- 1International Water Management Institute, East Africa and Nile Basin Office, Addis Ababa, Ethiopia
- 2International Water Management Institute, Battaramulla, Sri Lanka
The sustainable agricultural intensification (SAI) debate, partly rooted in discussions over the Green Revolution, was developed in the 1990s in the context of smallholder agriculture in Africa. In many Sub-Saharan African (SSA) countries, production is still largely rainfed, with the prevalence of significant yield gaps and rapid environmental degradation. Projections indicate that climate and demographic changes will further intensify the competition for freshwater resources. Currently, SAI is centered around predominantly rain-fed agricultural systems, often at a farm and plot scales. There has been increased attention to the improved role of agricultural water management (AWM) to address the daunting challenges of climate change, land degradation and food and nutritional insecurity in SSA. Nonetheless, the supporting frameworks and tools remain limited and do not connect the sustainability assessment and the development of intensification pathways (SIP) along multiple scales of the rainfed irrigation continuum. This paper reviews the gaps in concepts and practices of SAI and suggests a methodological framework to design context-specific and water-centered SIP for the SSA region. Accordingly, the proposed methodological framework demonstrates: (a) how to couple sustainability assessment methods to participatory SIPs design and adaptive management approach; (b) how contextualized sustainability domains and indicators can help in AWM centered SIP development; (c) the approaches to handle multiple scales and water-related indicators, the heterogeneity of biophysical and social settings when tailoring technology options to local contexts; and (d) the principles which enable the SIP designs to enable synergies and complementarities of SAI measures to reinforce the rainfed-irrigation continuum. This methodological framework allows researchers to integrate the sustainability assessment and SIP design, and guides policymakers and practitioners in planning, implementing and monitoring SAI initiatives (e.g., Framework for Irrigation Development and Agricultural Water Management in Africa) across multiple scales.
Introduction
The world's population is expected to grow to 10 billion by 2050, in turn increasing the demand for agricultural products by about 50% as compared to 2013, in a scenario of modest economic growth (FAO, 2017). At the same time, in Sub-Saharan Africa (SSA), the projected population growth is higher, leading to intensified pressure on already scarce agricultural land and water resources (Conway et al., 2013). Worldwide, about 80% of the cropped area is dependent on rain-fed agriculture—this figure rises to almost 90% for the SSA region (You et al., 2011). Rain-fed agriculture is vulnerable to increasing climate variability leading to significant yield loss (AU, 2020). In the face of increasingly variable and uncertain rainfall, long dry seasons, recurrent droughts, and dry spells as well as floods, water is a key yield-limiting factor for rain-fed agricultural production systems, particularly in tropical semiarid and arid regions (Gleick, 2003; You et al., 2011; Conway et al., 2013; Nakawuka et al., 2018). In SSA the present yield level is only 24% of the potential yield (AU, 2020). Notably, an emergent major challenge is the production of 50% more produce under rain-fed dominated global agricultural systems (FAO, 2017; AU, 2020). The situation can be severe for SSA where the dominant system is rain-fed agriculture, with irrigation undertaken of only 6% of the total arable land (You et al., 2011; Conway et al., 2013). A rapidly increasing population (with a growth rate of 3%) in tandem with lagging agricultural growth has led to rising food imports, expected to swell from US$ 35 billion in 2015 to over US$ 110 billion by 2025. The import of agricultural products already accounts for about 15% of total African imports (AU, 2020).
Sustainable agricultural intensification (SAI) has been proposed (Conway et al., 2013; Steiner et al., 2020) as one option to address the increasing demand for agricultural production in SSA (AU, 2013). However, despite its merits—i.e., as illustrated by Pretty et al. (2011)—various forms of land degradation threaten the uptake of SAI. For example, Barrett (2008) and Sutton et al. (2013) have highlighted the linkages between excessive or otherwise inappropriate use of agrochemicals and environmental problems in many SSA areas (also note Assegide et al., 2022; Teklu et al., 2022); on the other hand, nutrient deficiencies and insufficient fertilizer application prevent productivity increases (Conway et al., 2013; Nakawuka et al., 2018). For example, in SSA (where subsistence agriculture dominates), continuous cultivation without adequate replenishment of soil nutrients leads to widespread soil nutrient mining, rendering people in poverty (Haileslassie et al., 2007). Many grazing lands are in a degraded state, affecting livestock productivity, household incomes, and environmental health (Haileslassie et al., 2007; Conway et al., 2013; Kemp et al., 2013). With the additional water resources required to sustain and increase production, there is a trade-off between the resulting agricultural benefits and the benefits foregone with alternative uses of the water (Cofie and Amede, 2015). These conditions are only prone to be further complicated in the face of climate change in SSA (AU, 2020). Overall, in many aspects, the planetary (e.g., biogeochemical flows) and local and regional boundaries (e.g., freshwater uses, land use changes) have been transgressed, with heightened vulnerability of agriculture and food systems to disturbances (Struik and Kuyper, 2017). In fact, recent studies (Steiner et al., 2020) indicate that in the worst-case scenario, the current food system can even collapse.
The commonly discussed priorities for SAI include increasing production on existing cropland by closing yield gaps (Steiner et al., 2020); the same is underscored by Conway et al. (2013), but with the addition of water for SSA in the definition. For achieving the intensification of agriculture, the increased and prudent use of water to close the yield gaps is vital (Cofie and Amede, 2015; van Ittersum et al., 2016; Nakawuka et al., 2018; AU, 2020). It entails improved agricultural water use efficiency (AU, 2013), diversifying and increasing the number of crops grown per year as well as a reduction in pre- and post-harvest losses. For livestock, the challenge is to increase productivity and the productive use of water per animal through better feeding (water productive feeds), effective animal breeding, and livestock health care, and a shift to more efficient animals—such as from cattle to poultry and fish or small ruminants—wherever possible.
However, a recent review by Haileslassie et al. (2020a,b) contends that the intensification of agricultural systems leads to the altering of hydrological processes, where multiple water values can be influenced (AU, 2020). SAI practices centered around agricultural water management (AWM), as posited by Cofie and Amede (2015) and AU (2020), provide an opportunity to address the current challenges and achieve food and nutritional security while improving upon or maintaining environmental sustainability (also note Rosegrant et al., 2013; MacDonald et al., 2016). Despite the differing opinions on SSA solutions given the diverse landscape, socio-economic settings, and technologies to pursue, a consensus is emerging that AWM-centered SAI needs to be built on context-specific sustainable intensification pathways (SIPs) (Godfray et al., 2010; Haileslassie et al., 2011, 2016; Conway et al., 2013; MacDonald et al., 2016; Nakawuka et al., 2018).
The current intensification practices and SIP design in the SSA region rarely include AWM in addressing challenges, ameliorating agricultural productivity and enhancing environmental integrity (Conway et al., 2013; Cofie and Amede, 2015). This lack of consideration is worrisome with the increasingly apparent impacts of climate change which affect both the demand and supply sides of freshwater resources as well as the size of arable land (Conway et al., 2013; Mutambara et al., 2016). Furthermore, rigorous efforts of trade-off management through targeting strategic entry points that leverage complementarity and synergies of interventions impacting the same water resources are typically deficient. Practical efforts to link sustainable intensification to landscape and food system (FS), along with targeted transformation from a piecemeal approach to one with an integration of genetic, environmental, and socio-economic practices, are in shortfall (Conway et al., 2013; Struik and Kuyper, 2017; AU, 2020).
The development of SIPs (Struik and Kuyper, 2017) requires the engagement of all stakeholders, an understanding of the state of the system, knowledge and technologies, the farmers' choice and vision, and a consideration of multiple models (Conway et al., 2013). In addition, a vital aspect is the recognition that farms of different sizes and systems can coexist in any location, use water in different magnitudes, and that more differentiation over time is driven by the interaction of demographic and economic change and political ambition (Masters et al., 2013; Struik and Kuyper, 2017). To ensure SIPs, for instance, new business models are essential for defining diverse options of agricultural technology to fit all farms at different scales and levels of resource endowment. The overarching objective of this study is to strengthen the current SAI planning process and practices by incorporating a systemic water lens. We first examine and synthesize the water-related gaps in science and practices of SAI by focusing on SSA; secondly, a methodological framework is developed to analyze the status of SAI to design sustainable water-centered and context-specific SIPs across multiple scales in SSA.
Methodology
In this study a narrative review type has been undertaken with a qualitative interpretation of prior knowledge. It has been innovatively synthesized to a methodological framework to assess the status quo of SAI measures and develop water centric SIPs. The published scientific literature is indexed in a variety of databases. To identify relevant literature from the same, we developed a search strategy, mainly in three databases—Scopus, Google and Google Scholar and Web of Sciences. Application of key words—sustainable agricultural intensification, sustainable agricultural intensification in Africa, intensification pathways, climate change impacts on water, irrigation in Africa, agricultural water management in Africa, rain fed irrigation continuum, food systems in Africa, water centric planning, agricultural intensification framework—was undertaken.
The search period was set to 2000–2022 and after a brief review of abstracts, 81 pieces of literature was screened as a priority. In many aspects, the literature was of international context; some was specific to SSA, either at the country or at the regional level. With the aim of comprehensive lessons, global, regional as well as country level examples were considered.
Figure 1 depicts the review framework deployed in this study, comprised of four interactive steps. It synthesizes the steps adopted and the key knowledge inputs in each step. The first step establishes the foundation of gaps assessment by exploring sustainable agricultural intensification practices globally and in SSA. Subsequently, SIP is defined and justified in the context of food system and water centric planning, specifically discussing the need for water centric pathways. The third step builds upon the previous ones to conceptualize and elaborate the SIPs. The final step encompasses stakeholder engagement to help validate the framework and obtain relevant feedback.
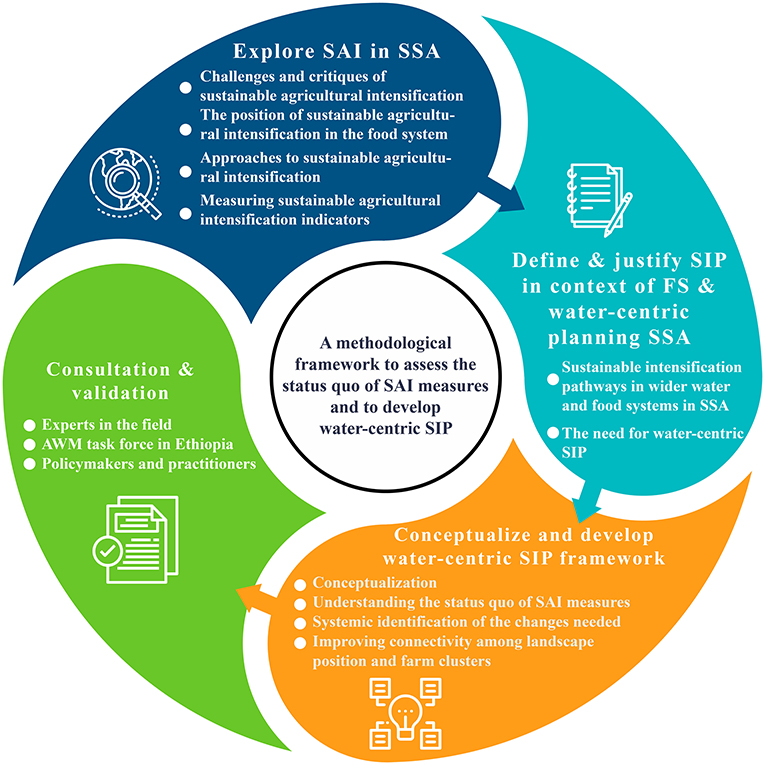
Figure 1. Simplified review framework synthesizing the steps followed and key knowledge ingredients in each step (SAI, sustainable agricultural intensification; SSA, Sub-Saharan Africa; SIP, sustainable intensification pathways).
