- Department of Geography and Environmental Sustainability, State University of New York College at Oneonta, Oneonta, NY, United States
Located at the eastern extent of the Great Lakes snowbelt, Central New York averages some of the highest annual snowfall totals east of the Rocky Mountains. This is in large part due to the variety of snowstorms that affect the region including lake-effect storms, coastal storms, and overrunning storms. Previous estimates suggest that lake-effect snowstorms account for approximately half of the seasonal snow in the Great Lakes basin, but ignore the spatial variability that exists within the region. Therefore, this study examines the seasonal snowfall contributions of the different snowstorm types to affect Central New York. Results suggest that although lake-effect snowstorms are the dominant snowstorm type in the region, their seasonal snowfall contributions vary between 13 and 48%. Although lake-effect snowstorms produce more snow during the peak and mid-seasons, their relative contribution is greatest during the early and mid-winter seasons. Generally, higher contributions occur near the Tug Hill Plateau, with lower contributions in southern Central New York. Instead, snowfall in southern Central New York is mostly dominated by Nor'easters (16–35%), with lesser contributions from Rocky lows (14–29%). Overrunning storms that originate in Canada (e.g., Alberta clippers) and non-cyclonic storms contribute the least to seasonal snowfall totals across Central New York; however, they are often the catalyst for lake-effect snowstorms in the region, as they advect continental polar air masses that destabilize across the lake. Understanding the actual snowfall contribution from different snowstorm types is needed for future climate predictions. Since the potential trajectory of future snowfall varies according to the type of storm, climate models must accurately predict the type of storm that is producing the snow.
Introduction
Snow is an integral part of the environment and society of high latitudes and high elevations (Rooney, 1967; Sharratt et al., 1992; Kocin and Uccellini, 2004; Cortinas and Kitron, 2006; Rohr et al., 2011; Hagenstad et al., 2018). This is especially prevalent in areas surrounding the Laurentian Great Lakes, which experience some of the greatest seasonal snowfall totals in North America. The presence of the Great Lakes considerably affects the climate of this region, especially during cold-season months (October–May). As cold air advects across the relatively warmer lakes, there is a destabilization of the air column and an influx of moisture (Peace and Sykes, 1966; Niziol, 1987; Pease et al., 1988). This leads to frequent, and sometimes intense lake-effect precipitation, downwind of the lakes (e.g., Niziol et al., 1995).
Lake-effect precipitation occurs approximately one out of every five days in the early winter season (September–November) of the Great Lakes basin (Miner and Fritsch, 1997). Precipitation is predominately rain from September through October, but often transitions to snow following the first week of November. Lake-effect storms become more frequent as the season progresses, peaking in early January (Niziol et al., 1995). Due to their relative frequency, it is estimated that lake-effect snowstorms contribute anywhere from 20 to 80% of the seasonal snowfall in the Great Lakes basin (Eichenlaub, 1970; Miner and Fritsch, 1997; Liu and Moore, 2004; Veals and Steenburgh, 2015). Eichenlaub (1970) suggests that lake-effect storms contribute at least 20% of the seasonal snowfall in areas lee of Lake Superior and Lake Michigan. This estimate is modest, compared to those from other studies that suggest contributions are closer to 50% in other subregions of the Great Lakes basin and during more recent years (Kelly, 1986; Braham and Dungey, 1995; Liu and Moore, 2004; Hartnett, 2020; Pettersen et al., 2020; Suriano and Wortman, 2021).
The influence of lake-effect snowstorms varies across the Great Lakes region. Multiple researchers have noted that seasonal snowfall is highly influenced by a location's elevation, and proximity and orientation to the lakes (e.g., Hjelmfelt, 1992; Scott and Huff, 1996; Perry and Konrad, 2006; Hartnett et al., 2014; Campbell et al., 2016). In general, areas downwind (east) of the lakes tend to be snowier than those upwind (west). For example, Changnon (1968) and Gatz and Changnon (1976) suggest that lake-effect snowstorms increase snowfall by up to 100% on the downwind shores of Lake Michigan compared to the upwind shores, especially in December. A more modest increase of 20–35% was found by subsequent studies conducted within the region (Kelly, 1986; Braham and Dungey, 1995; Scott and Huff, 1996, 1997). Some of the snowiest regions within the Great Lakes basin are found to the lee (downwind) of Lake Ontario, including the Tug Hill Plateau of New York State (further referred to as the Tug Hill). Using different approaches, the recent studies of Hartnett (2020) and Suriano and Wortman (2021) suggest that lake-effect snowstorms account for ~35–42% of the snowstorm events to the lee of Lake Ontario, yet contribute between 42 and 48% of the seasonal snowfall. The percent contribution is even greater within the Tug Hill, as Veals and Steenburgh (2015) estimate that lake-effect snow accounts for ~61–76% of the mean cool-season snowfall. The greater percentages in the Tug Hill are likely due to the more frequent and more intense lake-effect snowstorms that occur because of the land-breeze fronts that form along the southeastern shore of Lake Ontario, the localized ascent along the frontal boundary, and the intensified and broadened ascent regions induced by the Tug Hill (Campbell and James Steenburgh, 2017).
Although lake-effect snowstorms are found to dominate snowfall in this region, they are not the only snowstorm type to contribute to seasonal totals. Karmosky (2007) and Suriano et al. (2019) suggest that three distinctive weather patterns tend to produce snowfall in upstate New York including coastal mid-latitude cyclones, overrunning systems, and lake-effect or Great Lakes enhanced storms. Suriano et al. (2019) found that overrunning systems and coastal mid-latitude cyclones account for ~55% of the seasonal snowfall in Catskill/Delaware Watershed. Hartnett (2020) found 11 different snowstorm types that contribute to seasonal snowfall totals in central New York State. These storms included clippers, Colorado lows, frontal storms, Great Lakes lows, Hudson lows, lake-effect snowstorms, Nor'easters, Oklahoma hooks, Texas hooks, tropical cyclones, and upper atmospheric disturbances. Similar to Kelly (1986), the author found that non-lake effect snowstorms occur more frequently than lake-effect snowstorms, with Nor'easters and clippers contributing ~11.3 and 9.2%, respectively, of the seasonal snowfall between 1985 and 2015. However, distinguishing lake-effect snow from synoptically driven snow is complicated by mesoscale lake snow bands within large-scale cyclonic storms (e.g., Houze and Hobbs, 1982; Kristovich et al., 2000; Tardy, 2000; Owens et al., 2017; Kulie et al., 2021). This can result in additional snowfall, which is termed lake-enhanced snow (Eichenlaub and Hodler, 1979; Liu and Moore, 2004).
To date, most studies assessing snowfall contributions to the lee of Lake Ontario only focus on snowfall from lake-effect storms. Additionally, most studies use proxies to identify lake-effect snow versus non-lake-effect snow, such as synoptic patterns (Suriano et al., 2019; Suriano and Wortman, 2021) or cloud base heights (Pettersen et al., 2020), rather than a complete analysis of each individual snowstorm. There also is little attention given to the spatial variability that exists within the Lake Ontario basin, even though it is well document that lake-effect snow contributions decrease further from the Great Lakes (Dewey, 1970; Scott and Huff, 1996, 1997; Suriano et al., 2019). Snowstorms, especially lake-effect snowstorms, can produce extremely localized snow (e.g., Andersson and Nilsson, 1990; Steenburgh et al., 2000; Eito et al., 2005; Laird et al., 2009; Kindap, 2010). Relatively small topographic features such as hills and plateaus downwind of the lakes can dramatically increase snowfall totals (Hill, 1971; Hjelmfelt, 1992; Campbell and James Steenburgh, 2017). The objectives of this study are to provide the first comprehensive examination of snowstorms affecting the Lake Ontario basin and to determine how snowfall contributions from various storms varies spatially across the region. Since snowfall trends vary between lake-effect and non-lake-effect snowstorms (Norton and Bolsenga, 1993; Leathers and Ellis, 1996; Burnett et al., 2003; Kunkel et al., 2009a; Bard and Kristovich, 2012; Hartnett et al., 2014; Notaro et al., 2015; Suriano and Leathers, 2016), teasing out the snowfall contributions of different snowstorm types will help to better understand potential future changes.
Methods
Snowstorm Categorization
Seasonal snowfall contributions were determined by identifying every snowstorm to influence central New York State (Figure 1) from the 1995/96 to 2014/15 cold seasons (October to May). Snowstorms were identified using data from the National Weather Service's Cooperative Observer Program (COOP) and are defined as any storm in which at least 0.1 cm of snow was observed for at least two of the two-hundred and two reporting stations within the study area (Perry et al., 2007; Hartnett, 2020; Figure 2). Using the methods outlined by Hartnett (2020), a storm was considered independent from other events if there was at least a 6-h gap in precipitation (e.g., 6-h gap in 15-min precipitation records and/or radar) anywhere within the study area. There were 1,285 snowstorms identified during the study period. Daily snowfall totals for the 202 reporting COOP stations were assigned to individual storms. In some instances, a single storm spanned multiple days, and therefore its snowfall total represents the summation of multiple daily snowfall observations. Due to snowfall measurement practices (Doesken and Judson, 1996; NWS, 2012), limitations exist on the temporal resolution of snowfall observations. Thus, it is assumed that a single storm produces the entire daily snowfall total observed on a day. Although there may be inaccuracies that emerge from this assumption (Wu et al., 2005; Daly et al., 2007; Kunkel et al., 2007; Leeper et al., 2015; Lundquist et al., 2015), it is an adequate method of interpretation and has been partly addressed later in this study with the inclusion of lake snow.
