-excavation values. Also, the injections of Kr at piezometer B and Xe at piezometer C were once again detected at the pumping well, albeit with travel times above the pre-excavation values.
Overall, the observed behavior of the He, Kr, and Xe tracers injected at piezometers A, B, and C are well in-line with the excavation-controlled changes in the groundwater flow system at the test site. The noble-gas tracer tests correctly reproduce the expected spatial characteristics of the groundwater flow field and its temporal evolution throughout the entire course of the riverbed excavation experiment.
The systematic tests conducted for this work demonstrate that noble gases are indeed suitable as artificial tracers to study groundwater flow systems. Analogous to other widely-used artificial tracers, the delay of a noble-gas breakthrough peak relative to a Dirac-like gas injection reflects groundwater travel time, whereas the smearing of the breakthrough peak is controlled by groundwater diffusion and dispersion. The travel times of the different gas species within the groundwater system were consistent with those determined from fluorescent dye tracers, and were found to be in line with the evolution of the expected groundwater flow field at our test site.
Our simple technique for gas injection and high-resolution analysis of He, Kr, and Xe breakthrough curves proved to be practical and efficient. In contrast to earlier attempts of using noble gases as artificial tracers, the automated on-site analysis of dissolved gas tracers using a GE-MIMS instrument substantially reduced the logistics and effort for field work. Furthermore, the GE-MIMS avoids the laborious water sampling and subsequent time-consuming analysis in a specialized noble-gas laboratory
For the purposes of our field tests, we injected the noble gases into the groundwater at piezometers using a downhole bubble-diffuser. This simple method to label the groundwater with dissolved gases is straight-forward to apply during field work. However, depending on the construction of the piezometer, gas bubbles may get trapped in the screen of the piezometer. The delayed dissolution of these trapped bubbles results in a smearing and tailing of the breakthrough peaks, but had limited effect on the derived travel times. In addition, the escape of gas bubbles at the water surface in the piezometer results in incomplete dissolution of the gas volume injected via the diffuser. Tracer recovery rates were therefore not evaluated, and noble-gas concentrations observed at the pumping well were not used to quantify groundwater mixing ratios or dilution factors. To avoid the trapping of gas bubbles in the piezometer screen and to allow quantitative Dirac-like gas injections, bubble-free injection methods can be used. For example, predefined amounts of gas-labeled water with known tracer concentrations could be injected into the aquifer (e.g., Davis et al., 1985), or membrane gas/water contactors for bubble-free dissolution of controlled amounts of tracer gases into the groundwater could be used (Sanford et al., 1996; Sanford and Solomon, 1998; Solomon et al., 1998; Peel et al., 2021). The shapes of the breakthrough curves resulting from bubble-free injections are also expected to allow further analyses of site-specific transport processes and parameters by fitting analytical or numerical groundwater models to the observed breakthrough curves (e.g. Leibundgut and Seibert, 2011; Molinari et al., 2015).
The simultaneous analysis of multiple gas species with the GE-MIMS proved highly beneficial to study the hydrogeological dynamics. In combination with the analytical simplicity of the autonomous miniRUEDI gas analyser, simultaneous injections of He, Kr, and Xe at different locations were shown to provide an efficient tool for application in future studies assessing the spatial characteristics of groundwater flow systems. We also demonstrated how the temporal evolution of a groundwater flow system can be probed through repeated injections of different gas tracers at strategic locations within an aquifer.
While noble gases have previously been proposed as artificial tracers for groundwater tests, our systematic field experiments corroborate the scientific potential of noble gases as ideal artificial conservative tracers. This potential can now be exploited using the recently developed GE-MIMS instruments, which allow continuous and autonomous on-site analysis of multiple noble-gas tracer species dissolved not only in groundwater, but also in other aquatic environments such as rivers, lakes or oceans. These new analytical tools therefore allow noble gases to be used as safe, convenient and practical tracers in aquatic systems.
The original contributions presented in the study are included in the article/supplementary material, further inquiries can be directed to the corresponding author/s.
MB: conceptualization, field work, data processing, and text. MP and DH: conceptualization, field work, and data processing. TB and YT: field work. RK and PB: conceptualization. All authors contributed to the article and approved the submitted version.
This project was supported by Swiss National Science Foundation (Project number 200021_179017). Open access funding provided by Eawag—Swiss Federal Institute of Aquatic Science and Technology.
