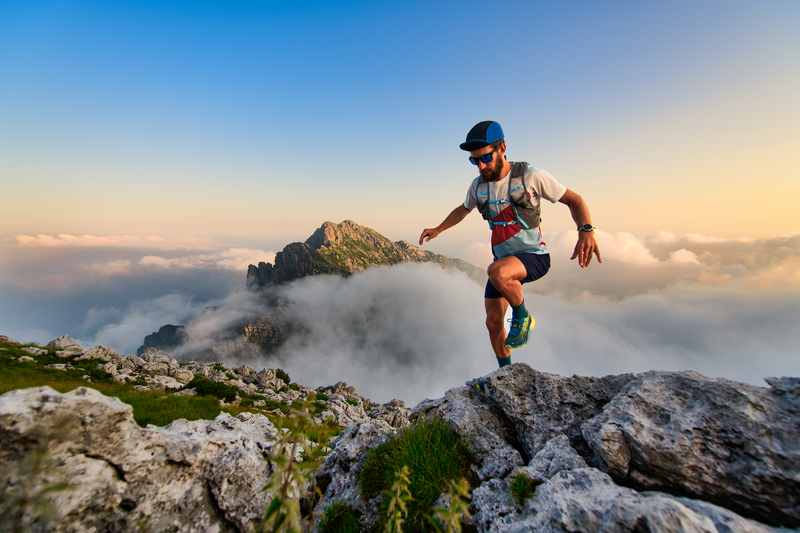
95% of researchers rate our articles as excellent or good
Learn more about the work of our research integrity team to safeguard the quality of each article we publish.
Find out more
ORIGINAL RESEARCH article
Front. Water , 20 October 2021
Sec. Water and Critical Zone
Volume 3 - 2021 | https://doi.org/10.3389/frwa.2021.736824
This article is part of the Research Topic Stable Isotope Ecohydrological Research of the Critical Zone View all 6 articles
As a result of canopy interception and transpiration, trees are often assumed to have negative effects on the local hydrological budget resulting in reduced soil and groundwater resources. However, it has also been shown that trees can have positive effects through reducing surface run-off and improving soil infiltrability and groundwater recharge, especially in many tropical ecosystems characterized by high rain intensity and degradation-prone soils. In this study, we used isotopic measurements of soil water to better understand the main processes by which trees influence local soil water dynamics within a tropical pasture with scattered tree cover in the Copan River catchment, Honduras. We also determined the stable isotope signature of xylem water in grasses and trees to assess potential competition for water sources during the wet and dry seasons. During the wet season, when soil water availability was not limiting, both grasses and trees primarily utilized soil water near the soil surface (i.e., 0–10 cm). In contrast, during the dry season, we observed niche partitioning for water resources where grasses primarily utilized soil moisture at deeper soil depth (i.e., 90–100 cm) while trees relied heavily on groundwater. Moreover, isotopic data of soil water suggest that trees reduce evaporative water losses from the soil surface, as indicated by the lack of correlation between soil water content and lc-excess (line condition excess) values of surface soil water under trees, and enhance preferential flow as suggested by less negative lc-excess values under trees compared to open areas during the dry season. Taken together, our findings provide further support that trees can have positive effects on the local water balance with implication for landscape management, promoting the inclusion of scattered trees to provide water ecosystem services in silvopastoral systems, adding to other ecosystem services like biodiversity or carbon sequestration.
In tropical Central America, 74% (i.e., 84 million hectares) of the total agricultural land was used for meadows and pastures in 2019 (FAOSTAT, 2019), and this area is expected to increase by 32% by 2030 (Acosta and Díaz, 2014). At the same time, the sustainable use of pastures is at risk due to ongoing degradation (Szott et al., 2000; Muchagata and Brown, 2003), resulting in negative consequences on productivity, erosion, and water resources (Martínez and Zinck, 2004). Trees are commonly integrated into pastoral systems for various reasons including reducing soil degradation, increasing carbon stocks, providing protecting shade, and reducing financial risks due to farmers' income diversification (Erdmann, 2005; Verchot et al., 2007; Wassenaar et al., 2007; Harvey et al., 2014; Mulugeta, 2014). Despite their multiple benefits, trees are commonly assumed to decrease valuable soil and groundwater resources due to their higher interception and transpiration compared to shorter vegetation, leading to increased transport of water back to the atmosphere (Giambelluca, 2002, Calder, 2001; Fisher et al., 2009; Ellison et al., 2012, 2017). However, given the prevalent high rain intensity (Lal, 1990; Labrière et al., 2015) and severe soil degradation in many tropical ecosystems, trees could potentially have positive effects on the local water balance by reducing surface run-off and improving soil water infiltrability and groundwater recharge (Bargués Tobella et al., 2014; Benegas et al., 2014; Ilstedt et al., 2016; Bargués-Tobella et al., 2020). According to the optimum tree cover theory, intermediate tree densities on soils prone to degradation may maximize groundwater recharge, as the hydrologic gains attributed to tree cover can be proportionally higher than the additional losses from increased transpiration and interception (Ilstedt et al., 2016). Given the importance of water availability for human health, economy, ecosystem functioning, geophysical processes, and social-ecological system resilience at large (Cazenave et al., 2004; Mooney et al., 2005; Falkenmark et al., 2019), the lack of studies on water dynamics, including water source partitioning, in tropical pastures with open tree cover is alarming.