Sustainable agricultural intensification in a landscape context
Sustainable agricultural intensification has been advocated as a paradigm shift for SSA (Conway et al., 2013). Since many efforts and successful instances of approaches and technologies have focused merely on plot and farm scales (Pretty et al., 2011), there is a paucity of multiple scale planning and interventions which range from plot through farm to landscape. Despite water being advocated as a critical input for SSA agricultural transformation (Conway et al., 2013; Cofie and Amede, 2015), water-centric planning tools and frameworks have been found to be lacking in several different approaches.
Such gaps need to be addressed through a critical understanding of the spectrum of sustainable intensification discourse in the context of SSA and world experiences. Furthermore, sustainable intensification pathways, in the perspective of wider water and food systems as well as in the context of AWM, need greater exposition. The following section of this paper will systematically address these.
Understanding the spectrum of the sustainable agricultural intensification discourse
Challenges and critiques of sustainable agricultural intensification
Sustainable intensification debate has its roots in the Green Revolution discussions. The concept came to be coined in the 1990s in the context of smallholder agriculture in Africa marked by low productivity levels and a degradation of natural resources (Cook et al., 2015; Struik and Kuyper, 2017). In general, sustainable intensification has variously been considered as a goal, a process (Firbank et al., 2018), a trade-off between economic production activity and ecological performance (Gadanakis et al., 2015), or a group of interventions (Godfray and Garnett, 2014).
In practical terms, however, SAI primarily means the delivery of more products per unit of resource while preventing the dwindling of natural resources and ecosystem services now and in the future (Conway et al., 2013; Garnett et al., 2013). Realizing these goals and reducing agriculture's environmental footprint necessitates precision farming as well as the presence of necessary support systems—policies, infrastructure, markets (for inputs and produce) (Mutambara et al., 2016), research and development, and ways to manage trade-offs (Struik and Kuyper, 2017).
SAI approaches are scale-dependent (Struik and Kuyper, 2017)—agronomy and input efficiency practices, for instance, are limited to the field and farm level in SSA (Pretty et al., 2011; Conway et al., 2013) while natural resources management, such as water, is typically manifested at a watershed, landscape, or regional level (Haileslassie et al., 2016; Weltin et al., 2018). Considering the same, a key aspect is planning and practicing SAI across scales in the SSA context. Some SAI approaches draw on the value-add generated through the pro-active embeddedness of the farm into the larger regional/landscape context, i.e., through coordinated actions, cooperation in supply chains, resource allocation or knowledge exchange. These indicate that observing SAI at multiple scales in SSA allows the integration of indicators that can be captured at different levels and in conducting a comprehensive assessment of the effectiveness of SAI approaches as its implementation evolves. In this respect, the usage of a hydrological boundary—hydrological response unit (HRU) as a basis to delineate the landscape boundary—could be a means to match the relevant landscape and water resources approaches and to enable AWM centered SAI assessment and SIP development across scales. Furthermore, the same is applicable to a river basin scale where several landscapes can be identified.
SAI practices in a landscape follow the shift in functional or structural components of landscape or both, with different degrees of a feedback loop between the two (Haileslassie et al., 2016, 2020b). Practices focusing on functional changes include precision farming, e.g., fertilizer micro-dosing in many SSA countries (Pretty et al., 2011), or crop-livestock integration (Haileslassie et al., 2007) as well as targeted decisions on the land use allocation basis the site characteristics and functions. Since water is an enabler and provides the interface of these functional and structural components, therefore, SAI practices and SIP development without adequate attention to water are prone to fail—production of enough quantities of food, feeding and meeting the nutritional needs of a young (60%) and growing SSA population and minimizing the negative impacts of food systems on the environment may all be compromised (Cofie and Amede, 2015). This is also critical in the light of pernicious climate change impacts (Mutambara et al., 2016; AU, 2020).
Allowing agricultural production to be increased without exceeding the earth's local and regional carrying capacity is one of the greatest challenges for SAI (Garnett et al., 2013; Firbank et al., 2018). In addition, another complication is the lack of consensus on the meaning and wider uptake of SAI (Pywell et al., 2015; Musumba et al., 2017; Xie et al., 2019). This is coupled with insufficient attention to the drivers influencing water use in agricultural systems and practices in the landscape along the food chain (including producers, manufacturers, distributors and consumers) (Godfray and Garnett, 2014; Hallstrom et al., 2015; Macfadyen et al., 2015). Trade-offs must be addressed when implementing different interventions, as the process of intensification does not improve the efficiency of all inputs at a time or aligns different actors in input, such as water use (Struik and Kuyper, 2017).
There are various criticisms (Cook et al., 2015) relating to SAI and its water-centered approach: (a) the current interpretation of SAI focuses mainly on production (supply); (b) more emphasis should be provided to resource scarcity such as water (Mutambara et al., 2016); (c) SAI is not viewed as a subsystem of a larger landscape and food system; (d) sustainability is defined too narrowly, with vital social and economic elements neglected, such as, livelihoods, equity, social justice, and economic viability; and (e) there is a focus on corporate-dominated food systems rather than transformation to a system delivering food and nutrition security for all. Notable, while these gaps are global in nature, they do fairly reflect the situation in SSA (Pretty et al., 2011; Conway et al., 2013).
The focus on production, with the consequent emphasis on “high-yielding varieties” has faced robust reproval; such approaches are more “productive” only in response to certain inputs such as fertilizers and water. The measurement of output, especially in rural societies, must consider more than just the marketable elements of crops. This critique also recognizes that the agricultural landscape in SSA is a multi-functional, multi-output system which produces not merely commodities (food, feed, fiber, agrofuels, medicinal products, and ornamentals), but also non-commodity outputs such as environmental services, landscape amenities and cultural heritage (Smith et al., 2017; Mekuria et al., 2021). The output of ecosystem services is analogous to multiple water values (AU, 2020) and entails the need for water-centric planning (Haileslassie et al., 2020a).
In addressing global food security, an excessive focus on yields (and supply) is restrictive since it fails to impart significance to equally important issues of access to food and governance of FS, including demand-side management which is critical in SSA. While Fischer and Edmeades (2010) emphasize the need to look at intensification within the context of the larger FS, it is practiced in SSA in a fragmented manner.
The position of sustainable agricultural intensification in the food system
Figure 2 illustrates the placement of SAI within a sustainable FS. SAI must be considered within a wider sustainable food system, as the need for food security is one of the major drivers of sustainable intensification. Furthermore, addressing multiple issues such as food waste, population growth and natural resources conservation (e.g., water) is critical (Fischer and Edmeades, 2010). Increasingly severe negative impacts on the FS are stemming from climate change, such as the hydrological regime being impacted (Mutambara et al., 2016). Interestingly, food systems are also culpable in this cycle, through direct and indirect emissions (Steiner et al., 2020). Thus, the SAI concept needs to be situated within a larger food system perspective to achieve the goal of increased production in tandem with environmental protection, in addition to SAI contributing to climate change adaptation and mitigation of these impacts. Some intensification interventions—those requiring a change in the structure or function of the landscape components in SSA (Haileslassie et al., 2020b)—are strongly linked to the water system and implicitly need practical interventions (e.g., improved governance of land and water) linked to the food system (Figure 2). Following a summary of SAI from the perspectives of its definition, practices, principles, and its characteristics (Xie et al., 2019), reduction of food waste is suggested as an integral part of a sustainable food system (Figure 2).
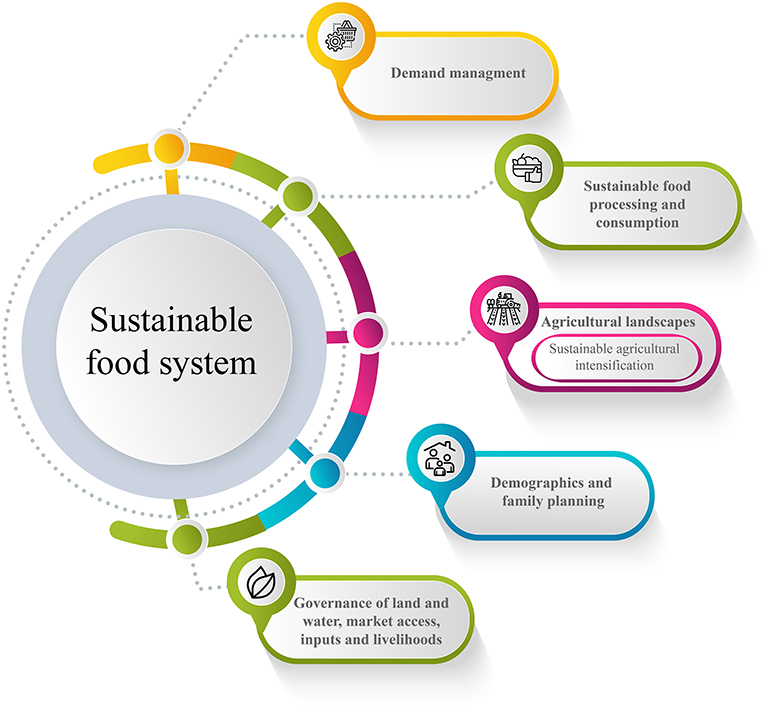
Figure 2. Sustainable agricultural intensification within a sustainable food system in an African or a low-income country (adapted from Fischer and Edmeades, 2010).
Furthermore, while practicing intensification, food security and resource conservation is not guaranteed by ameliorated food production through SAI alone—it is vital to encompass multiple complex factors that determine accessibility to food at different scales. These include purchasing power, socio-political context, and access to distribution channels (MacDonald et al., 2016), all significant in the SSA context. Soil and water conservation in rainfed agriculture illustrates the same, since it does not guarantee sustainable intensification in a watershed as part of the conserved additional water can be used downstream by other farms in producing more food per unit land or for the industrial purpose to sustain food processing. The necessity of enhancing rainfed irrigation continuum in context of the FS is thus made visible.
In general, the SAI activities involving crop selection and livestock management for beef/dairy, as revealed by MacDonald et al. (2016), may require a change in consumption behavior. For example, at a global scale, shifting diets away from animal protein in favor of local crop-derived proteins has the potential to reduce irrigation water consumption by as much as 14%; substantially curtailing supply chain food losses and waste could reduce it by 12%. Diets, waste management, and governance are therefore critical determinants of the degree of crop production increase needed to ensure global food security (MacDonald et al., 2016). In addition, creating a set of robust criteria for deploying SAI in individual SSA countries through multi-stakeholder dialogues is crucial (Mutambara et al., 2016). Such dialogues must embrace representatives of food insecure/vulnerable groups and build upon local knowledge and priorities, and these criteria should provide at least equal weighting to sustainability issues and intensification. Also included should be the need for sustainable intensification to push beyond production and tackle key challenges in processing, consumption and waste, access and entitlements and markets, etc., as illustrated in Figure 2 (Mutambara et al., 2016). Furthermore, the SAI framework—focusing predominantly on the production side—must transcend agricultural production to encompass indicators and principles which support monitoring of natural resource allocation and equitable and efficient use, such as of water, within transforming landscapes (Pereira et al., 2012). This is also vital in capturing competing water demands between agriculture and other sectors and influencing the feasibility of sustainable agricultural intensification in a given SSA landscape.