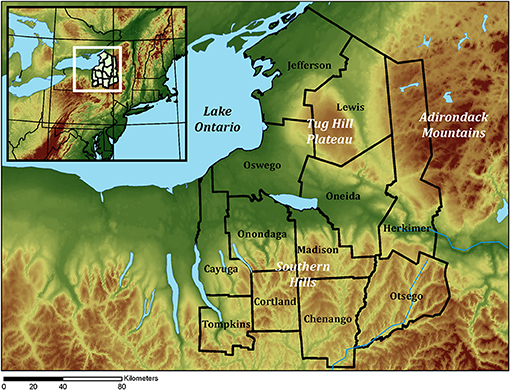
Figure 1. Central New York study area. Included are the county names (black text) and prominent regions (white text).
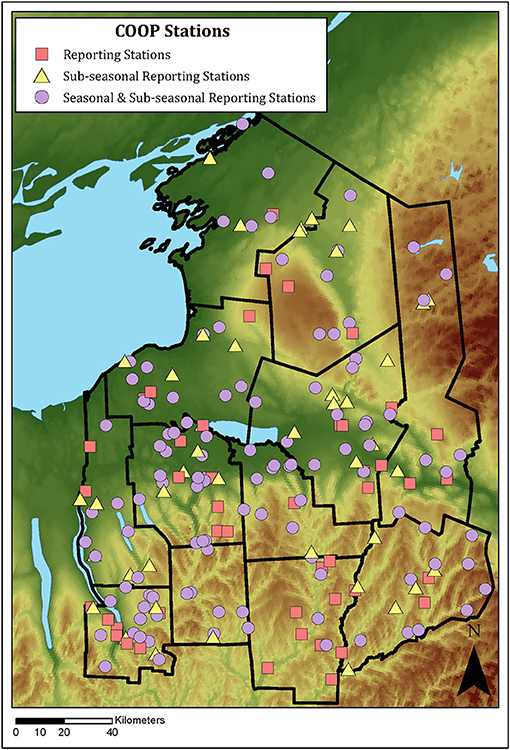
Figure 2. COOP stations used for analysis in Central New York. Included are the 202 COOP stations reporting from 1995/96 to 2014/15 (“Reporting Stations”), 155 stations used for the sub-seasonal analysis (“Sub-seasonal Reporting Stations”), and the 111 stations used for seasonal analyses (“Seasonal and Sub-seasonal Reporting Stations”).
Following the methods and classification system outlined by Hartnett (2020), snowstorms were categorized into storm types using NCEP/NCAR reanalyzes and NEXRAD data. Due to a lack of NEXRAD data prior to the 1995/96 season, snowstorm observations are limited to the establishment of the Binghamton, NY (KBGM) and Montague/Ft. Drum (KTYX) radar sites. Lake-effect snowstorms were delineated as any storm lacking a central low pressure within 150 km of the study area and a quasi-stationary banded precipitation pattern connected to Lake Ontario that strengthened downwind of the lake and was independent from other mesoscale cloud structures. If these conditions were not met, yet there was no central low pressure within 150 km of Central New York, the storm was classified as a non-cyclonic snowstorm. Cyclonically driven snowstorms are those with a central low pressure within 150 km of the study area. These storms were further classified based on their zone of cyclogenesis and grouped into one of three categories: Nor'easters, Canadian lows (Clippers, Hudson lows, and Great Lakes lows), and Rocky lows (Colorado lows, Oklahoma hooks, and Texas hooks). Snowstorms originating as tropical storms were omitted from this study due to their infrequency (only one storm during the study period). Snowstorm counts for the five categories area found in Table 1.
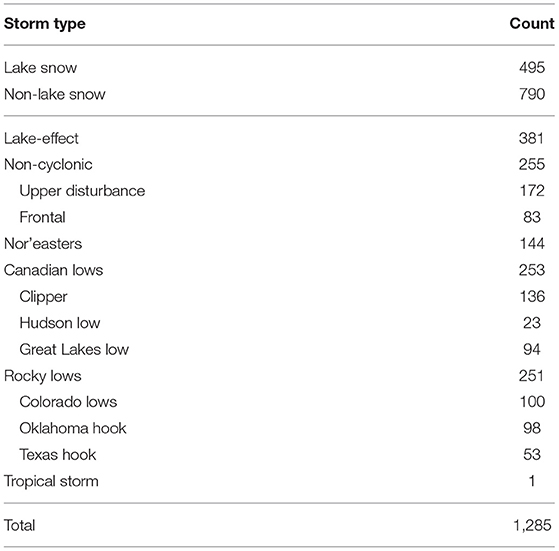
Table 1. Number of snowstorms per storm type to affect Central New York from the 1995/96 season to the 2014/15 season.
Since there are instances where lake-enhanced snow bands occur during cyclonic storms (Eichenlaub and Hodler, 1979; Liu and Moore, 2004; Owens et al., 2017; Kulie et al., 2021), storms were also categorized as lake snowstorms and non-lake snowstorms (Table 1). Lake snowstorms attempt to account for lake-enhanced snowfall events and are categorized similar to lake-effect snowstorms; however, precipitation and cloud structure does not have to be independent from other mesoscale storms and there does not have to be a six-hour gap in precipitation between storm events (Hartnett, 2020). Non-lake snowstorms are all other storms. These categories are mutually exclusive from each other, but not from the other storm types. For example, a storm can be classified as both a Nor'easter and a lake snowstorm but cannot be classified as both a lake snowstorm and a non-lake snowstorm. The methods presented in this study are a more direct analysis of snowstorms, expanding upon the procedures outlined by Pettersen et al. (2020) and Suriano and Wortman (2021) who use proxies to distinguish lake-effect snow versus non-lake-effect snow.
Total Seasonal Snowfall Contributions
To determine the seasonal snowfall contributions of the different snowstorm types within Central New York, total monthly snowfall for each station was calculated for cold-season months from July 1995 to June 2015. A station's monthly snowfall total was calculated by summing the snowfall produced at that station by each snowstorm that occurred during that month. Since these are voluntary data, there are instances where snowfall measurements are missing for some snowstorms, most notably, during light snowfall events. Therefore, using the guidance of Kunkel et al. (2009b), a station's monthly snowfall total was only used if snowfall observations were reported for at least 90% of the monthly snowstorms during that month. Data were removed for all months that did not contain any snowstorms.
Prior to calculating snowfall contributions, seasonal snowfall totals for each station were calculated by summing the monthly snowfall totals for that station across the snowfall season, which was defined as July 1–June 30. A station's seasonal snowfall total was only reported if monthly observations existed for every cold season month (October–May). To increase the spatial coverage of observations, any station with at least one season that met the above criteria were used for analysis (111 stations; Figure 2). Using the 111 available stations, interpolated surfaces were created for every snowfall season from 1995/96 to 2014/15. Surfaces were created using simple cokriging interpolations in ArcGIS with elevation as an independent variable (Guan et al., 2005; Grieser, 2015). The twenty (one per season) interpolated surfaces were then averaged in ArcGIS (Norton and Bolsenga, 1993), to produce a single map representing the average seasonal snowfall across Central New York (Figure 3). This figure provides a reference to compare the percent contributions.
To address the percent contribution of the different snowstorm types to seasonal snowfall totals within Central New York, seasonal totals for each storm type were calculated for the 111 stations. To compare snowfall totals across the study area and reduce biases in the results, the ratio of snowfall produced by each storm type to the total snowfall produced at a station was calculated. These values are referred to as the percent contributions. The percent contributions were then used to create interpolated surfaces for each storm type for every cold season from 1995/96 to 2014/15. The average snowfall contribution across the study area was calculated for each storm type by averaging the 20 interpolated surfaces (Figures 4, 5).
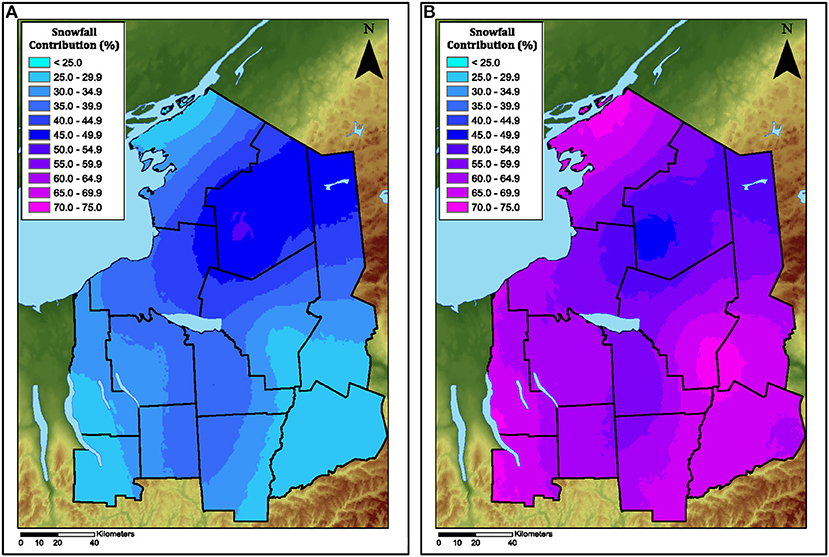
Figure 4. Seasonal snowfall contributions of lake snowstorms (A) and non-lake snowstorms (B) from 1995/96 to 2014/15.