Water is a key resource in determining the structure and composition of vegetation in savanna-like ecosystems (van Wijk and Rodriguez-Iturbe, 2002). It is commonly thought that the coexistence of tree-grass communities is the result of niche partitioning whereby woody and herbaceous plant species do not compete for the same limiting resource, i.e., soil water (Jeltsch et al., 2000; Ward et al., 2013). One possible explanation for the lack of competition between trees (or shrubs) and grasses may be the result of differences in root morphology (Lehmann et al., 1998; Dulormne et al., 2004). Previous studies in temperate ecosystems have shown that trees tend to be more deep-rooted, accessing deeper water sources compared to grasses, which have more fibrous rooting systems and thus rely on soil moisture near the soil surface (Sala et al., 1989; Weltzin and McPherson, 1997; Sankaran et al., 2004; Nippert and Knapp, 2007; Ward et al., 2013). However, evidence for niche-partitioning in tropical and subtropical ecosystems is mixed, and there is disagreement about the extent to which trees and grasses partition soil moisture in these ecosystems (Verweij et al., 2011; Kambatuku et al., 2013; Kulmatiski and Beard, 2013; Ward et al., 2013). Moreover, seasonality may strongly influence the degree to which soil water is partitioned between trees and grasses. For example, in a savanna ecosystem in South Africa, trees used soil moisture near the soil surface (i.e., 25 cm depth) during the wet season and water from deeper depths in the dry season, whereas grasses accessed soil water from the topsoil layer throughout the year (Priyadarshini et al., 2015). In pastures, determining the sources of water for trees and grasses is highly relevant, as competition for water between trees and grasses can reduce grass growth and production (Dulormne et al., 2004).
The analysis of the hydrogen (δ2H) and oxygen (δ18O) stable isotope ratios of water is a useful tool to assess where individual plants are accessing water within the soil profile (Ehleringer and Dawson, 1992; Brunel et al., 1995; Querejeta et al., 2007). During plant water uptake and transport within woody tissues, the isotopic signature of plant xylem water is assumed to remain unaltered, thereby reflecting the various zone(s) and depth(s) from which plants extract soil water (White et al., 1985). As a result of evaporative water losses from the soil surface, water in the upper soil layers often becomes enriched in the heavy isotopes (2H and 18O), resulting in a vertical variation of soil water δ2H and δ18O throughout the soil profile (Barnes and Turner, 1998). Thus, the isotopic signature of potential soil water sources at different depths can be compared to the isotopic signature of plant xylem water to better understand the sources of water used by individual plants (Ehleringer and Dawson, 1992; Jackson et al., 1995; Bonal et al., 2000; Hasselquist et al., 2010; Bargués Tobella et al., 2017).
The isotopic signature of soil water can also be used to examine how plants influence local soil water dynamics. We recently developed a conceptual model that describes how the isotopic signature of soil water and variation in soil water content (SWC) can be used to better understand the relative influence of canopy cover on local soil water (Hasselquist et al., 2018). The model uses the relationship between soil moisture and lc-excess (line condition excess, Landwehr and Coplen, 2006) of surface soil water to tease apart different processes by which trees can influence local soil water dynamics through transpiration, canopy interception, and evaporation. Additionally, the isotopic signature of mobile soil water at deeper soil depths can be useful to differentiate between preferential and matrix flow (Brooks et al., 2010; Zhao et al., 2013; Evaristo et al., 2015; Bargués-Tobella et al., 2020). Under preferential flow, water moves quickly through the soil profile through macropores, thereby by-passing much of the soil matrix, and as a result, is subject to less evaporative water losses. Thereby, under preferential flow, the isotopic signature of deep soil water is more similar to the isotopic composition of recent precipitation (i.e., less negative lc-excess values). In contrast, more negative lc-excess values of deeper soil water would suggest matrix flow as a result of previously evaporated water slowly percolating downward through the soil profile (Hasselquist et al., 2018).
In this study, we measured soil moisture and the isotopic composition of soil and xylem water to assess how trees influence local water dynamics and the potential competition between grasses and trees for soil water within a pasture landscape in the Copan River catchment, Honduras. Measurements were made in both the wet and dry seasons to see how seasonality affects the role trees play in the local soil water dynamics. More specifically, we hypothesized that (1) grasses utilize soil water near the soil surface regardless of season, (2) trees use surface soil water during the wet season but shift to deeper water sources as the upper soil layers dry out during the dry season, and (3) soil water content is higher under trees compared to adjacent open areas (covered by grasses) because of reduced soil evaporation and greater soil infiltrability and preferential flow under trees.