Approaches to sustainable agricultural intensification
Within sustainable intensification, there are broadly considered to be three pillars (Godfray and Garnett, 2014)—ecological, genetic, and socio-economic intensification—not necessarily mutually exclusive, with diverse practices in each; this has been similarly emphasized within the SSA context (Conway et al., 2013). Some work suggests that there is no single generalizable model of ecological intensification practices, which are rather site-specific (Kremen et al., 2012; Lemaire et al., 2014). Such practices include the implementation of measures related to agroecology, organic agriculture, diversified farming systems, and some forms of conservation agriculture, agroforestry, and integration of crops with livestock systems (Haileslassie et al., 2007; Conway et al., 2013). Ecological intensification underscores the understanding and intensification of biological and ecological processes and functions in agro-ecosystems and extends its scope to landscape use and ecosystem service (Xie et al., 2019). Inclusion of landscape within ecological intensification corroborates the consideration of multiple and interactive scales to capture resources (such as water) to be a key input and intensification enabler. Such a multiple-scale approach can capture the sources and sinks of water and thus aid in practicing reasonable water allocation and judicious use. Water is a fundamental driver of ecosystem services. While the characteristics of ecological intensification—e.g., ecological process and ecosystem services (Xie et al., 2019)—reveal water-centered SAI to be important, its practical application could pose a daunting challenge.
Genetic intensification in SSA can range from “conventional plant breeding,” “biotechnology,” and “livestock breeding” which incorporates elements of plant and animal breeding technologies. An important goal for genetic improvement of crops is to adapt existing food crops to be resilient to increasing temperatures, decreased water availability in some places and waterlogging in others, rising salinity, and changing pathogen and insect threats as a consequence of climate change (Conway et al., 2013; Abberton et al., 2016). Genetic improvement through livestock breeding attempts to sustainably increase the productivity of livestock with little to no increase in the amount of land devoted to livestock fodder production and grazing (Steiner et al., 2020). Water saving may be undertaken in delivering livestock products and services, though the practice may vary. To enable improved water saving in SAI in SSA (e.g., for the livestock sector), a model that synchronizes livestock breeding, feed sourcing, and feeding is vital, given the high volume of freshwater input required to produce a unit of dairy and beef products (Haileslassie et al., 2011). Transforming the agricultural paradigm away from high inputs toward stability and environmental resilience of yields, alongside decreasing inputs, is a daunting challenge (Conway et al., 2013). However, it may be met with the accelerated application of genetic intensification tools coupled with judicious consideration of associated input packages such as water productive feed sourcing and feeding in SSA (Haileslassie et al., 2011; Abberton et al., 2016); in this context, water-centered planning can be critical.
Socio-economic intensification incorporates supportive enabling environments and building the social and human capital of smallholder farmers in SSA (Conway et al., 2013). The former combines macro-economic policies that favor markets and trade, the provision of inputs and physical and social infrastructure as well as institutions (Conway et al., 2013; Yami and van Asten, 2017). Social capital is the value generated through social networking, trust, and cooperation within and between people and organizations, as well as through culture and tradition. It is an important element for creating sustainable livelihoods and economic development in SSA (Conway et al., 2013; Rivera et al., 2019). Human capital or capacities, such as education, skills, and health, can foster productivity and improve livelihoods and food security, and play a critical role in economic growth and development since human actors are at the center of production, processing, distribution and consumption (Davis et al., 2007). Since agricultural education and training, extension and research are critical aspects of the capacity building of farmers, more investments are needed in education to improve the human capital in SSA (Conway et al., 2013).
Lessons learned from the Green Revolution have highlighted the higher yield and better food security garnered through an emphasis on a few intensification pillars such as high-yielding varieties, irrigation, mineral fertilizers, and agrochemicals (Conway et al., 2013; Struik and Kuyper, 2017). However, notable negative consequences include the depletion of water resources and the salinization of agricultural land in many parts of the world like Gujarat (Mutambara et al., 2016; Steiner et al., 2020) as well as SSA. To evade the same, SIP development and SAI implementation must account for AWM. Sustainable agricultural intensification efforts without practical integration of the three sustainability pillars and centering of critical resources, such as water, can deplete the resources leading to the collapse of the food system.
Measuring indicators of sustainable agricultural intensification
Sustainable agricultural intensification requires indicators and associated metrics for tracking progress, assessing the trade-offs, and identifying synergies (Pereira et al., 2012; Haileslassie et al., 2016; Smith et al., 2017; Xie et al., 2019). Furthermore, measuring and monitoring sustainability also provides an opportunity to exercise adaptive management (Haileslassie et al., 2020b), while the indicators used can assist in understanding the status of the SAI gaps elaborated earlier. A review undertaken of 60 publications (Smith et al., 2017), has organized the SAI indicators into five dimensions: productivity, economic sustainability, environmental sustainability, social sustainability, and human wellbeing.
Productivity has been articulated in a variety of indicators and metrics (Smith et al., 2017) including yield, efficient use of inputs including water (Pereira et al., 2012) and animal health. Commonly cited indicators of economic sustainability include agricultural income and crop value. Metrics of agricultural income at the field level include net income from agriculture (Castellini et al., 2012), disposable income (Meul et al., 2008), losses of agricultural income due to natural disasters, or changes in total agricultural income (Zhen et al., 2005). The profitability of a crop and the estimated labor required to grow a crop comprise the crop value metrics (Gafsi et al., 2006). One of the indicators included in the human wellbeing dimension is food and nutrition security (Kamanga et al., 2010). Biodiversity conservation, carbon sequestration, soil erosion, nutrient dynamics, soil biological activity, soil quality, and in many cases the productive uses of water are indicators of environmental sustainability (Thrupp, 2000; Phalan et al., 2011; Pereira et al., 2012; Conway et al., 2013).
Social sustainability is measured by indicators such as information access and gender equity (Phalan et al., 2011). Farmers' access to information can be gauged by their level of connectivity within the agricultural knowledge network, consisting of farmers and local experts (Hoang et al., 2006). It can also be measured quantitatively by administering a test to farmers regarding a set of agricultural practices. Gender equity in each SAI effort can be evaluated by the percentage of farmers participating in the project or adopting an SAI technology suitable for women. Equal access to resources for male and female farmers could also be an indicator of gender equity in SAI (Degrande et al., 2013). Furthermore, equity in the impacts of intensification efforts can be reflected in the distribution of labor, or the proportion of SAI-related work performed by male relative compared to that performed by women, along with recognition of other attributes of inclusion and gender. This can be applied, for example, to some aspects of common property resources management, demand-side gaps analysis and livelihood options (Fischer and Edmeades, 2010).
Despite the attempt to measure water resources sustainability (at farm and plot level and more so for production-oriented SAI), water in the perspective of ecological sustainability and across multiple scales and multiple uses is in fact, a far more critical indicator than what is suggested (Pereira et al., 2012; Conway et al., 2013; Haileslassie et al., 2016; Smith et al., 2017; Xie et al., 2019). Water is a fundamental driver of ecosystem services and as discussed earlier, indicators on water allocation across multiple uses—ecological, social, cultural, and economic (Haileslassie et al., 2020a)—are missing for the SSA region. In general, water is an intensification enabler and an interface of the functional and structural components of an ecosystem. Limiting its indicators to a single dimension (economic or ecological or social) will deter a true picture of sustainability from emerging, distorting the SIP.
Sustainable intensification pathways in perspective of wider water and food systems
SIPs are a means toward SAI in achieving food security, social inclusion and environmental sustainability goals. Sustainable intensification pathways can largely be considered as balancing trade-offs between intensification and sustainability across its key dimensions (social, economic, and environmental) and diverse scales (Haileslassie et al., 2016; Kumar et al., 2019). Such an approach can help minimize the probability of hitting a non-beneficial tipping point (Xie et al., 2019) which is a critical point in a situation, process, or system beyond which a significant and often unstoppable effect or change takes place. There is a need to focus on the socio-economic, ecological and genetic intensification in conceptualizing practical approaches to SAI for SSA (Conway et al., 2013). Knowledge on integrating these approaches into the landscape scale and water-centered planning is complex and often deficient.
Different farm types can coexist in a landscape in SSA countries such as Ethiopia, with numerous heterogeneities in terms of the level of SAI efficacy and its pathways within a landscape (Mutyasira et al., 2018), with several farm types in a landscape involving farms with high intensification-low sustainability, low intensification-high sustainability, low intensification-low sustainability and high intensification-high sustainability. While SIPs attempt to bring many farms to an intensive-sustainable status, identification of common pathways to this destination in the realm of such diversity is a significant challenge.
This complexity partly stems from the specific location of the farms in a landscape that influences the biophysical and climatic conditions and their access to water resources for irrigation. Farms situated in the upper landscape may need to focus on soil and water conservation-based intensification to reduce on-site erosion induced land degradation. This leads to a longer growing period (onsite), higher yields, and flow of water (surface and groundwater) to lower landscape positions. The process supports the idea of operationalizing rain fed-irrigation continuum across a scale, illustrating water to not only be a point of convergence of SAI measures but also a chief factor in linking lives and livelihoods across landscape positions (Kumar et al., 2019). The challenge arises in supporting this connectivity.
A smaller sized SAI plan may have linkages to a more homogeneous group of farms, reducing the issues of aggregation in developing the SIP (Mutyasira et al., 2018; Kumar et al., 2019). This means a multi-scale approach where farms are nested in farm typologies and farm typologies are nested in the landscape can help identify effective SIPs at a landscape scale for SSA. Such a nested approach requires both landscape-level and farm-level indicators that complement each other.
Much like its spatial scale, SIP is also time-bound. Change in external drivers, actors' behavior, or emerging issues such as climate change can shift the threshold points of sustainability. Thus, a systematic decision and continuous co-learning through an adaptive management approach to achieve sustainable intensification in SSA becomes necessary (Birge et al., 2016; Haileslassie et al., 2020b).
Designing sustainable intensification pathways
The principles of SAI are applicable to any production system and agricultural landscape and its associated value chains, whether it is conventional, organic, or some other system of agriculture. SAI can be implemented in farm enterprises of different sizes and degrees of market integration. It should be noted, however, that simplistic, universal prescriptions or recommendations cannot lead to successful SIPs (Lobell et al., 2009). Designing the right SIPs requires an understanding of the status of SAI in terms of the condition and trajectory of a system and the reason behind certain trends occurring in specific contexts, as illustrated by the priority indicators set by actors reflecting their challenges, opportunities, and ambition at different scales (i.e., landscape, community/local, and farm). A knowledge of the practice and status quo of sustainability indicators identified through stakeholder engagement is indispensable. A combination of qualitative and quantitative methods which explore the level of resources endowment and utilization, community livelihood and future expectations and political ambitions is essential (van Ittersum et al., 2013).
SIPs should not aim to imitate the natural ecosystems that have not been optimized for food, feed, fiber, or bioenergy production (Denison et al., 2003). Instead options should be derived from the sustainable use of natural systems, traditional systems, and “alternative” systems, from experimentation and traditional knowledge as well as scientific theory and empirical observation. These options need to be tailored to local conditions by the use of well-integrated research and development systems subsequent to a participatory approach. Although many principles for systematic SIPs development are generic, the scope for increasing eco-efficiency in agriculture varies widely.