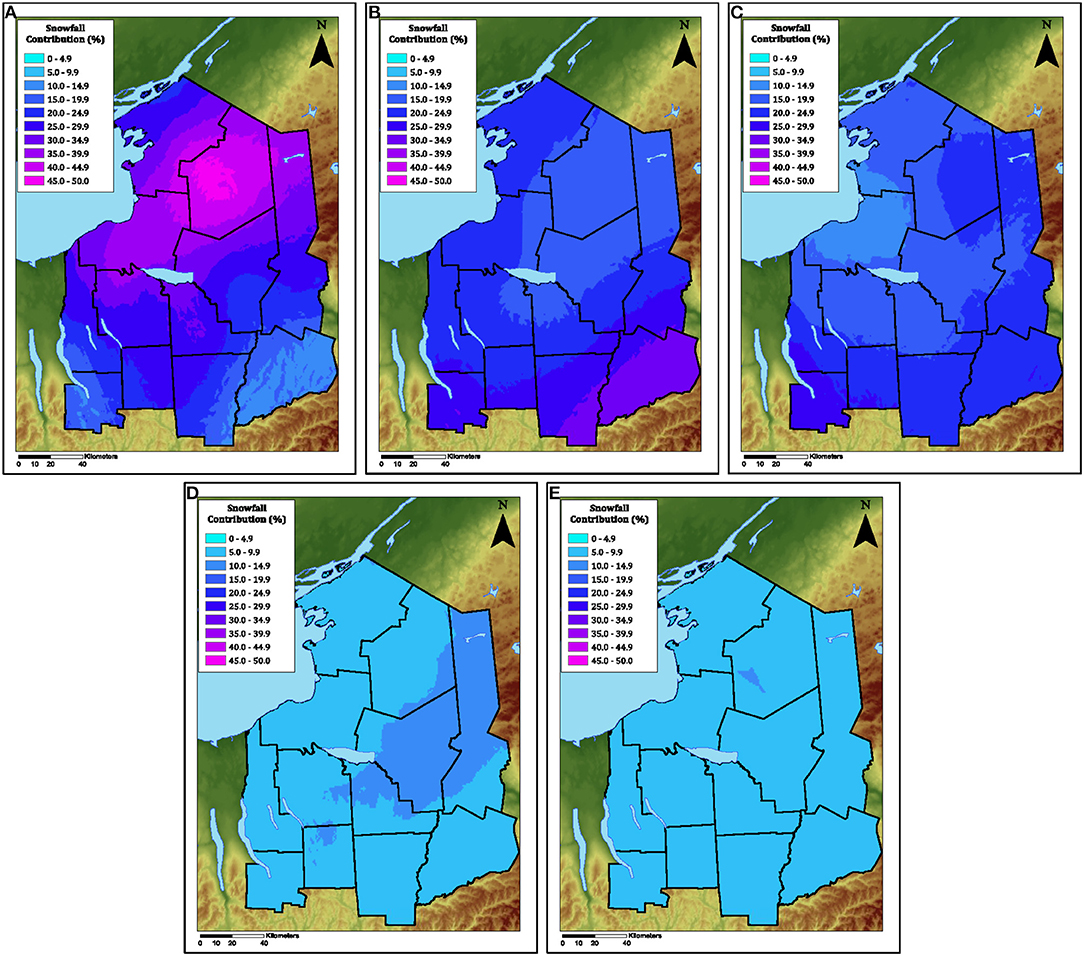
Figure 5. Seasonal snowfall contributions for the five different snowstorms to affect Central New York from 1995/96 to 2014/15. Figures include contributions from lake-effect storms (A), Nor'easters (B), Rocky lows (C), Canadian lows (D), and non-cyclonic storms (E).
Sub-seasonal Lake-Effect Snow Contributions
To address the seasonal variability in snowfall contributions from lake-effect storms that exists within a cold season, monthly totals were grouped into four sub-seasonal categories: early season (October to November), peak season (December to January), mid-season (February to March), and late season (April to May) (Niziol et al., 1995; Veals and Steenburgh, 2015; Clark et al., 2016). Monthly snowfall totals for each cold season calculated in “Total Seasonal Snowfall Contributions” Section were summed across the sub-seasonal scale for each COOP station. Sub-seasonal totals were only reported if that station had reliable records (snowfall reports for 90% of the monthly snowstorms) for both months (e.g., reliable data for December 1995 and January 1996). To increase the spatial density of observations, COOP stations were retained for analysis if there was at least one reliable sub-seasonal snowfall total during the study period. Thus, 155 COOP stations were used for analysis in this section (Figure 2). Sub-seasonal lake-effect snowfall contributions for each station were calculated by comparing the sub-seasonal snowfall totals from these storms to the total sub-seasonal snowfall at that station. Like “Total Seasonal Snowfall Contributions” Section, interpolated surfaces were created in ArcGIS for each sub-season. The 20 surfaces were averaged to produce two figures for each sub-season, one representing the average snowfall contribution total and the other representing the percent contribution of lake-effect snow across Central New York.
Results
Seasonal Snowfall Totals
The mean seasonal snowfall in Central New York from 1995/96 to 2014/15 is plotted in Figure 3. Seasonal snowfall totals are greatest (~500 cm) over the Tug Hill, with lower totals generally further from the region. Seasonal snowfall contributions of lake-snowstorms and non-lake-snowstorms are presented in Figure 4. Throughout the majority of Central New York (98.4%), non-lake snowstorms contribute more to these seasonal snowfall totals than lake snowstorms. A small section of the Tug Hill is the only location that receives more snowfall from lake snow than non-lake snow. Instead, the majority (77.8%) of the area receives between 30 and 50% of its seasonal snowfall from lake snowstorms, including areas downwind of Lake Ontario, the western Adirondack Mountains, and the Southern Hills. Lake snow contributions were least in areas furthest from and sub-parallel to the horizontal axis of Lake Ontario.
Seasonal Snowfall Contributions
To examine the spatial variation of seasonal snowfall contributions from different storm types, snowfall contributions were mapped for the five snowstorm types (Figure 5). While it is widely believed that lake-effect snowstorms are the dominant snowfall producer in Central New York (e.g., Burnett et al., 2003; Hall et al., 2017; Suriano et al., 2019; Hartnett, 2020), the figures show that this is not the case throughout the entire study area (Figure 5A). Lake-effect snow accounts for 35–48% of the seasonal snowfall over northern Central New York, whereas contributions are as low as 13–15% in southeastern Central New York. Lake-effect snowstorms for the majority (71.7%) of the region account for at least 20% of the seasonal snowfall.
Seasonal snowfall contributions of Nor'easters and Rocky lows are greatest for southern Central New York (Figures 5B,C). Although patterns are similar, there are noticeable differences in the spatial distribution of their contributions. Snowfall contributions from Nor'easters are greatest (30–35%) in southeastern Central New York, and generally decrease to the northwest (Figure 5B). Comparatively, there is a pronounced increase in the percent contribution (25–30%) from Rocky lows in southwestern Central New York and a noticeable decrease (10–15%) over Oswego County, just west of Lake Ontario (Figure 5C). It should be noted that these figures represent the percent contribution of a snowstorm to seasonal snowfall totals relative to the amount of snow a location receives. Therefore, even though Nor'easters only contribute between 15 and 20% of the annual snow in the Tug Hill, this region also averages ~200 cm of more snowfall than other areas in Central New York (Figure 3). Conversely, the greater snowfall contributions in southern and southeastern Central New York are in large part due to the lower seasonal snowfall totals. However, the use of percentages allows for comparisons between different areas regardless of their seasonal snowfall totals.
Seasonal snowfall contributions from Canadian lows and noncyclonic storms are more homogeneous throughout the study area (Figures 5D,E). Although there is some spatial variability that exists, it is not as prominent as the other three storm types. Snowfall contributions from Canadian lows are greatest (10–15%) over eastern Central New York, most notably around the western edge of the Adirondack Mountains (Figure 5D). Seasonal contributions from noncyclonic snowstorms exhibit a slightly higher contribution (10–15%) over a small area in Lewis County (Figure 5E).
Sub-seasonal Lake-Effect Snow Contributions
Multiple researchers have suggested that lake-effect snow exhibits sub-seasonal variability in its prevalence (Strommen and Harman, 1978; Niziol et al., 1995; Veals and Steenburgh, 2015; Clark et al., 2016, 2020; Fairman et al., 2016). Harrington and Dewey (1987) found that lake-effect snowstorms are most common from November to January, when the lake surface is the warmest, resulting in large surface to 850 hPa lapse rates and minimal ice cover. Therefore, cold seasons were divided into four time periods (early, peak, mid-, and late seasons) and contributions were averaged across the 20 seasons (Figure 6). The results suggest that lake-effect snowstorms contribute considerably more snowfall during the peak season and mid-season than during the early and late seasons (Figures 6A,C,E,G). Nontheless, lake-effect snow accounts for a greater percentage of the sub-seasonal snow during the early and peak-seasons (October–January) (Figures 6B,D,F,H). Spatial patterns were mostly consistent across the four sub-seasons, as lake-effect snowfall contributions were greatest directly east of Lake Ontario. However, there was a movement of the greatest snowfall totals closer to the lake as the season progressed (Figures 6A,C,E,G). Comparatively, the percent contribution of lake-effect snow exhibited greater variability throughout a season than snowfall totals. During the early and peak seasons, lake-effect contributed the most to seasonal snowfall totals in north-central Central New York, including the Tug Hill (Figures 6B,D,F,H). By the mid-season, the greatest contributions were near the southeastern shore of the lake, centered over western Oswego County. Come the late-winter, lake-effect was relatively sparse throughout Central New York.