We selected a study area in the Copan River catchment, a 620 km2 catchment located near the border between Honduras and Guatemala (14°94′N, 89°15′W) (Figure 1). Pastures are the predominant land use within the catchment, covering 30% of its area (CATIE-MESOTERRA, 2009). The soils are classified as Typic Argiustolls, according to the USDA Soil Taxonomy 1999. The textural class of the topsoil is sandy clay loam and sandy loam, and at deeper depths (> 1 m) it varies between loam, clay loam and sandy loam. The climate is described as tropical savanna according to the Köppen-Geiger classification system (Peel et al., 2007). Average annual precipitation is 1,772 mm based on data collected during the past 32 years (Victor López, personal communication), with a pronounced dry season between November and April (Figure 2).
Figure 1. Field site location in pasture landscapes in the Copan River catchment, Honduras. Yellow circles show the three sites: (1) El Malcote (Byrsonima crassifolia (L.) Kunth), (2) Sesesmiles (Ficus colubrinae Standl.), and (3) El Zapote (Lonchocarpus hedyosmus Mig.); white stars show the location of the two wells.
Figure 2. Mean monthly rainfall (on the right) and temperature (on the left) distribution (average 1990-2019), in the Copan River catchment, Honduras (Zepner et al., 2020).
Within the Copan River catchment, we selected three sites: El Malcote, Sesesmiles and El Zapote (Figure 1). In all cases, these sites were characterized by an agroforestry system that has been under cattle pasture for the past 20 years, with a ground cover of native and introduced grass species, including Cynodon dactylon (L.) Pers., Cynodon plectostachyus (K.Schum.) Pilg., Panicum maximum Jacq., and Brachiaria brizantha (A.Rich.) Stapf. Within the landscape, there are scattered clumps of native tree species with three trees per clump on average as well as living fences of introduced tree species (e.g., Gliricidia sepium (Jacq.) Walp.) to mark farm boundaries. We selected three sites that represented one of the three dominant tree species each, sampled as follows: site 1, El Malcote (Byrsonima crassifolia (L.) Kunth); site 2, Sesesmiles (Ficus colubrinae Standl.); and site 3, El Zapote (Lonchocarpus hedyosmus Mig.). Average tree density in the pasture landscape ranged between 5 and 7 trees ha−1, based on Google Earth images acquired in 2007. Although we identified representative grass species in the sites, but besides they were well mixed spatially, their differences were not statistically significative, thus, we pooled all species under the category of “grasses.” The root system of Cynodon dactylon mostly develops within 0–25 cm depth but can go as deep as 70–80 cm in sandy soils, with underground biomass mostly rhizomatous. Creeping solons of 0.5–1.5 m length, but can reach 20 m, spread rapidly with numerous culms (8–40) (Heuzé et al., 2015). While Brachiaria brizantha have deep roots (down to 2 m) and short rhizomes (Heuzé et al., 2016). And the roots of Panicum maximum are fibrous, long and and occasionally have rhizomes, which confer some drought tolerance; the roots are fibrous, long and gnarled and occasionally have rhizomes, which confer some drought tolerance (Peters et al., 2010).
The mean animal load was 1.8 AU ha−1 (Animal Unit equivalent to 400 kg live weight per ha) n Sesesmiles, where the reference for sustainable livestock management is between 1 and 1.3 AU ha-1 (Cristobal Villanueva, personal communication). At El Malcote and El Zapote we estimated that the animal load could be even greater. At all three sites, there were visible signs of soil degradation due to cattle trampling and overgrazing.
In each site, we collected three samples from three trees located within a clump, which were of the same species in each site, together with three samples of grasses located underneath the trees and another three samples of grasses in the adjacent open area at a distance from the center of the clump equivalent to one length of the tallest tree (ca. 20 m). Samples were collected in both the dry (January 2013) and wet (June 2013) seasons.
We randomly selected three trees per clump. For each selected tree, twig samples were collected from three locations in the tree crown at random cardinal directions. We cut suberized twigs, ~10 mm in diameter and 50–80 mm long, from individual trees. Twigs were collected at 150 cm height or from the lower branches in taller trees at three different locations within each tree canopy to minimize any bias based on location within the canopy.
Grass samples were collected also following random cardinal directions both below the tree canopy and in the open areas. We collected culms, the non-photosynthetic part of the stem near the junction with roots, from individual grasses.
Both collected twigs and culms were immediately placed in a capped vial, wrapped in parafilm and stored in a refrigerator (4°C) until water extraction for stable isotope analyses.
At the same time and location where grass samples were collected, we also collected soil samples using a soil core (8 cm diameter) to a depth of 100 cm. From individual soil cores, two samples were taken for water extraction and isotopic analyses; one corresponding to topsoil (0–10 cm depth), and the other to subsurface soil (90–100 cm depth). Freshly collected soil samples were placed in capped glass vials, wrapped in parafilm and refrigerated at 4°C until water extraction for isotopic analyses.
Rain water samples were collected during the rainy season of 2013 (from 64 events in total) in two open areas within the catchment using plastic funnels (25 cm in diameter) connected to opaque collection bottles. Water was collected from the collection bottles immediately after each rain event and placed in 20 ml bottles, sealed with parafilm, and stored in a refrigerator (4°C) until isotopic analysis.