Research on SAI has illustrated the pathways which combine the maintenance or increase of agricultural production on the same area of land and the contribution to sustainable development in a balanced way (Godfray et al., 2010; Garnett et al., 2013; Gadanakis et al., 2015). Four fields of action based on the spatial scale have been identified (Weltin et al., 2018), including agronomic development, resource use efficiency, land use allocation, and regional integration, whether these are SIPs or just fields focusing on productivity improvement. The first, agronomic development, ranges from adapted cropping such as crop rotations and intercropping to available land and water resources as well as climate patterns. Within a specific crop management system, practices can contain the choice of variety and crop management including techniques from tillage to soil conservation (Townsend et al., 2016). This field of action also looks at the use of novel technical solutions, new digital technology applications, the use of site-specific information and system data, and precision farming.
Sustainable intensification practices grouped under resource use efficiency center around the SIPs aiming to increase agricultural productivity either through the use of fewer inputs or with the higher production at a given input level (Weltin et al., 2018). Resources could be natural (green, blue and greywater, manure, residues, and animal feed), chemical (fertilizers and pesticides), and human (labor, knowledge and managerial abilities). The majority of approaches related to resource use efficiency (Weltin et al., 2018) pertain to fertilizers (fertilizer use efficiency and measurements of soil nutrient balances and losses), residues and water (marginal water use, integrated crop water management or rainwater harvesting). However, sustainable resource use on-farm may not generate sustainable intensification at a basin level. The approaches associated with human resources, such as knowledge management and labor productivity, are less frequently covered by the current SAI (Weltin et al., 2018).
The field of action of land-use allocation comprises of approaches that aim at improving the joint provision of various environmental services in the same landscape and/or to produce the same amount of food and biomass on less land or in a different organization of land (Weltin et al., 2018). Most of the literature surrounding the same concerns landscape design and its decline in the land sharing and land sparing SAI approaches (Shackelford et al., 2015). Both the dwindling land and water resources in SSA (Mutambara et al., 2016) available for increasing agricultural productivity through extensive agriculture, along with the need to conserve biodiversity and key ecosystem functions call for the holistic integration of productive and natural spaces at the landscape level and the Identification of innovative land-use practices.
The land sharing and land sparing intensification approaches like the integration of livestock and pasture, coffee plantations and native vegetation and birds are linked to coexistence on a specific landscape of agricultural production (Mastrangelo and Gavin, 2012). Such approaches also work with mixed crop-livestock systems on the landscape scale. Sustainable agricultural intensification practices grouped in this category generally increase the diversity within the agricultural systems, allowing for the improved regulation and maintenance of key environmental services such as water through a diversified landscape mosaic (Lemaire et al., 2014). This implies that certain land uses have a lower application of water and can enhance “natural storage” in the landscape. Hence the diversification at the landscape level can only benefit ecosystem services if suitably undertaken, for instance through water-centric planning and judicious implementation.
Regional integration covers elements such as knowledge exchange and innovation diffusion, the functioning of institutions, governance mechanisms and local networks (Mutambara et al., 2016; Weltin et al., 2018). Institutional changes such as taxation, land tenure policies, or access to credits, improved forms of leadership and governance are considered to be triggers for SAI practices (Southern et al., 2011; Bird, 2014). Such intensification practices play a significant role in cooperation and experience exchange between different actors including policy and decision-makers at the regional level for different purposes, such as common resource use, value chains and marketing strategies (Mutambara et al., 2016). Studies indicate that multi-level and multi-stakeholder networks in SSA can enable common resource use, redistribute inputs and close nutrient loops (Pretty et al., 2011), and emphasize not only the role of extension services (Pretty et al., 2011), but also the effectiveness of farmer-to-farmer learning (Baulcombe et al., 2009).
Numerous studies have looked at SAI and generic SIPs by considering emerging issues such as environmental externalities and climate change (Steiner et al., 2020). The focus is largely upon understanding the yield-limiting (soil, water and plant varieties), yield-reducing (pests), enabling environments (policies and institutions) and the complementarities and minimized trade-offs (among the package-ingredients and external factors) in proposing the pathways (Lobell et al., 2009; Bindraban and Rabbinge, 2012). According to projections, climate change is predicted to hit, in particular, water resources even harder and impact agricultural production and ecosystem services (Haileslassie et al., 2016; Mutambara et al., 2016). Keeping the same in mind, the new model for designing effective SIPs must consider water resources (Cofie and Amede, 2015); the following section elaborates on the same.
SIPs in the context of agricultural water management
Current predictions indicate declining water availability and the occurrence of more frequent and severe droughts and floods in the coming decades in many agricultural regions worldwide (Rosegrant et al., 2009). Agriculture is responsible for about 70% of the global freshwater withdrawals; notably, this figure can reach to more than 90% in some agricultural economies (such as SSA). Of the total world food production, 40% is derived from irrigated systems on only about 20% of the arable land area. More investments in improving water productivity in existing schemes and safely expanding irrigated agriculture are thus vital for sustainable FS, with a strong emphasis on policies and new technologies that ensure maximum efficiency and productivity while protecting critical freshwater resources and the environment (Rosegrant et al., 2009; MacDonald et al., 2016; Mutambara et al., 2016; Pretty et al., 2018; AU, 2020). Integrated solutions must balance the use of surface water and groundwater resources by different sectors (Gleick, 2003) while optimizing water productivity in the whole FS. Consuming less water for instance can also be achieved through wasting less food, consuming less water-intensive food, and augmenting the water use efficiency in crop-livestock systems; altogether creating practical linkages to a sustainable FS (MacDonald et al., 2016).
Of the total the cropped area, about 80% worldwide and more than 90% in the SSA region depends on rain-fed agriculture. Rain-fed agriculture is vulnerable to increasing climate variability which leads to significant yield loss (Mutambara et al., 2016). With factors such as increasingly variable and uncertain rainfall, long dry seasons, recurrent droughts, and dry spells as well as floods, water is a key constraint for rain-fed agricultural production systems, especially in tropical semiarid and arid regions (Gleick, 2003; AU, 2020). The challenge of 50% more production of food (FAO, 2017) in the rain-fed dominated global agricultural system is significant, with the situation for SSA being potentially dire (AU, 2020).
Sustainable intensification pathways in SSA in the context of AWM and water-centric planning recognize multiple values of water (Haileslassie et al., 2020a), enhancing water availability and expanding irrigation (full and supplementary) by optimizing consumptive and reducing non-productive water losses (Pereira et al., 2012; MacDonald et al., 2016). There is a huge potential for crop water management to sustainably intensify agriculture including multiple production and mitigation of crop failure risks related to dry spells. Along this line, many global policies, including the ones at the regional level in East Africa, concentrate on irrigation development to meet food demands (Nakawuka et al., 2018). Although this approach is important in different contexts, there are recognized limits in the scale of its expansion in many parts of the world. Only a small proportion of cultivable land in Africa (5.5%) is suitable for irrigation (Abrams, 2018) because of limited water availability and soil/topographical characteristics. Contrastingly, Altchenko and Villholth (2015) suggest that the total area of cropland irrigable with renewable groundwater ranges from 44.6 to 105.3 × 106 ha, which corresponds to 20.5–48.6% of the cropland over the continent; there is visibly diverse opportunity for AWM-based SAI in Africa (Nicol et al., 2015). Optimal climates are specific to each cropping system (Li and Troy, 2018), where irrigation provides a benefit; there are also other conditions where irrigation proves to have marginal, if any, benefits. It is also prudent to understand at which point and under which circumstances irrigated agriculture is more beneficial than rain-fed agriculture and the best way for the two systems to complement each other (Vanschoenwinkel and Passel, 2018).
Different farms should consider water management options across a wide spectrum ranging from purely rain-fed farms to purely irrigated ones (Vanschoenwinkel and Passel, 2018). Some farming, for example in East Africa, is noted to be moving toward somewhere in between rain-fed and irrigated agriculture, i.e., practicing supplementary irrigation and water conservation measures (Nicol et al., 2015).
Access to green and blue water for agriculture (Vidal et al., 2009) is not simply addressed by opposing rain-fed and irrigated agriculture—agricultural systems have often not been strictly rain-fed or irrigated. Irrigation farmers typically also use green water and at times rain-fed farmers, if possible, also use blue water, even in the absence of formal irrigation systems (Vidal et al., 2009). Farmers have predominantly always dealt with a green to blue water continuum, struggling to extract the best product value from the same. Moving along the continuum, the impact on hydrological processes intensifies, with varying accessibility for other actors and sectors. Furthermore, the inclusion of greywater has become increasingly important in areas dealing with physical water scarcity in SSA (Vidal et al., 2009; Mutambara et al., 2016); this further complicates the SIP design.
In nurturing the rain-fed and irrigation continuum through principles and practices of SIP planning, the significance of water-centric planning in pursuing SAI in SSA and globally is paramount. This is revealed by the following factors:
i) The agricultural landscape is a system hierarchy stretching across plots, farms, watersheds and basin, operating within the same hydrological system. Water flows create intra- and inter-system linkages; therefore changes in one part of the landscape affect water availability and attendant livelihoods and ecosystem services (provision, regulation, support, and cultural) in other parts. Water-centric SIP design ensures desirable water quality and quantity while maximizing agricultural production in the upstream areas. This leads to mitigation of impacts, and provision of reliable water supply to the downstream areas.
ii) Climate change is projected to reduce renewable surface water and groundwater resources significantly in the driest subtropical regions such as SSA (Mutambara et al., 2016). Competition for water among different uses and users will only be amplified, threatening water, energy, and food security (Boretti and Rosa, 2019). Water-centric SIP design can ensure judicious use and optimum allocation.
iii) Farmers in SSA have not managed well in adapting to increasing water scarcity in the face of climate change, especially on the exploration of ground water (Mutambara et al., 2016). Africa's intensification efforts are in part triggered by Sustainable Development Goals (SDGs) (Pretty et al., 2018), with water at the heart of many of the Goals. It is a key input, identified to be a resource cross-cutting several SDGs and their respective targets—no poverty (SDG1), zero hunger (SDG 2), good health and wellbeing (SDG3), gender equality (SDG 5), climate action (SDG13), life below water (SDG14), and life on land (SDG 15). Achieving the targets under each of these goals in a region as water scarce as SSA without water-centric SIP becomes a major obstacle.
iv) Global water demand will grow significantly over the next two decades in the industry, domestic and agriculture sectors. While industrial and domestic demand will outpace agricultural demand, demand for agriculture will nonetheless remain the largest, increasing by 60% by 2025. These figures could be even higher for SSA given the young population and the high growth rate (Boretti and Rosa, 2019). In total, this entails the need for judicious use of water ensured through water-centric SIP.
v) Water is the major yield-limiting factor globally and in SSA. Meeting a 50% expected increase in food demand will not be possible without a water-centric SIP design. Within SSA, meeting future cereal demand will not be feasible with the existing production area through yield gap closure alone (van Ittersum et al., 2016). A yield gap analysis for 10 countries in SSA revealed that in addition to yield gap closure, other more complex and uncertain components of intensification are also indispensable. These include increasing cropping intensity (the number of crops grown per 12 months on the same field) and sustainable expansion of irrigated production area (van Ittersum et al., 2016). Similarly, Agenda 2063 of Africa Union proposes to increase water productivity from rainfed and irrigated agriculture by 60%, harvest at least 10% of rainwater for productive use and recycle at least 10% of wastewater for agricultural and industrial uses (AU, 2013). Considering the same, AWM-centric SIP can unlock Africa's potential to ensure food security.