The authors declare that the research was conducted in the absence of any commercial or financial relationships that could be construed as a potential conflict of interest.
All claims expressed in this article are solely those of the authors and do not necessarily represent those of their affiliated organizations, or those of the publisher, the editors and the reviewers. Any product that may be evaluated in this article, or claim that may be made by its manufacturer, is not guaranteed or endorsed by the publisher.
We are grateful to the Internationale Rheinregulierung (Austria and Switzerland), who provided us full access to their groundwater test site and infrastructure of the RHESI project. We thank Sébastien Giroud, Alexandra Lightfoot, Pascal Rünzi, and Capucine Marion for their help with the fieldwork.
Benischke, R. (2021). Review: advances in the methodology and application of tracing in karst aquifers. Hydrogeol. J. 29, 67–88. doi: 10.1007/s10040-020-02278-9
Brennwald, M. S., Schmidt, M., Oser, J., and Kipfer, R. (2016). A portable and autonomous mass spectrometric system for on-site environmental gas analysis. Environ. Sci. Technol. 50, 13455–13463. doi: 10.1021/acs.est.6b03669
Brennwald, M. S., Tomonaga, Y., and Kipfer, R. (2020). Deconvolution and compensation of mass spectrometric overlap interferences with the miniRUEDI portable mass spectrometer. MethodsX 7:101038. doi: 10.1016/j.mex.2020.101038
Brunner, P., Therrien, R., Renard, P., Simmons, C. T., and Franssen, H.-J. H. (2017). Advances in understanding river-groundwater interactions. Rev. Geophys. 55, 818–854. doi: 10.1002/2017RG000556
Carter, R. C., Kaufman, W. J., Orlob, G. T., and Todd, D. K. (1959). He as a ground-water tracer. J. Geophys. Res. 64, 2433–2439. doi: 10.1029/JZ064i012p02433
Chatton, E., Labasque, T., de La Bernardie, J., Guihéneuf, N., Bour, O., and Aquilina, L. (2017). Field continuous measurement of dissolved gases with a CF-MIMS: applications to the physics and biogeochemistry of groundwater flow. Environ. Sci. Technol. 51, 846–854. doi: 10.1021/acs.est.6b03706
Clark, J. F., Hudson, G. B., and Avisar, D. (2005). Gas transport below artificial recharge ponds: insights from dissolved noble gases and a dual gas (SF6 and 3He) tracer experiment. Environ. Sci. Technol. 39, 3939–3945. doi: 10.1021/es049053x
Davis, S. N., Campbell, D. J., Bentley, H. W., and Flynn, T. J. (1985). An Introduction to Ground-Water Tracers. Technical Report EPA/600/2-85/022, University of Arizona.
Davis, S. N., Thompson, G. M., Bentley, H. W., and Stiles, G. (1980). Ground-water tracers-a short review. Groundwater 18, 14–23. doi: 10.1111/j.1745-6584.1980.tb03366.x
Ekwurzel, B., Hudson, G., Moran, J., and Davisson, M. (1999). “Enriched noble gas isotopes: ideal tracers for groundwater recharge,” in International Symposium on Isotope Techniques in Water Resources Development and Management, IAEA-SM-361 (Vienna), 285–286.