Two wells of 3 m depth were installed to collect shallow groundwater samples; one well was located next to sites 1 and 2, whereas the second well was located closer to site 3 (Figure 1). Groundwater samples were collected using a stick fastened to a plastic bottle (20 ml) when water was available in the well, which was usually after heavy rain events.
A cryogenic vacuum distillation line (Ehleringer et al., 2000) was used to extract water from vegetation and soil samples following the procedure described in Bargués Tobella et al. (2017) and Hasselquist et al. (2018).
Gravimetric soil water content (SWC) was calculated as: [(fresh weight—dry weight)/dry weight x 100], where fresh and dry weight correspond to the sample weight before and after thorough water extraction (100 °C, 1.5 h), respectively. The isotopic composition of water extracted from soil and plant material was analyzed using Cavity Ring-Down Spectroscopy (CRDS) on a Picarro L2130-i isotopic water analyzer (Picarro Inc., Sunnyvale, CA, USA, Picarro, 2012) at the SLU Stable Isotope Laboratory (SSIL) at the Department of Forest Ecology and Management, Swedish University of Agricultural Sciences (SLU), Umeå. We use the delta notation (%0) to the Vienna Standard Mean Ocean Water (VSMOW) to express all isotopic values (van Geldern and Barth, 2012). We obtained an analytical precision of 0.05%0 for δ18O and 0.26%0 for δD, based on repeated analysis of control samples.
lc-excess values of soil water were calculated as described by Landwehr and Coplen (2004) to obtain a relative index of evaporation. To do this, we used the measured δ18O signature of soil water (topsoil water and at 100 cm depth) (δ18OM) and the Local Meteoric Water Line (LMWL) we obtained for Copan [δD = 8.05 (δ18O) + 16.55]. We then calculated the predicted δD signature (δDP) of each topsoil water sample as δDP = 8.05 δ18OM +16.55. Finally, we calculated the lc-excess values of our samples by subtracting the predicted δD signature from the measured one (lc-excess = δDM–δDP). Values close to zero indicate little difference between the samples and local precipitation, whereas more negative values indicate a greater degree of evaporation (Landwehr and Coplen, 2006). These analyses are based on the conceptual model on how trees can potentially influence soil water dynamics proposed by Hasselquist et al. (2018).
The proportion of different water sources used by trees and grasses was estimated using the IsoSource mixing model (Phillips and Gregg, 2003, available at http://www.epa.gov/wed/pages/models/stableIsotopes/isosource/isosource.htm). In this model, the mean δ18O signature of xylem water was compared to that of soil water at two different depth intervals (0–10 cm and 90–100 cm), and to that of shallow groundwater (water table at 3 m depth) to estimate the relative contribution of surface, subsurface and groundwater sources to water uptake by trees and grasses. Using an isotopic mass balance approach, this model provides the distribution and mean contribution from each potential water source. We used a source increment of 1% as suggested by Phillips and Gregg (2003) and a mass balance tolerance of 0.1%0. The mixture was defined for each vegetation type/location (tree, grass underneath tree, grass in open area) according to its mean δ18O xylem water signature at each site (3 replicates per site). We defined a total of 18 mixtures [3 sites x 3 vegetation types/locations x 2 seasons-dry and wet-].
Although we recognize the advances in the Bayesian mixing models which include posterior parameter estimates and the extent of uncertainty in source contributions to the mixture population (Semmens et al., 2013), we still rely in simplicity of earlier linear mixing models (e.g., IsoSource), which are easier to evaluate, such that if a consumer's isotopic signature is outside the mixing polygon bounding the proposed mixture sources, then mass balance cannot be established and there is no logical solution. Such assumption is not inherent in the Bayesian mixing models, because the source data are distributions not average values, and these models will quantify source contributions even when the solution is very unlikely (Smith et al., 2013). Also recognizing the limitations of IsoSource, Phillips and Gregg (2003) emphasized that the outputs of IsoSource should be characterized in terms of the distribution of the solutions, not just of a single value such as the mean or most likely value. For practicality, we choose the single isotope framework (δ18O), based on previous studies showing no significant differences using only δ2H and only δ18O by IsoSource model (Wang et al., 2019), and for practicality, we decide to use only d18O.
To test differences in soil moisture we used a general linear model (one way ANOVA) with SWC as response variable and season, soil depth and location (i.e., under trees and open areas) as fixed explanatory variables and site as a blocking factor. However, blocking for site was never significant in this or any other analysis, and we therefore removed it from all statistical analyses. We tested the relation between soil water content at 0–10 cm depth with lc-excess to assess different mechanisms by which trees may influence local soil water dynamics following the model of Hasselquist et al. (2018). This is a conceptual model able to explain that trees can directly affect surface soil moisture by either transpiration and/or interception of incoming precipitation. Surface soil moisture derived by changes in evaporative water losses from the soil surface is also affected by trees. Different isotopic fractionation processes that can be disentangled by examining the relationship between surface soil water content and the isotopic composition of surface soil water. The model also allows to assess different pathways in which water moves throughout the soil profile (i.e., matrix vs. preferential flow).