The proposed methodological framework for sustainability assessment and development of water-centric sustainable agricultural intensification pathways
Conceptual framework
Sustainability assessment is a complex appraisal system that supports decision making and policy formulation in the broader social and environmental context. It transcends purely scientific evaluation (Sala et al., 2015; Xie et al., 2019)—there have been several attempts, for the last decades, to develop a sustainability assessment framework (Sala et al., 2015; Smith et al., 2017), on top of efforts to categorize sustainability assessment practices. Some look specifically at the tools applied (Gasparatos and Scolobig, 2012), while others focus on the broader process employed and the aims and scope of the assessment (Hugé et al., 2013). There are gaps in terms of the understanding of SAI status quo, designing SIP and employing adaptive management.
AU (2020) initiated a framework for irrigation development and AWM in Africa (IDAWM), which is structured around four strategic AWM areas or agricultural water developmental pathways. It includes AWM in rain-fed farming; farmer-led irrigation; scheme development and modernization; and unconventional water use for irrigation. IDAWM is a high-level direction of development, therefore does not include a detailed methodology, details on implementation and on the ways that different plans can complement each to bring additional benefits.
Critiques highlight the failure of the SAI assessment and SIP development efforts to address: (a) how sustainability concept and assessment methods evolve in time and space with emerging agricultural techniques and a better understanding of global challenges such as climate change and the limitation of freshwater resources (Mutambara et al., 2016); (b) methodological principles and elements to couple agricultural sustainability assessment with SIP development; (c) the means to integrate the different pillars of sustainable agricultural intensification; (d) how the best indicators of sustainable agricultural intensification are anchored in the overall FS, and (e) how to operationalize the multiple scales approach of agricultural sustainability assessment and SIP design to capture the performances of different attributes, which is unlikely at a single scale.
We argue that a framework that outlines how to move from agricultural sustainability assessment per se to the development of SIPs is crucial to enable complementarities and synergies of interventions and address existing and emerging challenges.
The water-centric framework proposed here (Figures 3, 4) aims to ensure comprehensiveness, flexibility, and robustness in the evaluation and planning of SAI. The framework is constructed from four building blocks: (a) targeting sustainable agricultural intensification dimensions (Figure 4A); (b) understanding the status quo of SAI measures (Figure 4B); (c) defining SIPs to bring desired change and manage the trade-offs (Figure 4C), and (d) principles and approaches of SIP design which enable synergies and complementarities of interventions (Figure 4D).
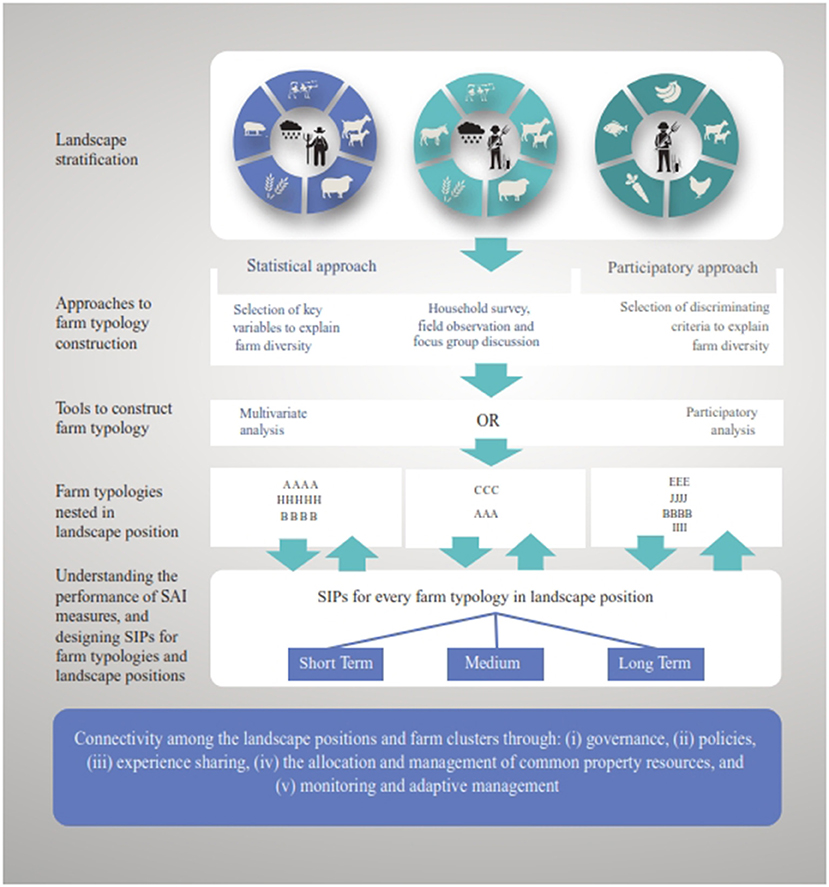
Figure 3. Schematic diagram illustrating how to operationalize the multiple scales approach in sustainability assessment and SIP planning.
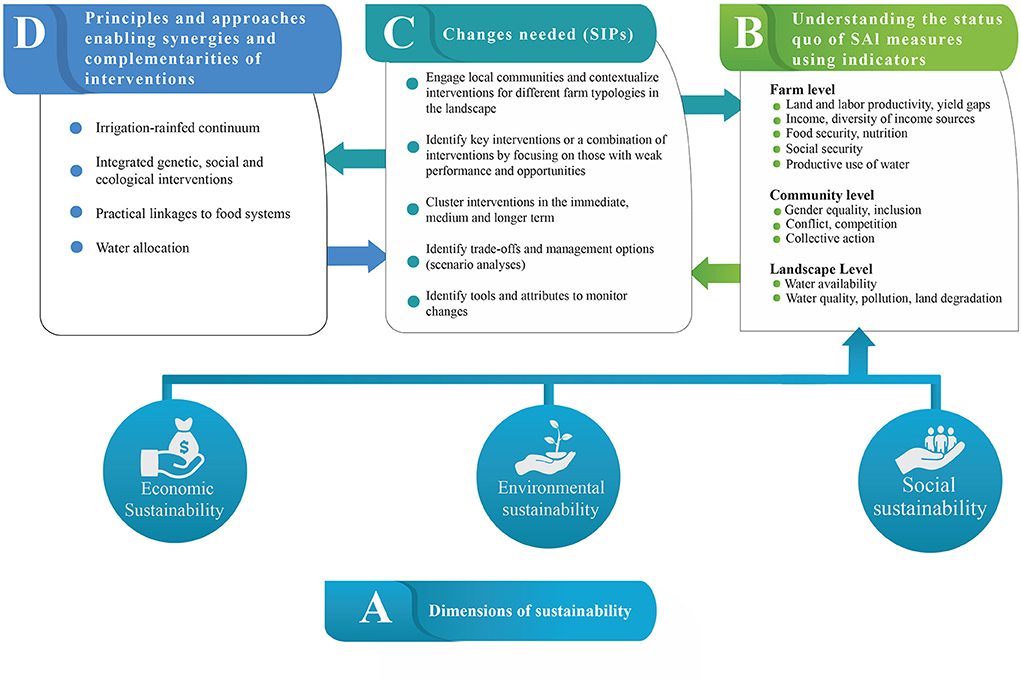
Figure 4. A methodological framework to assess the status quo of SAI measures and to develop sustainable agricultural intensification pathways (SAI: Sustainable agricultural intensification; SIPs: Sustainable intensification pathways. Each alphabet (A–D) represents sequential steps and explained by texts next to it)
Figure 4 is an integral part of Figure 3 and it illustrates: (a) the approaches to address landscape and farm level heterogeneity; (b) the approaches operationalizing the sustainability assessment and the development of SIPs at multiple scales; (c) links with weak performing indicators with changes needed (SIPs) in time (short, medium and long term), space (landscape positions) and social context (farm type/income level/tenure); (d) combination of sustainability assessment, SIP development, and adaptive management. The following sections discusses each of these components.
Defining sustainability dimensions to understand the performance of sustainability indicators and the changes needed
In SAI assessment, the starting point is deciding on sustainability dimensions which form the basis for indicators co-identification. The sustainability dimensions (Figure 4A) in the water-centric framework build upon earlier work from Haileslassie et al. (2016). The relative importance of sustainability dimensions is pliable and should be fixed in discussion with actors such as local decision-makers and the community. Particular attention can be given to emerging threats (e.g., climate change or a global pandemic like COVID-19), opportunities and political ambitions. The sustainability dimension must place at the forefront the concerns which need special attention in the perspective of local communities—the first entry point to design the SIP. For this study, a water-centric approach was deployed since water management is a challenge in most cases in many SSA countries.
Understanding the status quo of SAI measures: A critical step following the sustainability dimensions is to understand the status quo of the SAI measures (Figure 4B). This involves the identification of an inclusive matrix and indicators in a participatory manner. SAI indicators are an important tool to inform the design of SIPs by offering a basis. The same enables a better understanding of current conditions, goals, identifying trends and targeting changes needed in SAI practices; furthermore, it aids in comprehending and monitoring the progress made in both relative and absolute terms. Indicators can also help in evaluating and selecting alternatives, given that they are concise and easily interpreted—thus, they too can offer an entry point to enable water-centric SIP planning.
It is important to distinguish between indicators as landscape units, structures, functions or processes and as measures, i.e., properties of a phenomenon, body, or substance to which a magnitude can be assigned, and between descriptive and normative indicators. Generic indicators and matrices contributing to each of the target dimensions can be identified using previous studies (Pereira et al., 2012; Haileslassie et al., 2016; Smith et al., 2017). Since every potentially available indicator cannot be utilized, so an element of simplification while maximizing unique and relevant information, is essential. Balancing of indicators for sustainable intensification of crop production at the field and river basin levels is also recommended (Chukalla et al., 2020), confirming the significance of multiple scales and water-centric SAI.
Subsequently, the above must be contextualized basis the desk work, key stakeholder consultations, and expert knowledge of the site (Figure 4B). Table 1 describes an example of generic indicators proposed to understand the status quo of a water-centric SAI assessment. During the first visit, farmers will be asked to co-develop additional indicators. Participatory pairwise ranking approach can be used to prioritize indicators. Indicators that do not reflect end-users' interest and ambition, as well as the SIPs based on the same would not be adopted much.