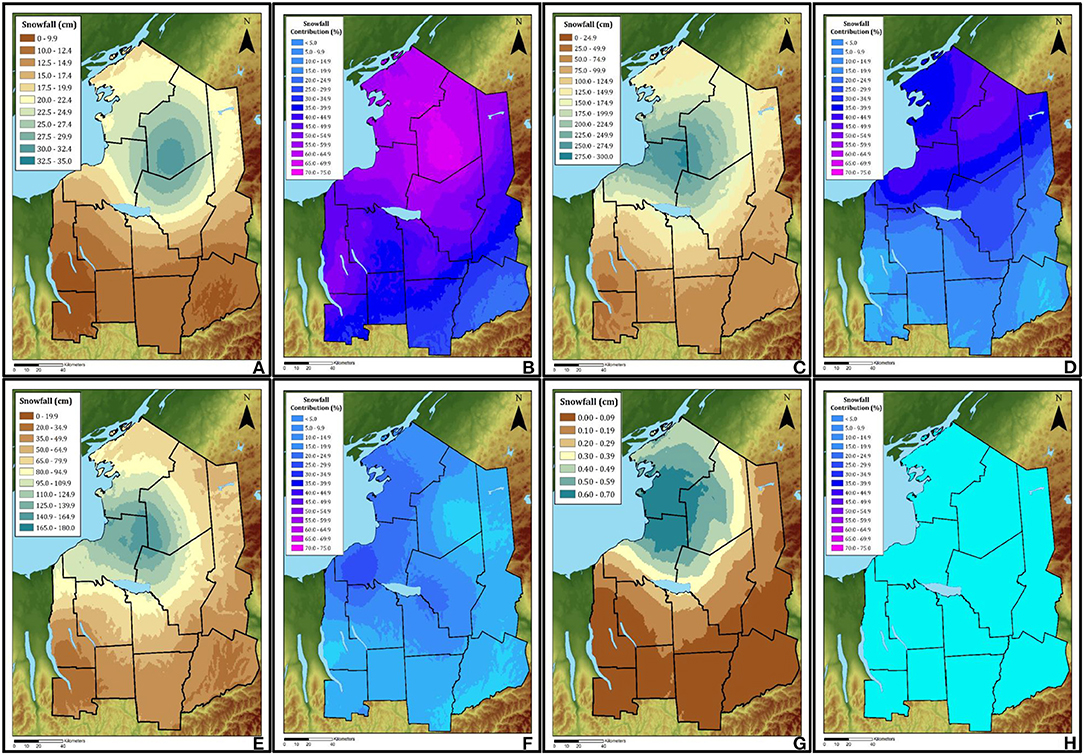
Figure 6. Sub-seasonal snowfall contributions of lake-effect snow within Central New York from 1995/96 to 2014/15. Snowfall contributions (totals and percentage) are mapped for October to November (A,B), December to January (C,D), February to March (E,F), and April to May (G,H).
Even though the general spatial patterns of lake-effect snowfall contributions remained relatively consistent across the four sub-seasons, the relative percentage of lake-effect snow compared to the other snowstorm types changed dramatically as winter progressed. In the early season (October–November), lake-effect snow dominates throughout Central New York, accounting for at least 40% of the snow throughout the majority (73.9%) of the study area (Figure 6B). The greatest contributions (> 65%) are centered over the Tug Hill, producing upwards of 30 cm per season (Figure 6A). Lake effect snow remains the dominant snowfall contributor in December and January for northern Central New York, but produces considerably more snowfall (225–300 cm) during this time (Figures 6C,D). The largest contributions from lake-effect snow shifted north, with parts of northern Lewis and northwest Jefferson Counties receiving 45–55% of their snowfall from lake-effect (Figure 6D). Lake effect is noticeably less prevalent in southern Central New York with contributions ranging from 9 to 15%. Later in the snowfall season, lake-effect contributions decrease further. Although these storms are still producing an average of 80–180 cm of snow over and around the Tug Hill from February–March, they only account for 25–35% of the snow, mostly near the lakeshore (Figures 6E,F). The maximum percentage that lake-effect contributed during this time (30–35%) was located on the southeastern shore of the lake over western Oswego County. By the late-snowfall season (April–May), lake-effect snow accounts for less than one centimeter of the snow, which equates to <5% of the snowfall that occurs throughout Central New York (Figures 6G,H).
Discussion
Snowfall Contributions From Lake-Effect Snow
A persistent question in the climatological community has been how much of the Great Lakes' snowfall derives from lake-effect snow. Previous estimates suggest that lake-effect snowstorms contribute between 30–70% of the seasonal snowfall downwind of the Great Lakes (Eichenlaub, 1970; Miner and Fritsch, 1997; Liu and Moore, 2004; Veals and Steenburgh, 2015). However, these estimates ignore the spatial variability that exists within a lake's snow basin, and often assumes a single contribution at a coarse resolution. Findings from this research suggest that the greatest contributions (45–55%) from lake snowstorms are located directly east of Lake Ontario, including over the Tug Hill (Figures 3, 4); findings that are consistent with those of Hartnett (2020) and Suriano and Wortman (2021). Although lake snowstorms account for over 50% of the seasonal snowfall in parts of Central New York, contributions are closer to 25% further from the lake. These findings align with those of Eichenlaub (1970), who found that lake-derived snowfall accounts for at least 20% of the seasonal snowfall in areas to the lee of the Great Lakes. However, this is notable variability within a relatively small area. It is well-documented that lake-effect snow can be highly localized, as it depends on factors such as wind speed and direction, fetch, and elevation (Peace and Sykes, 1966; Niziol, 1987; Reinking et al., 1993; Niziol et al., 1995; Lackmann, 2001). Slight changes in any of these factors can produce large magnitude differences in snowfall totals between locations only kilometers away. This research shows that seasonal snowfall contributions vary considerably across central New York State, and the importance of scale when working with lake-effect snow, something that has been largely ignored in previous studies.
Lake-effect storms contribute the most to seasonal snowfall totals to the lee of Lake Ontario, with enhanced contributions also over the Southern Hills and western Adirondack Mountains (Figure 5A). The higher elevations and orientations of these regions are prime for lake-effect snow, leading to greater contributions (Wilson, 1977; Hjelmfelt, 1992; Notaro et al., 2013b). Lake-effect snow is especially common from December to January with contributions between 100 and 300 cm of snow for over half of the study area (Figure 6C). Although still common in the early and mid-seasons, snowfall contributions are considerably lower than the peak season (Figures 6A,E). Relative to the total snowfall during each sub-season, the majority of the study area receives at least 25–35% of its snowfall from lake-effect snowstorms from October–January (Figures 6A,B). Lake-effect contributions decrease considerably from February–May across the study area. The decrease is likely due to changes in lake and atmospheric dynamics (e.g., colder surface lake temperature, greater ice cover extent, smaller surface to 850 hPa lapse rates and CAPE, lower boundary layer height, etc.) which inhibit the formation of lake-effect snow (Cordeira and Laird, 2008; Brown and Duguay, 2010; Notaro et al., 2013a; Vavrus et al., 2013). Additionally, by the late snowfall season, other snowstorms (e.g., Nor'easters and Rocky lows) become more frequent due to the southern displacement and meridional patterns of the polar jet stream (Hirsch et al., 2001; Hoskins and Hodges, 2019).
There is also a noticeable shift in the maximum contribution of lake-effect across a cold-season. Although lake-effect contributions are generally greatest directly east of Lake Ontario, there is a movement of the largest snowfall totals closer to the lake as the season progresses (Figures 6A,C,E,G). Additionally, the largest percent contributions of lake-effect snow are over the Tug Hill during the early season, shift north by the peak-season, and then southeast of and closer to the lake shore by the mid-season (Figures 6B,D,F,H). These intraseasonal shifts may be due to a weakening of the conditions necessary for the formation of lake-effect snow, most notably greater ice cover on Lake Ontario and a decreased surface to 850 hPa lapse rate (Wright et al., 2013). As Wright et al. (2013) suggest, frozen lakes and cooler lake surface temperatures will shrink the snow bands and confine them closer to the lake shore, which ultimately diminishes lake-effect contributions further from the lake. Furthermore, the location of snow bands is influenced by the presence of upper-tropospheric short-wave troughs (Metz et al., 2019). Metz et al. (2019) found that lake-effect bands concurrent with short-wave troughs were most common during the peak season (December–January), which may displace snow bands directly east or northeast of the long-axis of the lake.
Multiple researchers have noted a pronounced increase in snowfall in areas downwind of the Great Lakes compared to areas upwind of the lakes (Changnon, 1968; Gatz and Changnon, 1976; Kelly, 1986; Braham and Dungey, 1995; Scott and Huff, 1996, 1997); but few have examined the spatial variability that exists within a snow basin. From the results, lake-effect snow contributions are 150–250% greater in areas directly east of Lake Ontario compared to southeastern, southwestern, and northern Central New York (Figure 5A). Although lake-effect snow is mostly confined to the nearshore of the lakes, snow bands can propagate inland (Eipper et al., 2019). The presence of lake-effect in this region requires strong, organized snow bands capable of retaining moisture over high terrain. To produce such snow bands there generally needs to be a multi-lake upwind connection. These connections produce lake-to-lake snow bands, snow bands that continuously extend across two or more lakes, that tend to be less organized and less frequent (Schultz et al., 2004), resulting in the lower lake-effect contributions. Nonetheless, this leads to challenges in defining a lake's snow basin. If defined by the influence of lake-effect snow, it may be argued that all of Central New York falls within the Lake Ontario snow basin. However, as per the results, there are clear spatial variations that exist throughout this relatively small region. The results highlight the importance of non-lake snowstorms in a region that is seemingly dominated by lake-effect snow. The pronounced occurrence of non-lake snowstorms is likely due to the additional moisture supply from the Atlantic Ocean, a source less available to other parts of the Great Lakes region (Sanders and Gyakum, 1980; Zishka and Smith, 1980; Jacobs et al., 2005; Changnon et al., 2008).