Gupta, S. K., Lau, L. S., and Moravcik, P. S. (1994). Ground-water tracing with injected helium. Ground Water 32, 96–102. doi: 10.1111/j.1745-6584.1994.tb00616.x
Hauns, M., Jeannin, P.-Y., and Atteia, O. (2001). Dispersion, retardation and scale effect in tracer breakthrough curves in karst conduits. J. Hydrol. 241, 177–193. doi: 10.1016/S0022-1694(00)00366-8
Holocher, J., Peeters, F., Aeschbach-Hertig, W., Kinzelbach, W., and Kipfer, R. (2003). Kinetic model of gas bubble dissolution in groundwater and its implications for the dissolved gas composition. Environ. Sci. Technol. 26, 1337–1343. doi: 10.1021/es025712z
Jähne, B., Heinz, G., and Dietrich, W. (1987). Measurement of the diffusion coefficients of sparingly soluble gases in water. J. Geophys. Res. 92, 10767–10776. doi: 10.1029/JC092iC10p10767
Jardine, P. M., Sanford, W. E., Gwo, J. P., Reedy, O. C., Hicks, D. S., Riggs, J. S., et al. (1999). Quantifying diffusive mass transfer in fractured shale bedrock. Water Resour. Res. 35, 2015–2030. doi: 10.1029/1999WR900043
Kipfer, R., Aeschbach-Hertig, W., Peeters, F., and Stute, M. (2002). “Noble gases in lakes and ground waters,” in Noble Gases in Geochemistry and Cosmochemistry, Vol. 47 of Rev. Mineral. Geochem., eds D. Porcelli, C. J. Ballentine, and R. Wieler (Mineralogical Society of America, Geochemical Society), 615–700. doi: 10.2138/rmg.2002.47.14
Knapp, J., Osenbrück, K., Brennwald, M. S., and Cirpka, O. A. (2019). In-situ mass spectrometry improves the estimation of stream reaeration from gas-tracer tests. Sci. Tot. Environ. 655, 1062–1070. doi: 10.1016/j.scitotenv.2018.11.300
Leibundgut, C., and Seibert, J. (2011). “Chapter 2.09: Tracer hydrology,” in Treatise on Water Science, ed P. Wilderer (Oxford: Elsevier), 215–236. doi: 10.1016/B978-0-444-53199-5.00036-1
Liedl, R., and Ptak, T. (2003). Modelling of diffusion-limited retardation of contaminants in hydraulically and lithologically nonuniform media. J. Contam. Hydrol. 66, 239–259. doi: 10.1016/S0169-7722(03)00028-7
Mächler, L., Brennwald, M. S., and Kipfer, R. (2012). Membrane inlet mass spectrometer for the quasi-continuous on-site analysis of dissolved gases in groundwater. Environ. Sci. Technol. 46, 8288–8296. doi: 10.1021/es3004409
Mächler, L., Brennwald, M. S., and Kipfer, R. (2013). Argon concentration time-series as a tool to study gas dynamics in the hyporheic zone. Environ. Sci. Technol. 47, 7060–7066. doi: 10.1021/es305309b
Maliva, R. G. (2016). “Chapter 13: Tracer tests,” in Aquifer Characterization Techniques, ed Maliva (Springer), 403–444. doi: 10.1007/978-3-319-32137-0_13
Małoszewski, P., Herrmann, A., and Zuber, A. (1999). Interpretation of tracer tests performed in fractured rock of the Lange Bramke Basin, Germany. Hydrogeol. J. 7, 208–218. doi: 10.1007/s100400050193
Małoszewski, P., and Zuber, A. (1985). On the theory of tracer experiments in fissured rocks with a porous matrix. J. Hydrol. 79, 333–358. doi: 10.1016/0022-1694(85)90064-2
McNeill, G. W., Yang, Y., T, E., and B, K. (2001). “Krypton gas as a novel applied tracer of groundwater flow in a fissured sandstone aquifer,” in New Approaches to Characterizing Groundwater Flow, eds K. P. Seiler, S. Wohnlich (Lisse: AA Balkema), 143–148.
Moench, A. F. (1989). Convergent radial dispersion: a Laplace transform solution for aquifer tracer testing. Water Resour. Res. 25, 439–447. doi: 10.1029/WR025i003p00439
Molinari, A., Pedretti, D., and Fallico, C. (2015). Analysis of convergent flow tracer tests in a heterogeneous sandy box with connected gravel channels. Water Resour. Res. 51, 5640–5657. doi: 10.1002/2014WR016216
Pedretti, D., Fernàndez-Garcia, D., Bolster, D., and Sanchez-Vila, X. (2013). On the formation of breakthrough curves tailing during convergent flow tracer tests in three-dimensional heterogeneous aquifers. Water Resour. Res. 49, 4157–4173. doi: 10.1002/wrcr.20330
Pedretti, D., and Fiori, A. (2013). Travel time distributions under convergent radial flow in heterogeneous formations: insight from the analytical solution of a stratified model. Water Resour. 60, 100–109. doi: 10.1016/j.advwatres.2013.07.013