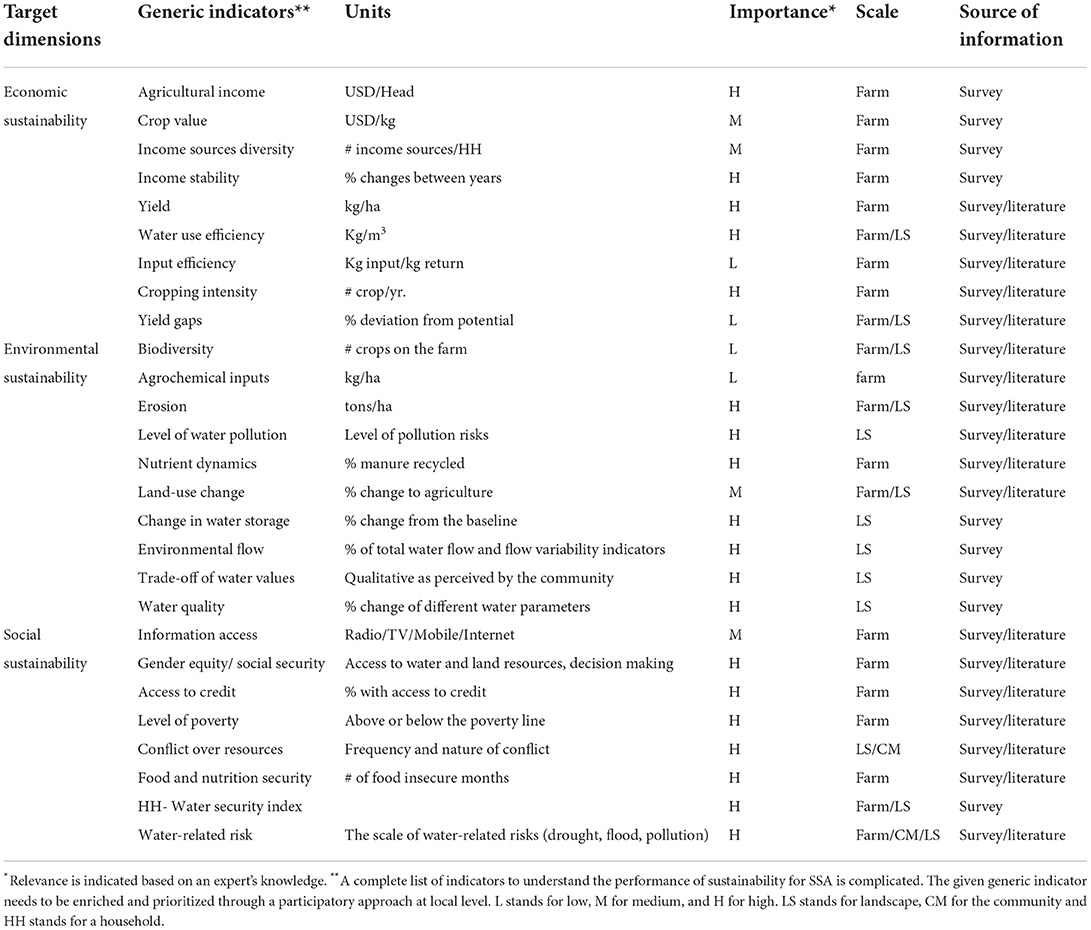
Table 1. Generic indicators and indexes proposed for understanding the performance of sustainability.
Much like to the sustainability dimension, the indicators can be used as an entry point to address the erstwhile gaps in the SAI practices (Heink and Kowarik, 2010). For instance, the relevance and level of integration of environmental, genetic, and institutional intensification and elements of the sustainable food system such as governance, NRM and demand management could be explored. While water use efficiency and access to water may be essential indicators (Pereira et al., 2012) at the farm and landscape level, practices reinforcing rain-fed irrigation continuum (e.g., off- and in-situ water harvesting) and bringing the ecological intensification into the forefront could provide the principle and strategic direction where the water-centric SIPs could be rooted. Investigating the relevant indicator at this stage is beneficial in realizing water-centric SIP.
Time, space and a social dimension are critical in the identification of indicators. Common landscape-level indicators may not be relevant for farms, and vice versa, although some may be cross-cutting. Furthermore, today's indicators for food security might be less relevant in the long run. This implies that the indicators could be scale specific but also interactive across scales (Figure 4B). Farms and landscapes are heterogenous, hence reaching mutual consent on the choice of representative indicators is a challenge, and the question is how to address these system hierarchy and scale dimensions at a time.
Different techniques are available to deal with heterogeneity, of which the common practice is to cluster farms and landscapes into a homogeneous group (Haileslassie et al., 2016) (Figure 4). Landscape typology can be constructed using traditional altitude belts or the farming systems (Figure 4) in the landscape under consideration. A quantitative statistical tool such as multivariate analysis, based on a survey dataset, can be applied to construct farm typology (Haileslassie et al., 2016; Mutyasira et al., 2018). Alternatively, participatory learning and action may be undertaken, employ informal group discussion and key informant interviews to create farm typology (Figure 4). This method is advantageous due to the inclusion of additional groups of females and “landless” farmer—these groups are significant in the communities but were omitted in the survey for the statistical typology. Such an approach would help in managing system hierarchy, by nesting the individual farms into farm clusters and subsequently the farm clusters into landscape position (Figure 4).
To understand the changes needed, the next step involves the status of sustainability indicators by comprehensively exploring the performances of each of the selected indicators (Figure 4B). Depending on the number of indicators identified, sustainability assessment could be data-intensive and therefore expensive. In keeping the exercise cost-effective, relevant and locally available datasets addressing scales of interest should be located.
Data sourcing mechanisms ought to consider multiple scales and methods. While Household Survey (HHS) is a useful tool to capture farm-level data for the target indicators, Focus Group Discussions (FGDs) and Key Informant Interviews (KIIs) can help collect qualitative data at community/farm typology and landscape levels. Geospatial datasets can be used to triangulate the HHS datasets and capture bio-physical data at a landscape scale (e.g., weather attributes, groundwater distribution). Furthermore, due to the ongoing advances in high-resolution satellite imagery and computing engines like the Google Earth Engine, the use of products derived from Sentinel 2 or Landsat, such as FAO Water Productivity Open-access Portal (WaPOR), can complement the household surveys and assess key changes in land and water resources across watersheds in a landscape.
Analysis of the relevant datasets could involve, for example, simple descriptive statistics such as coefficient of variation (CVs) or the development of indexes such as food security, water productivity, biodiversity and poverty (Table 1). Depending on the target indicators, point and spatial modeling exercises can be considered to quantify risks (climate change-related, erosion), opportunities (availability of groundwater, achievable yield), and trends. These can assist in the evaluation of the farmers' vision of short-, medium- and long-term livelihood ambition.
The performance of sustainability indicators can be evaluated for each sustainability dimension and at the individual indicator level. Individual indicator level evaluation is important in essential in determining where future intervention (SIPs) is needed, with comparisons that can be made among the values of indicators in relative or in absolute terms. The absolute and relative values of the performance of the indicators reveal what is achievable under the current level of knowledge and practices, can track the progresses made over time. Tools such as radar charts can be engaged to visualize and compare the performance of indicators and identify the specific benefits and trade-offs.
Systematic identification of changes needed (SIPs): One of the bases for SIPs design is the status quo of SAI measures. In addition to the poor performing indicators, SIPs design is determined by farmers' livelihood expectations, government policy goals, emerging risk, and available options. Mixed methods involving science backing and community engagement help reveal the causes behind weak performing indicators and in prioritizing the interventions and clusters emanating from the SIPs design determinants. SIP is about co-designing context- specific interventions through an iterative participatory process (Kumar et al., 2019). The prioritization of the different interventions could follow simple tools such as pair-wise ranking. This type of discussion can be organized along with status quo feedback and SIP-co-design sessions at the farm typology and landscape levels.
In identifying the intervention or cluster of interventions, for farm typologies and landscape for a specific time scale (Figure 4C), critical thinking is vital, and must include: (a) the type of innovations required to address priority constraints concerning results of the SAI assessment; (b) potential risks such as climate change (Mutambara et al., 2016) and opportunities like the level of water resources endowment; (c) scenarios to manage trade-offs and principles to integrate innovation and harness their complementarities—irrigation rain-fed continuum, integrating genetic, social and ecological interventions; practical linkage of SAI to FS, equity, water allocation etc. (Figure 4D), and (d) a model to maintain and improve connectivity among farm clusters and landscape positions through governance (policies, institutions) to understand resources allocation, common property resources management and experience sharing (innovation platform). In SIP design and its implementation, the application of adaptive management can help navigate the critical thinking and post-implementation monitoring and learning.
Improving connectivity among landscape positions and farm clusters: The proposed framework assumes landscapes bounded by large-size watersheds and livelihood activities and natural processes connected through the hydrological process. This entails the presence of improved governance mechanisms which can coordinate among upstream-downstream and incentivize or disincentivize SAI measures (Clement et al., 2011) (Figure 4). From the perspective of SAI, governance refers to the norms, institutions and processes that determine how power and responsibilities over natural resources are exercised. It comprises how men, women, indigenous people, local communities and marginalized members of the society (minorities) participate in the decision-making process and benefit from natural resources management (NRM). Rules and norms for the same can be both formal and informal. A wide range of social movements, networks and federations have emerged to support the transitions toward sustainability and equity in SSA (Pretty et al., 2020). Social capital has been established in SSA, with the presence of intentionally formed collaborative groups within specific geographic territories—water user association, innovation platform and watershed management group. Local governance can facilitate experience sharing with these collaborative groups, like local institutions (Mutambara et al., 2016), in turn enhancing the spill-over effects of the SAI measures.
SAI practices may veer in unpredictable trajectories, e.g., environmental pollution, land use conflict, as experienced in many instances in the SSA region (Assegide et al., 2022; Teklu et al., 2022). SIPs design cannot be a one-time undertaking; it must evolve to address emerging changes and capitalize on new opportunities (Kumar et al., 2019). Thus, mechanisms to operationalize adaptive management approaches may prove useful (Haileslassie et al., 2020b). Adaptive management is an approach that helps to act under prevailing uncertainty about what is being managed and the impacts of actions (Kumar et al., 2019). It is an effective tool to manage SAI in landscapes and has the benefit of addressing the knowledge management gaps. Sustainability assessment (Table 1) could also be linked to the adaptive management matrix used across scales and thus help to keep improve connectivity and manage trade-offs. Notably, at present this practice is lacking in many SSA countries (Haileslassie et al., 2020b).
Conclusions
There are several critiques and issues pertaining to the science and practices of sustainable agricultural intensification. The current practices fail to link sustainability assessment with context-specific SIPs design. Methodological options that consider multiple scales—addressing emerging issues and opportunities, related to scarce water resources—are limited. A consideration of AWM at the core of SIP development is critically absent, as is the linkage of SAI with the larger FS. In many cases, there is a paucity of rigorous efforts of trade-off management through targeting strategic entry points, such as water allocation and monitoring and recognition of multiple values of water, that leverage complementarity and synergies of interventions.
Within this context, this study offers three contributions: first, it synthesized and provided insights into the gaps in the science and practice of SAI in SSA and in the framework of water resources management. Secondly, a methodological framework is proposed that connects sustainability assessment methods to participatory SIPs design and adaptive management approach at multiple scales; furthermore, it illustrates how the co-design of sustainability domains and indicators can help in AWM centered SIP development. Third, the study proposes approaches to maneuver the heterogeneity of biophysical and social settings (in tailoring the technology options to context) along with the SIP design principles to enable synergies and complementarities of the SAI measures. The knowledge generated, and the methods proposed by this review can help researchers in integrating sustainability assessment and design of SIPs. The study can also guide policymakers and practitioners on how best to plan, implement, and monitor sustainable intensification initiatives such as Framework for Irrigation Development and Agricultural Water Management in Africa across multiple scales.
Author contributions
AH: conceptualization. WM: review of literature. AH and WM: methodology and writing original draft. SU, EL, and PS: review and editing. All authors have read and agreed to the final version of the manuscript. All authors contributed to the article and approved the submitted version.
Funding
The authors are grateful for financial support from the Coordinated Research Program Water, Land and Ecosystem (WLE) of the CGIAR and Growth for Future Program II: Natural Resources Management for Resilience and Economic Development in Rural Ethiopia project funded by the Swedish International Development Cooperation Agency (SIDA) and Water Security and Sustainable Development Hub funded by the UK Research and Innovation's Global Challenges Research Fund (GCRF) (Grant Number: ES/S008179/1).
Acknowledgments
The authors are grateful to Dr. Fitsum Hagos and Dr. Meron Teferi for reviewing the original draft. We are also thankful to participants of the high-level partners forum of Growth for Future Program II: Natural Resources Management for Resilience and Economic Development in Rural Ethiopia project, for their constructive comments.
Conflict of interest
The authors declare that the research was conducted in the absence of any commercial or financial relationships that could be construed as a potential conflict of interest.