Snowfall Contributions From Non-lake-effect Snow
Of the non-lake-effect snowstorms that produce snowfall in Central New York, Nor'easters and Rocky lows contribute the most to seasonal snowfall totals. Most of the study area (67.6%) receives 16–25% of its seasonal snowfall from Nor'easters (Figure 5B), while 86.1% receives 16–25% of its seasonal snowfall from Rocky lows (Figure 5C). Nor'easters are especially influential in southeastern Central New York, while Rocky lows dominate in southwestern Central New York. The greater contributions in these areas are likely due to the source regions of the storms and their general tracks. Nor'easters, also known as east-coast storms, form along the baroclinic zone of the eastern United States or Gulf Coast (Sanders and Gyakum, 1980; Zishka and Smith, 1980; Cione et al., 1993; Hirsch et al., 2001; Kocin and Uccellini, 2004; Jacobs et al., 2005). As these storms propagate to the northeast, the central low moves to the southeast of Central New York, producing snowfall over the region (northwest quadrant of the storm) (Bosart, 1973; Hirsch et al., 2001; Kocin and Uccellini, 2004; Mercer and Richman, 2007). Central New York's position to the northwest of the storm's center often brings a period of significant snowfall sourced in Atlantic moisture brought in by the northeasterly winds. In the northwest sector of the storm, northwest winds often cause the formation of lake-effect and lake-enhanced snowfall to the southeast of Lake Ontario (Niziol, 1987; Liu and Moore, 2004; Suriano and Leathers, 2017). Rocky lows typically originate to the lee of the Rocky Mountains (Whittaker and Horn, 1981; Zielinski, 2002; Changnon and Changnon, 2006; Hartnett, 2020). As they move eastward, they tend to display a pronounced curvature in their track west of the Appalachian range (Changnon, 1969; Branick, 1997; Zielinski, 2002; Changnon et al., 2008). This displaces the low pressure west of the central low of Nor'easters, likely accounting for the greater snowfall contributions in southwestern Central New York.
A noticeable difference between snowfall contributions from Nor'easters and Rocky lows was the pronounced spatial variability across the study area. Although lake-effect snowstorms have the highest contribution of any snowstorm types to a single area, snowfall contributions from Nor'easters were more homogenous. This likely reflects the general size of these storms. Since Nor'easters are some of the largest snowstorms (Davis and Dolan, 1993), they tend to affect the entire study area. Additionally, there is a pronounced decrease in snowfall contributions from Rocky lows directly east of Lake Ontario over Oswego County. Since Rocky lows tend to occur more frequently in late winter (Whittaker and Horn, 1981), lake surface temperatures are cold and ice cover extent is at a maximum (Wang et al., 2012). Therefore, when these storms pass across the region, there is limited access to moisture from the lake inhibiting the formation of lake-effect and lake-enhanced snow which may be common with earlier season storms. Thus, areas that typically receive lake-effect snow do not receive any additional snow from the Rocky lows.
Snowfall contributions from Canadian lows and non-cyclonic storms are relatively low and homogenous throughout the study area (Figures 5D,E). The northern formation of Canadian lows is likely responsible for their low seasonal contribution (Figure 5D). Since these storms form at northern latitudes inland from major moisture sources, they are often associated with anomalously cold, yet dry conditions (Hutchinson, 1995; Thomas and Martin, 2007). Snowfall directly generated from the central is often light to moderate in magnitude with a high snow-to-liquid ratio (Rochette et al., 2017). Non-cyclonic storms are associated with an upper-level disturbance, cold air advection, or quasi-stationary fronts, but no central low pressure near the study area. Although they can be accompanied by significant lake-effect snow development, they tend to be varied in form and genesis (Lackmann, 2001; Scott and Sousounis, 2001; Chuang and Sousounis, 2003). Upper atmospheric disturbances tend to favor ascending air and an unstable atmosphere ahead of a westerly trough (NWS, 2014). Since the trough can extend to the Gulf of Mexico, the snowfall produced often occurs throughout Central New York, as shown by the relatively homogeneous percent contributions (Figure 5E). Behind the western trough, winds generally shift from the northwest, which are conducive for the formation of lake-effect snow, and the greater snowfall totals in northern Central New York. Stationary fronts can also produce a relatively homogenous percent snowfall contribution across Central New York when the warmer air mass contains a lot of water vapor (Neiman et al., 1998; Kusunoki et al., 2005). Since the front is stationary, this can lead to prolonged periods of intense precipitation. A storm's influence on snowfall is not just driven by the regional geography, but by the nature of the storm.
Conclusions
Lake-effect snow is a regional phenomenon that greatly influences the climate, hydrology, biology, and economy of the Laurentian Great Lakes. Although it is considered the dominant snowstorm type in this region, it is not the only storm that produces snow. This research examines the seasonal snowfall contributions from the different snowstorm types in Central New York. The results suggest that although lake-effect storms are the dominant snowfall contributor (48%) in parts of the study area, notably the Tug Hill, seasonal contributions are as little as 13% in southeastern Central New York. Additionally, as the cold season progresses, there is a general decrease in the percent contribution from lake-effect throughout the study area and a movement of the maximum contributions. During the early and peak sub-season, the largest contributions are found tens of kilometers inland, directly east of Lake Ontario. By the mid to late cold season, greater snowfall totals from lake-effect snow are concentrated closer to the lakeshore with the largest percent contributions found southeast of the lake's longitudinal axis. Snowfall totals in southern Central New York are mostly dominated by Nor'easters (25–35%) and Rocky lows (15–20%). This is likely driven by moisture sourced from the Atlantic Ocean rather than the Great Lakes. To reduce the over homogenization of snowfall contributions, it is suggested that snowfall patterns within the Great Lakes basin are examined at the local level.
Understanding the actual snowfall contribution from different snowstorm types throughout a snowfall season is needed for future climate predictions. Since the early twentieth century, trends in lake-effect snowfall have fluctuated, while snowfall trends for areas less prone to lake-effect snow have remained unchanged or decreased (Norton and Bolsenga, 1993; Ellis and Leathers, 1996; Burnett et al., 2003; Kunkel et al., 2009a; Bard and Kristovich, 2012; Hartnett et al., 2014). Seasonal snowfall patterns within the Great Lakes region are closely tied to air temperatures, lake surface temperatures, and ice cover on the lakes (Tsuboki et al., 1989; Hanson et al., 1992; Segal and Kubesh, 1996; Wang et al., 2012; Notaro et al., 2015; Shi and Xue, 2019). Since the formation of lake-effect snowstorms and non-lake-effect snowstorms are fundamentally different, a warming climate may have contrasting influences on these storms. This is especially important for storms that occur near the beginning or end of the snowfall season, as recent studies have noted a transition from snow to rain in these storms (Schmidlin et al., 1987; Groisman and Easterling, 1994; Miner and Fritsch, 1997; Knowles et al., 2006; Pierce and Cayan, 2013; Kluver and Leathers, 2015; Clark et al., 2020). Since the potential trajectory of future snowfall varies according to the type of storm, for accurate snowfall predictions, models need to decipher the relative contributions of different snowstorm types to the seasonal snowfall total. Therefore, the analyses in this study help to better understand how snowfall may change in the future by directly teasing out the snowfall contributions from different snowstorm types. These analyses also emphasize the spatial variability of snowfall contributions, which suggest that future snowfall trends may vary across a region depending on the type of snowstorm that dominates seasonal totals. The results from this study provide an important baseline to track these future scenarios, and to help isolate the changes in frequency and contributions of all the storms that track across the region.
Data Availability Statement
The original contributions presented in the study are included in the article/Supplementary Materials, further inquiries can be directed to the corresponding author/s.
Author Contributions
This research was developed and completed by JH. All methodologies, analyses, and writing were conducted by JH.
Conflict of Interest
The author declares that the research was conducted in the absence of any commercial or financial relationships that could be construed as a potential conflict of interest.
Publisher's Note
All claims expressed in this article are solely those of the authors and do not necessarily represent those of their affiliated organizations, or those of the publisher, the editors and the reviewers. Any product that may be evaluated in this article, or claim that may be made by its manufacturer, is not guaranteed or endorsed by the publisher.
Acknowledgments
This research arose from authors' dissertation at Syracuse University. The author would like to acknowledge the input from Dr. Susan Millar, professor emeritus of Syracuse University, Dr. Jacob Bendix of Syracuse University, Dr. Peng Gao of Syracuse University, and Dr. Adam Burnett of Colgate University.
Supplementary Material
The Supplementary Material for this article can be found online at: https://www.frontiersin.org/articles/10.3389/frwa.2021.780869/full#supplementary-material
References
Andersson, T., and Nilsson, S. (1990). Topographically induced convective snowbands over the Baltic Sea and their precipitation distribution. Weather Forecast. 5, 299–312.
Bard, L., and Kristovich, D. A. R. (2012). Trend reversal in Lake Michigan contribution to snowfall. J. Appl. Meteorol. Climatol. 51, 2038–2046. doi: 10.1175/JAMC-D-12-064.1
Bosart, L. F.. (1973). Detailed analyses of precipitation patterns associated with mesoscale features accompanying United States East Coast Cyclogenesis. Mon. Weather Rev. 101, 1–12.