For this purpose, we used a general linear model (one way ANOVA)with lc-excess as response variable and season and location (under trees vs. open areas) as dependent variables together with the corresponding interactions. To assess the presence of preferential flow, we use a second GLM to check the relationship between lc-excess at 0–10 cm and lc-excess at 90–100 cm (topsoil vs. subsoil water lc-excess), again with season and location as additional dependent variables. To test if the proportion of water used from each of the three considered sources (i.e., 0–10 cm, 90–100 cm and groundwater) varied with season and vegetation type we used three general linear models with the mean percentage of uptake from each source as separate response variables and vegetation type/location (tree, grass under trees and grass in open areas), and season as explanatory fixed variables. All data were tested for normal distribution and homogeneous variance using the Shapiro-Wils test and the Levene statistic, respectively. We used InfoStat statistical software (National University of Cordoba- FCA-UNC, Argentina) for the analyses of mean proportional water uptake partitioning, and Minitab 19 (Kowalski and Montgomery, 2010) for the general linear regressions.
The dual-isotope plot diagram (Figure 3) confirms precipitation and groundwater signatures proximity, and the presence of evaporation and mixing processes given that soil water samples deviate from the GMWL, falling below it in most cases. Vegetation samples of trees and grasses underneath trees plotted mostly above the GMWL showing little overlap with soil samples.
During the dry season, mean SWC at 0–10 cm and 90–100 cm depth was ca. 1.3 times higher (p < 0.05) and 1.6 times higher (p < 0.05) under trees compared to the adjacent open areas, respectively (Figures 4A,B). In this study, open areas represent zones in between trees which are covered by grasses. In contrast, during the wet season, there was little difference in SWC between open areas and areas under trees at both 10 and 100 cm depth (Figures 4A,B).
Figure 4. Mean (±SE) soil water content at 0–10 cm (A) and 90–100 cm (B) under trees (white bars) and in adjacent open areas (gray bars) during the wet and dry season. Bars with different lowercase letters have statistically significant differences (p < 0.05).
During the wet season, both trees and grasses primarily utilized topsoil water, whereas during the dry season, they shifted to deeper water sources. The relative contribution of topsoil water to grasses water uptake was only ca. 25% on average during the dry season, a value significantly lower than that for the wet season with 62% on average (p < 0.001; Figure 5, top panel). In the dry season, grasses obtained 45% of their water from the subsoil, while trees relied mostly on groundwater (79 %) (Figure 5). The distribution of the mean fractional contribution of the three considered water sources to the water uptake of each sampled plant is shown in Figure 6.
Figure 5. Proportion of water uptake from the three considered water sources (0–10 cm depth, 90–100 cm depth, groundwater) by vegetation type/location in pasture landscapes in Copan, Honduras. White boxplots = trees, light gray=grasses in open areas, and dark gray = grasses underneath trees. Different letters at upper case show statistically significant differences between seasons, and at lower case, statistically significant differences between vegetation types/location (p < 0.05).
Figure 6. Summary distribution of the mean fractional contribution of each of the three considered water sources (0–10 cm depth, 90–100 cm depth, groundwater) to the water uptake of the 18 plants that were sampled (3 sites × 3 vegetation types/location (trees—T, grasses underneath tree -GuT, and grasses in open areas -G) x 2 seasons) in pasture landscapes in Copan, Honduras.
There was anearly significant positive relationship between SWC and lc-excess of topsoil water for treatment (under trees and open areas, p <0.09), but not for the season (p > 0.05). We also tested these relationships separately by treatment and season (Figure 7); Results showed no significant relationship between SWC and lc-excess under trees neither during the dry (p > 0.05) or wet seasons (p > 0.05). In the open areas, there was a significant positive relationship between SWC and lc-excess during the dry season (p < 0.05), but not during the wet season (p > 0.05).
Figure 7. Relationships between lc-excess of soil water and SWC at 0-10 cm depth under trees and open areas (squares) in a pasture landscapes in Copan, Honduras during the dry (A) and wet seasons (B).
In general, lc-excess values of bulk soil water samples did not differ between treatment (p > 0.05) or seasons (p > 0.05; Figure 8). However, the interaction between treatment and soil depth was nearly significant (p < 0.09, Table 1), indicating more negative lc-excess values near the surface compared with deeper soil depths under trees, with the opposite situation in open areas.
Figure 8. Mean ± SE lc-excess values of bulk soil water collected at different soil depths (0-10 cm vs.90-100 cm) under trees and in adjacent open areas in pasture landscapes in Copan, Honduras.
Table 1. Summary of the general linear model for preferential flow analysis (relationships between lc-excess values at different soil depth under trees and in open areas).