Peel, M., Blanc, T., Brennwald, M., Brunner, P., and Kipfer, R. (2021). “Using helium as artificial tracer to characterize surface water-groundwater interactions,” in Goldschmidt Conference. doi: 10.7185/gold2021.7866
Popp, A., Manning, C., Brennwald, M. S., and Kipfer, R. (2020). A new in situ method for tracing denitrification in riparian groundwater. Environ. Sci. Technol. 54, 1562–1572. doi: 10.1021/acs.est.9b05393
Rezanezhad, F., Price, J. S., and Craig, J. R. (2012). The effects of dual porosity on transport and retardation in peat: a laboratory experiment. Can. J. Soil Sci. 92, 723–732. doi: 10.4141/cjss2011-050
Roques, C., Weber, U. W., Brixel, B., Krietsch, H., Dutler, N., Brennwald, M. S., et al. (2020). In situ observation of helium and argon release during fluid-pressure-triggered rock deformation. Sci. Rep. 10. doi: 10.1038/s41598-020-63458-x
Sanford, W. E., Shropshire, R. G., and Solomon, D. K. (1996). Dissolved gas tracers in groundwater: Simplified injection, sampling and analysis. Water Resour. Res. 32, 1635–1642. doi: 10.1029/96WR00599
Sanford, W. E., and Solomon, D. K. (1998). Site characterization and containment assessment with dissolved gases. J. Environ. Eng. 124, 572–574. doi: 10.1061/(ASCE)0733-9372(1998)124:6(572)
Schilling, O., Parajuli, A., Tremblay, O. C., Müller, T., Antolinez, Q. W., Tremblay, Y., et al. (2021). Quantifying groundwater recharge dynamics and unsaturated zone processes in snow dominated catchments via on site dissolved gas analysis. Water Resour. Res. 57:e2020WR028479. doi: 10.1029/2020WR028479
Schilling, O. S., Cook, P. G., and Brunner, P. (2019). Beyond classical observations in hydrogeology: the advantages of including exchange flux, temperature, tracer concentration, residence time, and soil moisture observations in groundwater model calibration. Rev. Geophys. 57, 146–182. doi: 10.1029/2018RG000619
Solomon, D. K., Cook, P. G., and Sanford, W. E. (1998). “Chapter 9: Dissolved gases in subsurface hydrology,” in Isotope Tracers in Catchment Hydrology, eds C. Kendall and J. J. McDonnel (Oxford: Elsevier), 291–318. doi: 10.1016/B978-0-444-81546-0.50016-1
Tyroller, L., Brennwald, M. S., Mächler, L., Livingstone, D. M., and Kipfer, R. (2014). Fractionation of Ne and Ar isotopes by molecular diffusion in water. Geochim. Cosmochim. Acta 136, 60–66. doi: 10.1016/j.gca.2014.03.040
Visser, A., Singleton, M., and Esser, B. (2014). Xenon Tracer Test at Woodland Aquifer Storage and Recovery Well. Technical report, Lawrence Livermore National Lab, Livermore, CA. doi: 10.2172/1162248
Keywords: hydrogeology, recharge, groundwater test, travel time, dating, flow line
Citation: Brennwald MS, Peel M, Blanc T, Tomonaga Y, Kipfer R, Brunner P and Hunkeler D (2022) New Experimental Tools to Use Noble Gases as Artificial Tracers for Groundwater Flow. Front. Water 4:925294. doi: 10.3389/frwa.2022.925294
Received: 21 April 2022; Accepted: 16 June 2022;
Published: 11 July 2022.
Edited by:
Yuji Sano, Kōchi University, JapanReviewed by:
Daniele Pedretti, University of Milan, ItalyCopyright © 2022 Brennwald, Peel, Blanc, Tomonaga, Kipfer, Brunner and Hunkeler. This is an open-access article distributed under the terms of the Creative Commons Attribution License (CC BY). The use, distribution or reproduction in other forums is permitted, provided the original author(s) and the copyright owner(s) are credited and that the original publication in this journal is cited, in accordance with accepted academic practice. No use, distribution or reproduction is permitted which does not comply with these terms.
*Correspondence: Matthias S. Brennwald, bWF0dGhpYXMuYnJlbm53YWxkQGVhd2FnLmNo
Disclaimer: All claims expressed in this article are solely those of the authors and do not necessarily represent those of their affiliated organizations, or those of the publisher, the editors and the reviewers. Any product that may be evaluated in this article or claim that may be made by its manufacturer is not guaranteed or endorsed by the publisher.
Research integrity at Frontiers
Learn more about the work of our research integrity team to safeguard the quality of each article we publish.