Braham, R. R., and Dungey, M. J. (1995). Lake-effect snowfall over Lake Michigan. J. Appl. Meteorol. 34, 1009–1019. doi: 10.1175/1520-0450(1995)034<1009:LESOLM>2.0.CO;2
Branick, M. L.. (1997). A climatology of significant winter-type weather events in the contiguous United States, 1982–94. Weather Forecast. 12, 193–207. doi: 10.1175/1520-0434(1998)013<0884:COACOS>2.0.CO;2
Brown, L. C., and Duguay, C. R. (2010). The response and role of ice cover in lake-climate interactions. Prog. Phys. Geogr. 34, 671–704. doi: 10.1177/0309133310375653
Burnett, A. W., Kirby, M. E., Mullins, H. T., and Patterson, W. P. (2003). Increasing Great Lake-effect snowfall during the twentieth century: a regional response to global warming? J. Clim. 16, 3535–3542. doi: 10.1175/1520-0442(2003)016<3535:IGLSDT>2.0.CO;2
Campbell, L. S., and James Steenburgh, W. (2017). The OWLeS IOP2b lake-effect snowstorm: Mechanisms contributing to the Tug Hill precipitation maximum. Mon. Weather Rev. 145, 2461–2478. doi: 10.1175/MWR-D-16-0461.1
Campbell, L. S. W. J, Steenburgh, P. G., Veals, T. W., Letcher, J. R., and Minder (2016). Lake-effect mode and precipitation enhancement over the tug hill plateau during OWLeS IOP2b. Mon. Weather Rev., 144, 1729–1748. doi: 10.1175/MWR-D-15-0412.1
Changnon, D., Merinsky, C., and Lawson, M. (2008). Climatology of surface cyclone tracks associated with large Central and Eastern U.S. Snowstorms, 1950–2000. Mon. Weather Rev., 136, 3193–3202. doi: 10.1175/2008MWR2324.1
Changnon, S. A.. (1968). Precipitation climatology of Lake Michigan Basin. Illinois State Water Surv. Bull. 52:31.
Changnon, S. A.. (1969). Climatology of Severe Winter Storms in Illinois. Champaign, IL, 1–42. Available online at: http://www.isws.uiuc.edu/pubdoc/B/ISWSB-53.pdf (accessed August, 2021).
Changnon, S. A., and Changnon, D. (2006). A spatial and temporal analysis of damaging snowstorms in the United States. Nat. Hazards 37, 373–389. doi: 10.1007/s11069-005-6581-4
Chuang, H.-Y., and Sousounis, P. J. (2003). The impact of the prevailing synoptic situation on the lake-aggregate effect. Mon. Weather Rev. 131, 990–1010. doi: 10.1175/1520-0493(2003)131<0990:tiotps>2.0.co;2
Cione, J. J., Raman, S., and Pietrafesa, L. J. (1993). The effect of gulf stream-induced baroclinicity on U.S. East Coast Winter Cyclones. Mon. Weather Rev. 121, 421–430. doi: 10.1175/1520-0493(1993)121<0421:TEOGSI>2.0.CO;2
Clark, C. A., Elless, T. J., Lyza, A. W., Ganesh-Babu, B., Koning, D. M., Carne, A. R., et al. (2016). Spatiotemporal snowfall variability in the Lake Michigan Region: How is warming affecting wintertime snowfall? J. Appl. Meteorol. Climatol. 55, 1813–1830. doi: 10.1175/JAMC-D-15-0285.1
Clark, C. A., Goebbert, K. H., Ganesh-Babu, B., Young, A. M., Heinlein, K. N., Casas, E. G., et al. (2020). Classification of Lake Michigan snow days for estimation of the lake-effect contribution to the downward trend in November snowfall. Int. J. Climatol. 40, 5656–5670. doi: 10.1002./joc.6542
Cordeira, J. M., and Laird, N. F. (2008). The influence of ice cover on two lake-effect snow events over Lake Erie. Mon. Weather Rev. 136, 2747–2763. doi: 10.1175/2007MWR2310.1
Cortinas, M. R., and Kitron, U. (2006). County-level surveillance of white-tailed deer infestation by Ixodes scapularis and Dermacentor albipictus (Acari: Ixodidae) along the Illinois River. J. Med. Entomol. 43, 810–819. doi: 10.1603/0022-2585(2006)43
Daly, C., Gibson, W. P., Taylor, G. H., Doggett, M. K., and Smith, J. I. (2007). Observer bias in daily precipitation measurements at United States Cooperative Network Stations. Bull. Am. Meteorol. Soc. 88, 899–912. doi: 10.1175/BAMS-88-6-899
Dewey, K. F.. (1970). A Quantitative Analysis of Lake-Effect Snow. Illinois: Northern Illinois State University.
Doesken, N. J., and Judson, A. (1996). The Snow Booklet: A Guide to the Science, Climatology and Measurement of Snow in the United States. Colorado: Colorado State University.
Eichenlaub, V. L.. (1970). Lake effect snowfall to the lee of the Great Lakes: Its role in Michigan. Bull. Am. Meteorol. Soc. 51, 403–412. doi: 10.1175/1520-0477(1970)051<0403:LESTTL>2.0.CO;2
Eichenlaub, V. L., and Hodler, T. W. (1979). Weather and Climate of the Great Lakes Region. South Bend, IN: University of Notre Dame Press
Eipper, D. T., Greybush, S. J., Young, G. S., Saslo, S., Sikora, T. D., and Clark, R. D. (2019). Lake-effect snowbands in baroclinic environments. Weather Forecast., 34, 1657–1674. doi: 10.1175/WAF-D-18-0191.1
Eito, H., Kato, T., Yoshizaki, M., and Adachi, A. (2005). Numerical simulation of the quasi-stationary snowband observed over the southern coastal area of the Sea of Japan on 16 January 2001. J. Meteorol. Soc. Japan 83, 551–576. doi: 10.2151/jmsj.83.551
Ellis, A. W., and Leathers, D. J. (1996). A synoptic climatological approach to the analysis of lake-effect snowfall: potential forecasting applications. Weather Forecast. 11, 216–229. doi: 10.1175/1520-0434(1996)011<0216:ASCATT>2.0.CO;2
Fairman, J. G., Schultz, D. M., Kirshbaum, D. J., Gray, S. L., and Barrett, A. I. (2016). Climatology of banded precipitation over the contiguous United States. Mon. Weather Rev. 144, 4553–4568. doi: 10.1175/MWR-D-16-0015.1
Gatz, D. F., and Changnon, S. A. (1976). Atmospheric Environment of the Lake Michigan Drainage Basin. Argonne, Illinois: ANLlES.
Grieser, J.. (2015). Interpolation of global monthly rain gauge observations for climate change analysis. J. Appl. Meteorol. Climatol. 54, 1449–1464. doi: 10.1175/JAMC-D-14-0305.1
Groisman, P. Y., and Easterling, D. R. (1994). Variability and trends of total precipitation and snowfall over the United States and Canada. J. Clim. 7, 184–205. doi: 10.1175/1520-0442(1994)007<0184:VATOTP>2.0.CO;2
Guan, H., Wilson, J. L., and Makhnin, O. (2005). Geostatistical mapping of mountain precipitation incorporating autosearched effects of terrain and climatic characteristics. J. Hydrometeorol. 6, 1018–1031. doi: 10.1175/JHM448.1
Hagenstad, M., Burakowski, E., and Hill, R. (2018). “The economic contributions of winter sports in a changing climate,” in Prot. Our Winters, 1–69.
Hall, D. K., Digirolamo, N. E., and Frei, A. (2017). “Contribution of lake-effect snow to the catskill mountains snowpack,” in 74th East. SNOW Conference.
Hanson, H. P., Hanson, C. S., and Yoo, B. H. (1992). Recent great lakes ice trends. Bull. Am. Meteorol. Soc., 73, 577–584. doi: 10.1175/1520-0477(1992)073<0577:RGLIT>2.0.CO;2
Harrington, J. A. Jr,, Cerveny, R. S., and Dewey, K. F. (1987). A climatology of mean monthly snowfall for the conterminous United States: temporal and spatial patterns. J. Clim. Appl. Meteorol. 26, 897–912.
Hartnett, J. J.. (2020). A classification scheme for identifying snowstorms affecting central New York State. Int. J. Climatol. 41, 1712–1730. doi: 10.1002/joc.6922
Hartnett, J. J., Collins, J. M., Baxter, M. A., and Chambers, D. P. (2014). Spatiotemporal snowfall trends in Central New York. J. Appl. Meteorol. Climatol. 53, 2685–2697. doi: 10.1175/JAMC-D-14-0084.1
Hill, J. D.. (1971). Snow Squalls in the Lee of Lake Erie and Lake Ontario: A Review of the Literature. Volume 43. Bohemia: NOAA - National Weather Service Eastern Region.
Hirsch, M. E., DeGaetano, A. T., and Colucci, S. J. (2001). An East Coast winter storm climatology. J. Clim. 14, 882–899. doi: 10.1175/1520-0442(2001)014<0882:AECWSC>2.0.CO;2
Hjelmfelt, M. R.. (1992). Orographic effects in simulated lake-effect snowstorms over Lake Michigan. Mon. Weather Rev. 120, 373–377. doi: 10.1175/1520-0493(1992)120<0373:OEISLE>2.0.CO;2
Hoskins, B. J., and Hodges, K. I. (2019). The annual cycle of Northern Hemisphere storm tracks. Part II: Regional detail. J. Clim. 32, 1761–1775. doi: 10.1175/JCLI-D-17-0871.1
Houze, R. A., and Hobbs, P. V. (1982). “Organization and structure of precipitating cloud systems,” in B.B.T.-A., ed G. Saltzman (New York: Elsevier), 225–315. Available online at: https://www.sciencedirect.com/science/article/pii/S006526870860521X
Hutchinson, T. A.. (1995). An analysis of NCM's nested grid models of alberta clippers. Weather Forecast. 10, 632–641.