Forest hydrology models intended for policy decisions on tree-water interactions often only consider evapotranspiration effects of trees. It is also commonly assumed that enhanced transpiration by trees can reduce deep soil water drainage and thus groundwater recharge compared to croplands without trees (Jobbagy and Jackson, 2004). However, these generalizations may be biased since studies like ours from degraded seasonally dry tropical areas with an open tree cover are largely absent (Malmer et al., 2010; Ellison et al., 2017). Results from this study show that such assumptions oversimplify the impacts of trees on soil water dynamics in tropical agroforestry pastures with scattered trees. Specifically, we found that during the dry season, soil moisture was higher under tree canopies compared to open areas both at 0–10 and 90–100 cm depth. We also present soil isotopic data that suggests that evaporation of soil water is the main process influencing topsoil moisture, occurring when rainfall is low (dry season), as shown by the positive relationship between SWC and lc-excess of topsoil water in open areas, with greater isotopic enrichment of topsoil water in drier soils.
We used a combination of measurements, including stable isotopes of soil and plant xylem water, together with soil moisture at different depth intervals to gain insight on the main processes influencing soil water dynamics under trees in a tropical agroforestry pasture. Using the framework described in Hasselquist et al. (2018), we investigated potential relationships between soil water content and lc-excess of surface soil water as a way to gain a better understanding of how trees influence local soil water dynamics. Although only nearly significant (p < 0.09), we found a positive relationship between SWC and lc-excess of topsoil water in open areas during the dry season, suggesting that variation in surface soil water in open areas was largely driven by evaporation (Figure 7A). This relationship was not observed under trees, suggesting that, in this case, evaporative soil water losses were not the main driver influencing topsoil moisture. This, in turn, may help explain why SWC at 0–10 cm depth was ca. 1.3 times higher under trees compared to open areas during the dry season (Figure 4A). Similar results were attributed to shade tree effects by Akpo et al. (2005). It is well known that tree canopies drastically reduce incoming solar radiation in open grassland ecosystems (Belsky et al., 1989; Ko and Reich, 1993; Scholes and Archer, 1997) thereby lowering soil temperatures and consequently evaporative water losses from the soil surface (Jackson and Wallace, 1999; Lin, 2010). Other studies in savanna ecosystems in Africa (Georgiadis, 1989; Weltzin and Coughenour, 1990; Belsky et al., 1993), as well as in Central and South America (Kellman, 1979; Garcia Miragaya et al., 1994) have also reported improved soil water contents beneath isolated trees. During the wet season, although not significant, we found a negative relationship between SWC and lc-excess of surface soil water under trees, which according to the conceptual model described by Hasselquist et al. (2018) would suggest that canopy interception (Allen et al., 2014) plays an important role in the spatial variation of soil water dynamics in this ecosystem and may help explain the lack of differences in SWC under trees compared to open areas during the wet season.
Although not significant, we observed that lc-excess values of soil water were less negative under trees compared with open areas at the subsoil (Figure 8), which suggests a significant amount of recent rainwater quickly by-passing the topsoil thereby escaping evaporative water losses at the soil surface. Additionally, these results also suggest that trees lead to less evaporation of topsoil water. This finding supports the idea of enhanced preferential flow under trees compared to open areas, which is consistent with previous dye-tracing studies in the same pasture showing greater levels of macroporosity and occurrence of preferential flow in the vicinity of the trees (Benegas et al., 2014). Similar results have been observed in an agroforestry parkland in semiarid Burkina Faso, where the degree of preferential flow was highest close to trees, and minimal in large open areas (Bargués Tobella et al., 2014; Bargués-Tobella et al., 2020). Preferential flow is more easily initiated when the topsoil layer approaches near-saturated conditions (Alaoui et al., 2011) which in turn may explain the less negative lc-excess values of deep soil water under trees compared with open areas (Figure 8). Macroporosity and soil water holding capacity are enhanced by biological processes (i.e., bioturbation, release of organic acids, production of root exudates, etc) (Coleman et al., 1991; Rhoades, 1996; Lavelle et al., 2006; Villalobos-Vega et al., 2011; Beven and Germann, 2013), which in turn favors soil infiltration, thereby reducing surface runoff (Weiler and Naef, 2003). Therefore, in some ecosystems it has been shown that trees can enhance groundwater recharge and reduce surface runoff (Sandström, 1998; Bruijnzeel, 2004; van Dijk and Keenan, 2007; Xiong et al., 2008; Niemeyer et al., 2014; Ilstedt et al., 2016).
At the wet season, but in open areas, lc-excess of soil water collected at 100 cm, although no significantly different, was less negative compared to soil water collected at 10 cm depth. This result could imply the presence of preferential flow in open areas in the wet season, when water on the soil surface is more available. Streamflow and groundwater recharge are generated by non-uniform infiltration processes across the landscape, particularly at hillslope scales. Variables such as soil thickness, depth to water table, and rainfall characteristics may influence these processes (Berry et al., 2018).