Publisher's note
All claims expressed in this article are solely those of the authors and do not necessarily represent those of their affiliated organizations, or those of the publisher, the editors and the reviewers. Any product that may be evaluated in this article, or claim that may be made by its manufacturer, is not guaranteed or endorsed by the publisher.
References
Abberton, M., Batley, J., Bentley, A., Bryant, J., Cai, H., Cockram, J., et al. (2016). Global agricultural intensification during climate change: a role for genomics. Plant Biotechnol. J. 14, 1095–1098. doi: 10.1111/pbi.12467
Abrams, L. (2018). Unlocking the Potential of Enhanced Rain-Fed Agriculture. Stockholm: SIWI. Available online at: https://siwi.org/wp-content/uploads/2018/12/Unlocking-the-potential-of-rainfed-agriculture-2018-FINAL.pdf
Altchenko, Y., and Villholth, K. G. (2015). Mapping irrigation potential from renewable groundwater in Africa - a quantitative hydrological approach hydrol. Earth Syst. Sci. 19, 1055–1067. doi: 10.5194/hess-19-1055-2015
Assegide, E., Alamirew, T., Bayabil, H., Dile, Y. T., Tessema, B., and Zeleke, G. (2022). Impacts of surface water quality in the Awash River Basin, Ethiopia: a systematic review. Front. Water 3, 790–900. doi: 10.3389/frwa.2021.790900
AU (2013). AGENDA 2063 - A Shared Strategic Framework for Inclusive Growth and Sustainable Development. Addis Ababa: African Union.
AU (2020). Framework for Irrigation Development and Agricultural Water Management in Africa. Addis Ababa: African Union. Available online at: https://au.int/sites/default/files/documents/38632-doc-framework_for_irrigation_development_english.pdf
Barrett, C. B. (2008). “Poverty traps and resource dynamics in smallholder agrarian systems” in Economics of Poverty, Environment, and Natural Resource Use, eds A. Ruijs and R. Dellink (New York, NY: Springer). p. 17–40.
Baulcombe, D., Crute, I., Davies, B., Dunwell, J., Gale, M., Jones, J., et al. (2009). Reaping the Benefits: Science and the Sustainable Intensification of Global Agriculture. London: Royal Society.
Bindraban, P. S., and Rabbinge, R. (2012). Mega trends in agriculture- views for discontinuities in past and future developments. Glob. Food Sec. 1, 99–105. doi: 10.1016/j.gfs.2012.11.003
Bird, J. (2014). Game changers for irrigated agriculture-do the right incentives exist? Irrig. Drain. 63, 146–153. doi: 10.1002/ird.1838
Birge, H. E., Allen, C. R., Garmestani, A. S., and Pope, K. L. (2016). Adaptive management for ecosystem services. J. Environ. Manage. 183, 343–352. doi: 10.1016/j.jenvman.2016.07.054
Boretti, A., and Rosa, L. (2019). Reassessing the projections of the World Water Development Report. NPJ Clean Water 2, 1–5. doi: 10.1038/s41545-019-0039-9
Castellini, C., Boggia, A., Cortina, C., dal Bosco, A., Paolotti, L., Novelli, E., et al. (2012). A multi-criteria approach for measuring the sustainability of different poultry production systems. J. Clean. Prod. 37, 192–201. doi: 10.1016/j.jclepro.2012.07.006
Chukalla, A. D., Reidsma, P., van Vliet, M. T. H., Silva, J. V., van Ittersum, M. K., Jomaa, S., et al. (2020). Balancing indicators for sustainable intensification of crop production at field and river basin levels. Sci. Total Environ. 71, 1–13. doi: 10.1016/j.scitotenv.2019.135925
Clement, F., Haileslassie, A., Ishaq, S., Blümmel, M., Murty, M. V. R., Samad, M., et al. (2011). Enhancing water productivity for poverty alleviation: role of capitals and institutions in the Ganga basin. Expl. Agric. 47, 133–151. doi: 10.1017/S0014479710000827
Cofie, O., and Amede, T. (2015). Water management for sustainable agricultural intensification and smallholder resilience in sub-Saharan Africa. Water Resour. Rural. Dev. 6, 3–11. doi: 10.1016/j.wrr.2015.10.001
Conway, G., Wilson, K., and Wilson, E. (2013). Sustainable Intensification: A New Paradigm for African Agriculture. A 2013 Montpellier Panel Report. London: Agriculture for Impact. Available online at: https://ag4impact.org/wp-content/uploads/2013/04/MP_0176_Report_Redesign_2016.pdf
Cook, S., Laura, S., Barbara, A., and Sarah, W. (2015). Sustainable Intensification Revisited. London: IIED. Available online at: https://pubs.iied.org/sites/default/files/pdfs/migrate/14651IIED.pdf
Davis, K., Ekboir, J., Mekasha, W., Ochieng, C. M., Spielman, D. J., and Zerfu, E. (2007). Strengthening agricultural education and training in Sub-Saharan Africa from an innovation systems perspective: case studies of Ethiopia and Mozambique. J. Agric. Educ. 14, 35–51. doi: 10.1080/13892240701820371
Degrande, A., Siohdjie, Y., Franzel, S., Asaah, E., Takoutsing, B., Tsobeng, A., et al. (2013). “Disseminating agroforestry innovations in Cameroon: are relay organizations effective?” in Agro-Ecological Intensification of Agricultural Systems in the African Highlands, eds B. Vanlauwe, P. van Asten, and G. Blomme (London: Routledge). p. 1–10.
Denison, R. F., Kiers, E. T., and West, S. A. (2003). Darwinian agriculture: when can humans find solutions beyond the reach of natural selection? Quart. Rev. Biol. 78, 145–168. doi: 10.1086/374951
FAO (2017). The Future of Food and Agriculture - Trends and Challenges. Rome: Food and Agriculture Organization.
Firbank, L. G., Attwood, S., Eory, V., Gadanakis, Y., Lynch, J. M., Sonnino, R., et al. (2018). Grand challenges in sustainable intensification and ecosystem services. Front. Sustain. Food Syst. 2, 1–7. doi: 10.3389/fsufs.2018.00007
Fischer, R. A., and Edmeades, G. O. (2010). Breeding and cereal yield progress. Crop Sci. 50, 85–98. doi: 10.2135/cropsci2009.10.0564
Gadanakis, Y., Bennett, R., Park, J., and Areal, F. J. (2015). Evaluating the sustainable intensification of arable farms. J. Environ. Manage. 150, 288–298. doi: 10.1016/j.jenvman.2014.10.005
Gafsi, M., Legagneux, B., Nguyen, G., and Robin, P. (2006). Towards sustainable farming systems: effectiveness and deficiency of the French procedure of sustainable agriculture. Agric. Syst. 90, 226–242. doi: 10.1016/j.agsy.2006.01.002
Garnett, T., Appleby, M., Balmford, A., Bateman, I., Benton, T., Bloomer, P., et al. (2013). Sustainable intensification in agriculture: premises and policies. Science 341, 33–34. doi: 10.1126/science.1234485
Gasparatos, A., and Scolobig, A. (2012). Choosing the most appropriate sustainability assessment tool. Ecol. Econ. 80, 1–7. doi: 10.1016/j.ecolecon.2012.05.005
Gleick, P. H. (2003). Water use. Annu. Rev. Environ. Resour. 28, 275–314. doi: 10.1146/annurev.energy.28.040202.122849
Godfray, H. C. J., Beddington, J. R., Crute, I. R., Haddad, L., Lawrence, D., Muir, J. F., et al. (2010). Food security: the challenge of feeding 9 billion people. Science 327, 812–818. doi: 10.1126/science.1185383
Godfray, H. C. J., and Garnett, T. (2014). Food security and sustainable intensification. Philos. Trans. R. Soc. B. Biol. Sci. 369, 20120273. doi: 10.1098/rstb.2012.0273
Haileslassie, A., Blümmel, M., Murthy, M. V. R., Samad, M., Clement, F., Anandan, S., et al. (2011). Assessment of livestock feed and water nexus across mixed crop livestock system's intensification gradient: an example from the Indo-Ganaga Basin. Expl. Agric. 47, 113–132. doi: 10.1017/S0014479710000815
Haileslassie, A., Craufurd, P., Thiagarajah, R., Kumar, S., Whitbread, A., Rathor, A., et al. (2016). Empirical evaluation of sustainability of divergent farms in the dryland farming systems of India. Ecol. Indic. 60, 710–723. doi: 10.1016/j.ecolind.2015.08.014
Haileslassie, A., Ludi, E., Roe, M., and Button, C. (2020a). “Water values: discourses and perspective” in Clean Water and Sanitation (Encyclopedia of the UN Sustainable Development Goals), eds W. Leal Filho, A. M. Azul, L. Brandli, A. Lange Salvia, and T. Wall (Cham: Springer). p. 1–10.
Haileslassie, A., Mekuria, W., Schmitter, P., Uhlenbrook, S., and Ludi, E. (2020b). Changing agricultural landscapes in Ethiopia: examining application of adaptive management approach. Sustainability 12, 8939. doi: 10.3390/su12218939
Haileslassie, A., Priess, J., Veldkamp, E., and Lesschen, J. P. (2007). Nutrient flows and balances at the field and farm scale: exploring effects of land-use strategies and access to resources. Agric. Syst. 94, 459–470. doi: 10.1016/j.agsy.2006.11.013
Hallstrom, E., Carlsson-Kanyama, A., and Borjesson, P. (2015). Environmental impact of dietary change: a systematic review. J. Clean. Prod. 91, 1–11. doi: 10.1016/j.jclepro.2014.12.008
Heink, U., and Kowarik, I. (2010). What are indicators? On the definition of indicators in ecology and environmental planning. Ecol. Indic. 10, 584–593. doi: 10.1016/j.ecolind.2009.09.009
Hoang, L. A., Castella, J. C., and Novosad, P. (2006). Social networks and information access: implications for agricultural extension in a rice farming community in northern Vietnam. Agric. Hum. Values 23, 513–527. doi: 10.1007/s10460-006-9013-5
Hugé, J., Waas, T., Dahdouh-Guebas, F., Koedam, N., and Block, T. (2013). A discourse-analytical perspective on sustainability assessment: interpreting sustainable development in practice. Sustain. Sci. 8, 187–198. doi: 10.1007/s11625-012-0184-2
Kamanga, B. C. G., Waddington, S. R., Robertson, M. J., and Giller, K. E. (2010). Risk analysis of maize-legume crop combinations with smallholder farmers varying in resource endowment in central Malawi. Exp. Agric. 46, 1–21. doi: 10.1017/S0014479709990469
Kemp, D. R., Guodong, H., Xiangyang, H., Michalk, D. L., Fujiang, H., Jianping, W., et al. (2013). Innovative grassland management systems for environmental and livelihood benefits. Proc. Natl. Acad. Sci. U.S.A. 110, 8369–8374. doi: 10.1073/pnas.1208063110
Kremen, C., Iles, A., and Bacon, C. (2012). Diversified farming systems: an agroecological, systems-based alternative to modern industrial agriculture. Ecol. Soc. 17, 44. doi: 10.5751/ES-05103-170444
Kumar, S., Craufurd, P., Haileslassie, A., Thiagarajah, R., and Whitbread, A. (2019). Farm typology analysis and technology assessment: an application in an arid region of South Asia. Land Use Policy 8, 104–149. doi: 10.1016/j.landusepol.2019.104149
Lemaire, G., Franzluebbers, A., Carvalho, P. C. F., and Dedieu, B. (2014). Integrated crop-livestock systems: strategies to achieve synergy between agricultural production and environmental quality. Agric. Ecosyst. Environ. 190, 4–8. doi: 10.1016/j.agee.2013.08.009
Li, X., and Troy, T. J. (2018). Changes in rain fed and irrigated crop yield response to climate in the western US. Environ. Res. Lett. 13, 064031. doi: 10.1088/1748-9326/aac4b1
Lobell, D. B., Cassman, K. G., and Field, C. B. (2009). Crop yield gaps: their importance, magnitude and causes. Annu. Rev. Environ. Resour. 34, 179–204. doi: 10.1146/annurev.environ.041008.093740
MacDonald, G. K., D'Odorico, P., and Seekell, D. A. (2016). Pathways to sustainable intensification through crop water management. Environ. Res. Lett. 11, 091001. doi: 10.1088/1748-9326/11/9/091001
Macfadyen, S., Tylianakis, J. M., Letourneau, D. K., Benton, T. G., Tittonell, P., Perring, M. P., et al. (2015). The role of food retailers in improving resilience in global food supply. Glob. Food Secur. 7, 1–8. doi: 10.1016/j.gfs.2016.01.001
Masters, W. A., Djurfeldt, A. A., De Haan, C., Hazell, P., Jayne, T., et al. (2013). Urbanization and farm size in Asia and Africa: implications for food security and agricultural research. Glob. Food Sec. 2, 156–165. doi: 10.1016/j.gfs.2013.07.002
Mastrangelo, M. E., and Gavin, M. C. (2012). Trade-offs between cattle production and bird conservation in an agricultural frontier of the Gran Chaco of Argentina. Conserv. Biol. 26, 1040–1051. doi: 10.1111/j.1523-1739.2012.01904.x
Mekuria, W., Diyasa, M., Tengberg, A., and Haileslassie, A. (2021). Effects of long-term land use and land cover changes on ecosystem service values: an example from the central rift Valley, Ethiopia. Land 10, 1373. doi: 10.3390/land10121373
Meul, M., van Passel, S., Nevens, F., Dessein, J., Rogge, E., Mulier, A., et al. (2008). MOTIFS: a monitoring tool for integrated farm sustainability. Agron. Sustain. Dev. 28, 321–332. doi: 10.1051/agro:2008001
Musumba, M., Grabowski, P., Palm, C., and Snapp, S. (2017). Guide for the Sustainable Intensification Assessment Framework. Manhattan: USAID and Kansas State University.