Jacobs, N. A., Lackmann, G. M., and Raman, S. (2005). The combined effects of Gulf Stream-induced baroclinicity and upper-level vorticity on US East Coast extratropical cyclogenesis. Mon. Weather Rev. 133, 2494–2501. doi: 10.1175/MWR2969.1
Karmosky, C.. (2007). Synoptic Climatology of Snowfall in the Northeastern United States: An Analysis of Snowfall Amounts From Diverse Synoptic Weather Types. Delaware: University of Delaware.
Kelly, R. D.. (1986). Mesoscale frequencies and seasonal snowfalls for different types of lake michigan snow storms. J. Clim. Appl. Meteorol. 25, 308–312.
Kindap, T.. (2010). A severe sea-effect snow episode over the city of Istanbul. Nat. Hazards 54, 707–723. doi: 10.1007/s11069-009-9496-7
Kluver, D., and Leathers, D. (2015). Regionalization of snowfall frequency and trends over the contiguous United States. Int. J. Climatol. 35, 4348–4358. doi: 10.1002/joc.4292
Knowles, N., Dettinger, M. D., and Cayan, D. R. (2006). Trends in snowfall versus rainfall in the western United States. J. Clim. 19, 4545–4559. doi: 10.1175/JCLI3850.1
Kocin, P. J., and Uccellini, L. W. (2004). Northeast Snowstorms. Vol. 54. Massachusetts: American Meteorological Society.
Kristovich, D. A. R., Young, G. S., Verlinde, J., Sousounis, P. J., Mourad, P., Lenschow, D., et al. (2000). The lake-induced convection experiment and the snowband dynamics project. Bull. Am. Meteorol. Soc. 81, 519–542. doi: 10.1175/1520-0477(2000)081<0519:TLCEAT>2.3.CO;2
Kulie, M. S., Pettersen, C., Merrelli, A. J., Wagner, T. J., Wood, N. B., Dutter, M., et al. (2021). Snowfall in the Northern Great Lakes: lessons learned from a multisensor observatory. Bull. Am. Meteorol. Soc. 102, E1317–E1339. doi: 10.1175/BAMS-D-19-0128.1
Kunkel, K. E., Ensor, L., Palecki, M., Easterling, D., Robinson, D., Hubbard, K. G., et al. (2009a). A new look at lake-effect snowfall trends in the Laurentian Great Lakes using a temporally homogeneous data set. J. Great Lakes Res. 35, 23–29. doi: 10.1016/j.jglr.11.2008.003
Kunkel, K. E., Palecki, M., Ensor, L., Hubbard, K. G., Robinson, D., Redmond, K., et al. (2009b). Trends in twentieth-century U.S. snowfall using a quality-controlled dataset. J. Atmos. Ocean. Technol. 26, 33–44. doi: 10.1175/2008JTECHA1138.1
Kunkel, K. E., Palecki, M. A., Hubbard, K. G., Robinson, D. A., Redmond, K. T., and Easterling, D. R. (2007). Trend identification in twentieth-century U.S. snowfall: the challenges. J. Atmos. Ocean. Technol. 24, 64–73. doi: 10.1175/JTECH2017.1
Kusunoki, K., Murakami, M, Orikasa, N., Hoshimoto, M., Tanaka, Y., Yamada, Y., Mizuno, H., et al. (2005). Observations of quasi-stationary and shallow orographic snow clouds: spatial distributions of supercooled liquid water and snow particles. Mon. Weather Rev. 133, 743–751. doi: 10.1175/mwr2874.1
Lackmann, G. M.. (2001). Analysis of a surprise western new york snowstorm. Weather Forecast. 16, 99–116. doi: 10.1175/1520-0434(2001)016<0099:AOASWN>2.0.CO;2
Laird, N., Sobash, R., and Hodas, N. (2009). The frequency and characteristics of lake-effect precipitation events associated with the New York State Finger lakes. J. Appl. Meteorol. Climatol. 48, 873–886. doi: 10.1175/2008JAMC2054.1
Leathers, D. J., and Ellis, A. W. (1996). Synoptic mechanisms associated with snowfall increases to the lee of Lakes Erie and Ontario. Int. J. Climatol. 16, 1117–1135. doi: 10.1002/(SICI)1097-0088(199610)16:10<1117::AID-JOC80>3.0.CO;2-4
Leeper, R. D., Rennie, J., and Palecki, M. A. (2015). Observational perspectives from U.S. Climate Reference Network (USCRN) and Cooperative Observer Program (COOP) Network: temperature and precipitation comparison. J. Atmos. Ocean. Technol. 32, 703–721. doi: 10.1175/JTECH-D-14-00172.1
Liu, A. Q., and Moore, G. W. K. (2004). Lake-effect snowstorms over Southern Ontario, Canada, and their associated synoptic-scale environment. Mon. Weather Rev. 132, 2595–2609. doi: 10.1175/MWR2796.1
Lundquist, J. D., Hughes, M., Henn, B., Gutmann, E. D., Livneh, B., Dozier, J., et al. (2015). High-elevation precipitation patterns: using snow measurements to assess daily gridded datasets across the Sierra Nevada, California*. J. Hydrometeorol. 16, 1773–1792. doi: 10.1175/jhm-d-15-0019.1
Mercer, A. E., and Richman, M. B. (2007). Statistical differences of quasigeostrophic variables, stability, and moisture profiles in north american storm tracks. Mon. Weather Rev. 135, 2312–2338. doi: 10.1175/MWR3395.1
Metz, N. D., Bruick, Z. S., Capute, P. K., Neureuter, M. M., Ott, E. W., and Sessa, M. F. (2019). An investigation of cold-season short-wave troughs in the Great Lakes Region and their concurrence with lake-effect clouds. J. Appl. Meteorol. Climatol. 58, 605–614. doi: 10.1175/JAMC-D-18-0177.1
Miner, T. J., and Fritsch, J. M. (1997). Lake-effect rain events. Mon. Weather Rev. 125, 3231–3248. doi: 10.1175/1520-0493(1997)125<3231:LERE>2.0.CO;2
Neiman, P. J., Ralph, F. M., Shapiro, M. A., Smull, B. F., and & Johnson, D. (1998). An observational study of fronts and frontal mergers over the continental United States. Mon. Weather Rev. 126, 2521–2554. doi: 10.1155/2014/816729
Niziol, T. A.. (1987). Operational forecasting of lake effect snowfall in western and central New York. Weather Forecast. 2, 310–321. doi: 10.1175/1520-0434(1987)002<0310:OFOLES>2.0.CO;2
Niziol, T. A., Snyder, W. R., and Waldstreicher, J. S. (1995). Winter Weather Forecasting throughout the Eastern United States. Part IV: Lake Effect Snow. Weather Forecast. 10, 61–77. doi: 10.1175/1520-0434(1995)010<0061:WWFTTE>2.0.CO;2
Norton, D. C., and Bolsenga, S. J. (1993). Spatiotemporal trends in lake effect and continental snowfall in the Laurentian Great Lakes, 1951–1980. J. Clim. 6, 1943–1956. doi: 10.1175/1520-0442(1993)006<1943:STILEA>2.0.CO;2
Notaro, M., Bennington, V., Vavrus, S., and Zarrin, A. (2015). Dynamically downscaled projections of lake-effect snow in the Great Lakes Basin. J. Clim. 28, 1661–1684. doi: 10.1175/JCLI-D-14-00467.1
Notaro, M., Holman, K., Zarrin, A., Fluck, E., Vavrus, S., and Bennington, V. (2013a). Influence of the Laurentian Great Lakes on regional climate. J. Clim. 26, 789–804. doi: 10.1175/JCLI-D-12-00140.1
Notaro, M., Zarrin, A., Vavrus, S., and Bennington, V. (2013b). Simulation of heavy lake-effect snowstorms across the great lakes basin by RegCM4: synoptic climatology and variability. Mon. Weather Rev. 141, 1990–2014. doi: 10.1175/MWR-D-11-00369.1
NWS (2012). Snow Measurement Guidelines for National Weather Service Snow Spotters. Peachtree City, Georgia: NWS, 1–5.
NWS (2014). Winter Weather Basics: Cold Air Outbreaks. Gaylord, MI: Winter Talks Series, National Weather Service. Available online at: https://www.weather.gov/media/apx/spotter/Basics_Winter_Wx.pdf (accessed September, 2021).