In this study, we also determined the isotopic signature of plant xylem water to better understand the sources of water used by trees and grasses during the dry and wet season in a tropical agroforestry pasture. During the wet season, both trees and grasses primarily utilized soil water near the soil surface (i.e., 0–10 cm depth; Figure 5 top panel). However, during the dry season, both grass and trees utilized deeper water sources, with grasses primarily accessing water at deep soil depth (90–100 cm), and trees primarily utilizing groundwater (Figure 5 lower panel). This finding of different water sources for grasses and trees during the dry season supports Walter's two-layer hypothesis (Walter et al., 1971), which describes the below-ground partitioning of soil resources between woody and herbaceous vegetation. According to this hypothesis, grasses primarily use soil water near the soil surface because of their intense and shallow root system. In contrast, woody savannah trees tend to be deeper rooted, and thus primarily rely on soil moisture at depths below the rooting depths of grasses. Walter‘s hypothesis generally holds for dry savannahs, which is the ecosystem for which the hypothesis was developed, although it is important to point out that some studies do not support this hypothesis (Le Roux et al., 1995; Mordelet et al., 1997; Kulmatiski et al., 2010; Verweij et al., 2011; February et al., 2013). Our finding of trees utilizing deeper water sources compared to grasses during the dry season suggests niche partitioning when topsoil water availability becomes limiting, which is consistent with Walter's two-layer hypothesis and related studies (Strang, 1969; Sala et al., 1989; Ehleringer et al., 1991; Smit and Rethman, 2000; Miller et al., 2010; Rossatto et al., 2013). This finding is not consistent with previous studies, including tropical systems, that have shown similar water sources for trees and grasses (Le Roux et al., 1995; Seghieri et al., 1995; Mordelet et al., 1997; Le Roux and Bariac, 1998; Ludwig et al., 2003; February and Higgins, 2010; Verweij et al., 2011), thereby suggesting competition for soil water sources between trees and grasses (Walter et al., 1971; Walker et al., 1981; Walker and Noy-Meir, 1982). Even though competition is a likely interaction between trees and grasses, it often occurs only when soil water is not limiting (Maestre et al., 2005; Brooker et al., 2008), which is consistent with the findings from this study, which indicate that trees and grasses were primarily accessing shallow water sources during the wet season.
It is interesting to note that during the dry season, when SWC content near the soil surface was reduced, grasses also shifted to deeper water sources, suggesting a plasticity response in grass roots function in response to resource availability (Sala et al., 1989; Doussan et al., 2003). Among the grass species in this study, Cynodon spp. has the deepest root system, which can reach to 2 m depth in ecosystems characterized by drought periods with water limitations (Etemadi et al., 2005). Additionally, Panicum maximum, the most abundant grass in our system, has been reported to take up water from below 1 meter depth in primary forests and savanna-like abandoned pastures in eastern Amazon (Moreira et al., 2000). Brachiaria spp also have a rooting system that can penetrate depths > 1 m (Rattan and Stewart, 2013), with certain Brachiaria species having greater root biomass at deeper depth (i.e., B. humidicola and B. dictyoneura) making them better adapted to longer dry periods than other grass species (i.e., B. brizantha and B. decumbens) (Guenni et al., 2002). These morphological features of the grasses at our study site support our finding that grasses would be able to utilize deeper (i.e., 90–100 cm depth) water sources during the dry season. Moreover, these findings provide a mechanistic understanding of how these grasses can overcome seasonal water limitation, which is crucial for improving the foliage value and carrying capacity in these ecosystems (Sarmiento, 1992).
In conclusion, our results show that the positive coexistence of grasses and trees in the studied pasture landscape is possible through a spatiotemporal partitioning of water resources. During the wet season, when topsoil water is not limiting, both trees and grasses primarily utilized soil water near the soil surface (i.e., 0–10 cm depth). In contrast, during the dry season, both grasses and trees utilized deeper water sources, with grasses primarily accessing soil water at deeper soil depths (i.e., 90–100 cm) while trees relied heavily on groundwater. This finding of niche partitioning during the dry season provides a mechanistic understanding of the coexistence of trees and grass in pasture landscapes with scattered trees. Additionally, our results highlight that trees may have a positive effect on local soil water dynamics through reduced evaporative soil water losses during the dry season and enhanced preferential flow under trees. Nevertheless, we recognize that different species with higher water demands, and relatively greater planting densities may result in different outcomes. In any case, providing further support that trees can positively affect the local water balance, which has important implications for landscape management. Promoting the inclusion of scattered trees in pasture systems to provide water ecosystem services in the silvopastoral systems are adding to other ecosystem services like biodiversity or carbon sequestration.
The datasets presented in this study can be found in online repositories. The names of the repository/repositories and accession number(s) can be found below: https://doi.org/10.7910/DVN/C0EF3X.
LB, UI, and AM contributed to the conception and design of the work. LB acquired, organized the data base, performed statistical analysis, and wrote the manuscript. AB-T, NH, and UI revised critically the work and contributed with the manuscript drafting. All authors contributed to manuscript revision, read, and approved the submitted version.
We also acknowledge funding from the Swedish Research Council VR (2017-05566) and Formas (2017-00430).
The authors declare that the research was conducted in the absence of any commercial or financial relationships that could be construed as a potential conflict of interest.
All claims expressed in this article are solely those of the authors and do not necessarily represent those of their affiliated organizations, or those of the publisher, the editors and the reviewers. Any product that may be evaluated in this article, or claim that may be made by its manufacturer, is not guaranteed or endorsed by the publisher.