Mutambara, S., Darkoh, M. B. K., and Atlhopheng, J. R. (2016). A comparative review of water management sustainability challenges in smallholder irrigation schemes in Africa and Asia. Agric. Water Manage. 171, 63–72 doi: 10.1016/j.agwat.2016.03.010
Mutyasira, V., Hoag, D., Pendell, D., Manning, D. T., and Berhe, M. (2018). Assessing the relative sustainability of smallholder farming systems in Ethiopian highlands. Agric. Syst. 167, 83–91. doi: 10.1016/j.agsy.2018.08.006
Nakawuka, P., Langan, S., Schmitter, P., and Barron, J. (2018). A review of trends, constraints and opportunities of smallholder irrigation in East Africa. Global Food Secur. 17, 196–212. doi: 10.1016/j.gfs.2017.10.003
Nicol, A., Langan, S., Victor, M., and Gonsalves, J. (2015). Water-Smart Agriculture in East Africa. Colombo: International Water Management Institute.
Pereira, L. S., Cordery, I., and Iacovides, I. (2012). Improved indicators of water use performance and productivity for sustainable water conservation and saving. Agric. Water Manage. 108, 39–51. doi: 10.1016/j.agwat.2011.08.022
Phalan, B., Balmford, A., Green, R. E., and Scharlemann, J. P. (2011). Minimizing the harm to biodiversity of producing more food globally. Food Policy 36, 62–71. doi: 10.1016/j.foodpol.2010.11.008
Pretty, J., Attwood, S., Bawden, R., van Den Berg, H., Bharucha, Z. P., Dixon, D., et al. (2020). Assessment of the growth in social groups for sustainable agriculture and land management. Global Sustain. 23, 1–16. doi: 10.1017/sus.2020.19
Pretty, J., Benton, T. G., Bharucha, Z. P., Dicks, L. V., Flora, C. B., Godfray, H. C. J., et al. (2018). Global assessment of agricultural system redesign for sustainable intensification. Nat. Sustain. 1, 441–446. doi: 10.1038/s41893-018-0114-0
Pretty, J., Toulmin, C., and Williams, S. (2011). Sustainable intensification in African agriculture. Int. J. Agric. Sustain. 9, 5–24. doi: 10.3763/ijas.2010.0583
Pywell, R., Heard, M., Woodcock, B., Hinsley, S., Ridding, L., Nowaklowski, M., et al. (2015). Wildlife-friendly farming increases crop yield: evidence for ecological intensification. Proc. R. Soc. B Biol. Sci. 282, 20151740. doi: 10.1098/rspb.2015.1740
Rivera, M., Knickel, K., Díaz-Puente, J. M., and Afonso, A. (2019). The role of social capital in agricultural and rural development: lessons learnt from case studies in seven countries. ?Sociol. Rural. 59, 66–91. doi: 10.1111/soru.12218
Rosegrant, M. W., Ringler, C., and Zhu, T. (2009). Water for agriculture: maintaining food security under growing scarcity. Annu. Rev. Environ. Resour. 34, 205–222. doi: 10.1146/annurev.environ.030308.090351
Rosegrant, M. W., Tokgoz, S., and Bhandary, P. (2013). The new normal? A tighter global agricultural supply and demand relation and its implications for food security. Am. J. Agric. Econ. 95, 303–309. doi: 10.1093/ajae/aas041
Sala, S., Ciuffo, B., and Nijkamp, P. (2015). A systemic framework for sustainability assessment. Ecol. Econ. 119, 314–325. doi: 10.1016/j.ecolecon.2015.09.015
Shackelford, G. E., Steward, P. R., German, R. N., Sait, S. M., and Benton, T. G. (2015). Conservation planning in agricultural landscapes: hotspots of conflict between agriculture and nature. Divers. Distrib. 21, 357–367. doi: 10.1111/ddi.12291
Smith, A., Snapp, S., Chikowo, R., Thorne, P., Bekunda, M., and Glover, J. (2017). Measuring sustainable intensification in smallholder agroecosystems: a review. Global Food Sec. 12, 127–138. doi: 10.1016/j.gfs.2016.11.002
Southern, A., Lovett, A., O'Riordan, T., and Watkinson, A. (2011). Sustainable landscape governance: lessons from a catchment-based study in whole landscape design. Landscape Urban Plann. 101, 179–189. doi: 10.1016/j.landurbplan.2011.02.010
Steiner, A., Aguilar, G., Bomba, K., Bonilla, J. P., Campbell, A., Echeverria, R., et al. (2020). Actions to Transform Food Systems Under Climate Change. Wageningen: CGIAR Research Program on Climate Change, Agriculture and Food Security.
Struik, P. C., and Kuyper, T. W. (2017). Sustainable intensification in agriculture: the richer shade of green. A review. Agron. Sustain. Dev. 37, 39. doi: 10.1007/s13593-017-0445-7
Sutton, M. A., Bleeker, A., Howard, C. M., Bekunda, M., Grizzetti, B., de Vries, W., et al. (2013). Our Nutrient World: The Challenge to Produce More Food and Energy With Less Pollution. Edinburgh: Edinburgh NERC; Centre for Ecology & Hydrology.
Teklu, B. M., Haileslassie, A., and Mekuria, W. (2022). Pesticides as water pollutants and level of risks to environment and people: an example from Central Rift Valley of Ethiopia. Environ. Dev. Sustain. 24, 5275–5294. doi: 10.1007/s10668-021-01658-9
Thrupp, L. A. (2000). Linking agricultural biodiversity and food security: the valuable role of agrobiodiversity for sustainable agriculture. Int. Aff. 76, 283–297. doi: 10.1111/1468-2346.00133
Townsend, T. J., Ramsden, S. J., and Wilson, P. (2016). How do we cultivate in England? Tillage practices in crop production systems. Soil Use Manage. 32, 106–117. doi: 10.1111/sum.12241
van Ittersum, M. K., Kenneth, C., Grassini, P., Wolf, J., Tittonell, P., and Hochman, Z. (2013). Yield gap analysis with local to global relevance-a review. Field Crops Res. 143, 4–17. doi: 10.1016/j.fcr.2012.09.009
van Ittersum, M. K., Van Bussel, L. G., Wolf, J., Grassini, P., Van Wart, J., and Guilpart, N. (2016). Can Sub-Saharan Africa feed itself? PNAS 113, 14964–14969. doi: 10.1073/pnas.1610359113
Vanschoenwinkel, J., and Passel, S. V. (2018). Climate response of rain fed versus irrigated farms: the bias of farm heterogeneity in irrigation. Clim. Change 147, 225–234. doi: 10.1007/s10584-018-2141-2
Vidal, A., van Koppen, B., and Blake, D. (2009). The green-to-blue water continuum: an approach to improve agricultural systems' resilience to water scarcity. On the Water Front. 1, 66–72.
Weltin, M., Zasada, I., Piorr, A., Debolini, M., Geniaux, G., Perez, O. M., et al. (2018). Conceptualizing fields of action for sustainable intensification - a systematic literature review and application to regional case studies. Agric. Ecosyst. Environ. 257, 68–80. doi: 10.1016/j.agee.2018.01.023
Xie, H., Huang, Y., Chen, Q., Zhang, Y., and Wu, Q. (2019). Prospects for agricultural sustainable intensification: a review of research. Land 8, 157. doi: 10.3390/land8110157
Yami, M., and van Asten, P. (2017). Policy support for sustainable crop intensification in Eastern Africa. J. Rural Stud. 55, 216–226. doi: 10.1016/j.jrurstud.2017.08.012
You, L., Ringler, C., Wood-Sichra, U., Robertson, R., Wood, S., Zhu, T., et al. (2011). What is the irrigation potential for Africa? A combined biophysical and socioeconomic approach. Food Policy 36, 770–782. doi: 10.1016/j.foodpol.2011.09.001
Keywords: agricultural water management, ecosystem services, sustainable intensification, food systems, multiple scales
Citation: Haileslassie A, Mekuria W, Uhlenbrook S, Ludi E and Schmitter P (2022) Gap analysis and methodological framework to assess and develop water centric sustainable agricultural intensification pathways in Sub-Saharan Africa. Front. Water 4:747610. doi: 10.3389/frwa.2022.747610
Received: 26 July 2021; Accepted: 30 September 2022;
Published: 18 October 2022.
Edited by:
Mariele Evers, University of Bonn, GermanyReviewed by:
Faissal Aziz, Cadi Ayyad University, MoroccoBrighton Austin Chunga, Mzuzu University, Malawi
Copyright © 2022 Haileslassie, Mekuria, Uhlenbrook, Ludi and Schmitter. This is an open-access article distributed under the terms of the Creative Commons Attribution License (CC BY). The use, distribution or reproduction in other forums is permitted, provided the original author(s) and the copyright owner(s) are credited and that the original publication in this journal is cited, in accordance with accepted academic practice. No use, distribution or reproduction is permitted which does not comply with these terms.
*Correspondence: Amare Haileslassie, YS5oYWlsZXNsYXNzaWUmI3gwMDA0MDtjZ2lhci5vcmc=