Owens, N. D., Rauber, R. M., Jewett, B. F., and McFarquhar, G. M. (2017). The contribution of lake enhancement to extreme snowfall within the Chicago-Milwaukee urban corridor during the 2011 Groundhog Day Blizzard. Mon. Weather Rev. 145, 2405–2420. doi: 10.1175/MWR-D-17-0025.1
Peace, R. L., and Sykes, R. B. (1966). Mesoscale study of a lake effect snow storm. Mon. Weather Rev. 94, 495–507. doi: 10.1175/1520-0493(1966)094<0495:MSOALE>2.3.CO;2
Pease, S. R., Lyons, W. A., Keen, C. S., and Hjelmfelt, M. (1988). Mesoscale spiral vortex embedded within a Lake Michigan snow squall band—high resolution satellite observations and numerical model simulations. Mon. Weather Rev. 116, 1374–1380. doi: 10.1175/1520-0493(1988)116<1374:MSVEWA>2.0.CO;2
Perry, L. B., and Konrad, C. E. (2006). Relationships between NW flow snowfall and topography in the Southern Appalachians, USA. Clim. Res. 32, 35–47. doi: 10.3354/cr032035
Perry, L. B., Konrad, C. E., and Schmidlin, T. W. (2007). Antecedent upstream air trajectories associated with northwest flow snowfall in the Southern Appalachians. Weather Forecast. 22, 334–352. doi: 10.1175/WAF978.1
Pettersen, C., Kulie, M. S., Bliven, L. F., Merrelli, A. J., Petersen, W. A., Wagner, T. J., et al. (2020). A composite analysis of snowfall modes from four winter seasons in Marquette, Michigan. J. Appl. Meteorol. Climatol. 59, 103–124. doi: 10.1175/JAMC-D-19-0099.1
Pierce, D. W., and Cayan, D. R. (2013). The uneven response of different snow measures to human-induced climate warming. J. Clim. 26, 4148–4167. doi: 10.1175/JCLI-D-12-00534.1
Reinking, R. F., Caiazza, R., Kropfli, R. A., Orr, B. W., Martner, B. E., Niziol, T. A., et al. (1993). The Lake-Ontario winter storms (LOWS) project. Bull. Am. Meteorol. Soc. 74, 1828–1849. doi: 10.1175/1520-0477-74-10-1828
Rochette, S. M., Market, P. S., Gravelle, C. M., and Niziol, T. A. (2017). A case study of anomalous snowfall with an Alberta Clipper. Adv. Meteorol. 1–14. doi: 10.1155/2017/8406379
Rohr, J. R., Dobson, A. P., Johnson, P. T. J., Kilpatrick, A. M., Paull, S. H., Raffel, T. R., et al. (2011). Frontiers in climate change-disease research. Trends Ecol. Evol. 26, 270–277. doi: 10.1016/j.tree.03.2011.002
Rooney, J. F.. (1967). The urban snow hazard in the United States: an appraisal of disruption. Geogr. Rev. 57, 538–559.
Sanders, F., and Gyakum, J. R. (1980). Synoptic-dynamic climatology of the “bomb.” Mon. Weather Rev. 108, 1589–1606. doi: 10.1175/1520-0493(1980)108<1589:SDCOT>2.0.CO;2
Schmidlin, T. W., Dethier, B. E., and Eggleston, K. L. (1987). Freeze-thaw days in the northeastern United States. J. Clim. Appl. Meteorol. 26, 142–155.
Schultz, D. M., Arndt, D. S., Stensrud, D. J., and Hanna, J. W. (2004). Snowbands during the cold-air outbreak of 23 January 2003. Mon. Weather 132, 827–842. doi: 10.1175/1520-0493(2004)132<0827:SDTCOO>2.0.CO;2
Scott, C. P. J., and Sousounis, P. J. (2001). The utility of additional soundings for forecasting lake-effect snow in the Great Lakes Region. Weather Forecast. 16, 448–462. doi: 10.1175/1520-0434(2001)016<0448:TUOASF>2.0.CO;2
Scott, R. W., and Huff, F. A. (1996). Impacts of the Great Lakes on regional climate conditions. J. Great Lakes Res. 22, 845–863. doi: 10.1016/S0380-1330(96)71006-7
Scott, R. W., and Huff, F. A. (1997). Lake Effects on Climatic Conditions in the Great Lakes Basin. Report CR 617.
Segal, M., and Kubesh, R. (1996). Inferring snow-breeze characteristics from frozen-lake breezes. J. Appl. Meteorol. 35, 1033–1039. doi: 10.1175/1520-0450(1996)035<1033:ISBCFF>2.0.CO;2
Sharratt, B. S., Baker, D. G., Wall, D. B., Skaggs, R. H., and Ruschy, D. L. (1992). Snow depth required for near steady-state soil temperatures. Agric. For. Meteorol. 57, 243–251. doi: 10.1016/0168-1923(92)90121-J
Shi, Q., and Xue, P. (2019). Impact of lake surface temperature variations on lake effect snow over the Great Lakes region. J. Geophys. Res. Atmos. 124, 12553–12567. doi: 10.1029/2019JD031261
Steenburgh, W. J., Halvorson, S. F., and Onton, D. J. (2000). Climatology of lake-effect snowstorms of the Great Salt Lake. Mon. Weather Rev. 128, 709–727. doi: 10.1175/1520-0493(2000)128<0709:COLESO>2.0.CO;2
Strommen, N. D., and Harman, J. R. (1978). Seasonally changing patterns of lake-effect snowfall in western Lower Michigan. Mon. Weather Rev. 106, 503–509.
Suriano, Z. J., and Leathers, D. J. (2016). Twenty-first century snowfall projections within the eastern Great Lakes region: detecting the presence of a lake-induced snowfall signal in GCMs. Int. J. Climatol. 36, 2200–2209. doi: 10.1002/joc.4488
Suriano, Z. J., and Leathers, D. J. (2017). Synoptically classified lake-effect snowfall trends to the lee of Lakes Erie and Ontario. Clim. Res. 74, 1–13. doi: 10.3354/cr01480
Suriano, Z. J., Leathers, D. J., Hall, D. K., and Frei, A. (2019). Contribution of snowfall from diverse synoptic conditions in the Catskill/Delaware Watershed of New York State. Int. J. Climatol. 39, 3608–3618. doi: 10.1002/joc.6043
Suriano, Z. J., and Wortman, R. D. (2021). Temporal trends in snowfall contribution induced by lake-effect synoptic types. Phys. Geogr. 42, 416–433. doi: 10.1080/02723646.2020.1792048
Tardy, A.. (2000). Lake-effect and lake-enhanced snow in the Champlain Valley of Vermont. East. Reg. Tech. Attach. 1–27. Available online at: http://www.erh.noaa.gov/er/btv/research/Tardy-ta2000-05.pdf (accessed August, 2021).
Thomas, B. C., and Martin, J. E. (2007). A synoptic climatology and composite analysis of the Alberta Clipper. Weather Forecast. 22, 315–333. doi: 10.1175/WAF982.1
Tsuboki, K., Fujiyoshi, Y., Wakahama, G., Tsuboki, K., and Wakahama, G. (1989). Structure of a Land Breeze and Snowfall Enhancement at the Leading Edge. J. Meteorol. Soc. Japan, 67, 757–770.
Vavrus, S., Notaro, M., and Zarrin, A. (2013). The role of ice cover in heavy lake-effect snowstorms over the great lakes basin as simulated by RegCM4. Mon. Weather Rev. 141, 148–165. doi: 10.1175/MWR-D-12-00107.1
Veals, P. G., and Steenburgh, W. J. (2015). Climatological characteristics and orographic enhancement of lake-effect precipitation East of Lake Ontario and over the Tug Hill Plateau. Mon. Weather Rev. 143, 3591–3609. doi: 10.1175/MWR-D-15-0009.1
Wang, J., Bai, X., Hu, H., Clites, A., Colton, M., and Lofgren, B. (2012). Temporal and spatial variability of Great Lakes ice cover, 1973–2010. J. Clim. 25, 1318–1329. doi: 10.1175/2011JCLI4066.1
Whittaker, L. M., and Horn, L. H. (1981). Geographical and seasonal distribution of North American Cyclogenesis, 1958–1977. Mon. Weather Rev. 109, 2312–2322. doi: 10.1175/1520-0493(1981)109<2312:GASDON>2.0.C;2
Wilson, J. W.. (1977). Effect of Lake-Ontario on precipitation. Mon. Weather Rev. 105, 207–214. doi: 10.1175/1520-0493(1977)105<0207:EOLOOP>2.0.CO;2
Wright, D. M., Posselt, D. J., and Steiner, A. L. (2013). Sensitivity of lake-effect snowfall to lake ice cover and temperature in the great lakes region. Mon. Weather Rev. 141, 670–689. doi: 10.1175/MWR-D-12-00038.1
Wu, H., Hubbard, K. G., and You, J. (2005). Some concerns when using data from the cooperative weather station networks: a Nebraska case study. J. Atmos. Ocean. Technol. 22, 592–602. doi: 10.1175/JTECH1733.1
Zielinski, G. A.. (2002). A classification scheme for winter storms in the Eastern and Central United States with an emphasis on Nor'easters. Bull. Am. Meteorol. Soc. 83, 37–51. doi: 10.1175/1520-0477(2002)083<0037:ACSFWS>2.3.CO;2
Keywords: snowstorms, snowfall, lake-effect, Nor'easters, cokriging
Citation: Hartnett JJ (2021) The Seasonal Snowfall Contributions of Different Snowstorm Types in Central New York State. Front. Water 3:780869. doi: 10.3389/frwa.2021.780869
Received: 21 September 2021; Accepted: 15 November 2021;
Published: 07 December 2021.
Edited by:
Galina Guentchev, Met Office, United KingdomReviewed by:
Allan Frei, Hunter College (CUNY), United StatesZachary Suriano, University of Nebraska Omaha, United States
Copyright © 2021 Hartnett. This is an open-access article distributed under the terms of the Creative Commons Attribution License (CC BY). The use, distribution or reproduction in other forums is permitted, provided the original author(s) and the copyright owner(s) are credited and that the original publication in this journal is cited, in accordance with accepted academic practice. No use, distribution or reproduction is permitted which does not comply with these terms.
*Correspondence: Justin J. Hartnett, anVzdGluLmhhcnRuZXR0QG9uZW9udGEuZWR1