To CATIE's support through the project MESOTERRA, and its filed personnel. Thanks to Åsa Bengtsson and Jonas Lundholm for carrying out the isotopic analysis. NH would like to thank Formas (2016-20005) and the Swedish Research Council (2015-04791) for financial support.
Acosta, A., and Díaz, T. (2014). “Lineamientos de política para el desarrollo sostenible del sector ganadero,” in Oficina Subregional de la FAO para Mesoamérica. Ciudad de Panamá, 95.
Akpo, L., Goudiaby, V., Grouzis, M., and Le Houerou, H. (2005). Tree shade effects on soils and environmental factors in a savanna of Senegal. West Afr. J. Appl. Ecol. 7:45647. doi: 10.4314/wajae.v7i1.45647
Alaoui, A., Caduff, U., Gerke, H. H., and Weingartner, R. (2011). Preferential flow effects on infiltration and runoff in grassland and forest soils. Vadose Zone J. 10, 367–377. doi: 10.2136/vzj2010.0076
Allen, S. T., Brooks, J. R., Keim, R. F., Bond, B. J., and McDonnel,L, J. J. (2014). The role of pre-event canopy storage in throughfall and stemflow by using isotopic tracers. Ecohydrology 7, 858–868. doi: 10.1002/eco.1408
Bargués Tobella, A., Hasselquist, N. J., Bazié, H. R., Nyberg, G., Laudon, H., Bayala, J., et al. (2017). Strategies trees use to overcome seasonal water limitation in an agroforestry system in semiarid West Africa. Ecohydrology 10:e1808. doi: 10.1002/eco.1808
Bargués Tobella, A., Reese, H., Almaw, A., Bayala, J., Malmer, A., Laudon, H., et al. (2014). The effect of trees on preferential flow and soil infiltrability in an agroforestry parkland in semiarid Burkina Faso. Water Resourc. Res. 50, 3342–3354. doi: 10.1002/2013WR015197
Bargués-Tobella, A., Hasselquist, N. J., Bazié, H. R., Bayala, J., Laudon, H., Ilstedt, U., et al. (2020). Trees in African drylands can promote deep soil and groundwater recharge in a future climate with more intense rainfall. Land Degrad. Dev. 31, 81–95. doi: 10.1002/ldr.3430
Barnes, C. J., and Turner, J. V. (1998). “Isotopic exchange in soil water,” in Isotope Tracers in Catchment Hydrology. First, eds C. Kendall, and J. McDonnell (Oxford: Elsevier Science), 4.
Belsky, A. J., Amundson, R. G., Duxbury, J. M., Riha, S. J., Ali, A. R., and Mwonga, S. M. (1989). The effects of trees on their physical, chemical and biological environments in a semi-arid savanna in Kenya. J. Appl. Ecol. 26, 1005–1024. doi: 10.2307/2403708
Belsky, A. J., Mwonga, S. M., Amundson, R. G., Duxbury, J. M., and Ali, A. R. (1993). Comparative effects of isolated trees on their undercanopy environments in high- and low-rainfall savannas. J. Appl. Ecol. 30, 143–155. doi: 10.2307/2404278
Benegas, L., Ilstedt, U., Roupsard, O., Jones, J., and Malmer, A. (2014). Effects of trees on infiltrability and preferential flow in two contrasting agroecosystems in Central America. Agric. Ecosyst. Environ. 183, 185–196. doi: 10.1016/j.agee.2013.10.027
Berry, Z. C., Evaristo, J., Moore, G., Poca, M., Steppe, K., Verrot, L., et al. (2018). The two water worlds hypothesis: Addressing multiple working hypotheses and proposing a waym forward. Ecohydrology 2, 1–10. doi: 10.1002/eco.1843
Beven, K., and Germann, P. (2013). Macropores and water flow in soils revisited. Water Resourc. Res. 49, 3071–3092. doi: 10.1002/wrcr.20156
Bonal, D., Atger, C., Barigah, T. S., Ferhi, A., Guehl, J. M., and Ferry, B. (2000). Water acquisition patterns of two wet tropical canopy tree species of French Guiana as inferred from (H2O)-O-18 extraction profiles. Annal. For. Sci. 57, 717–724. doi: 10.1051/forest:2000152
Brooker, R. W., Maestre, F. T., Callaway, R. M., Lortie, C. L., Cavieres, L. A., Kunstler, G., et al. (2008). Facilitation in plant communities: the past, the present, and the future. J. Ecol. 96, 18–34. doi: 10.1111/j.1365-2745.2007.01295.x
Brooks, J. R., Barnard, H. R., Coulombe, R., and McDonnell, J. J. (2010). Ecohydrologic separation of water between trees and streams in a Mediterranean climate. Nat. Geosci. 3, 100–104. doi: 10.1038/ngeo722
Bruijnzeel, L. A. (2004). Hydrological functions of tropical forests: not seeing the soil for the trees? Agric. Ecosyst. Environ. 104, 185–228. doi: 10.1016/j.agee.2004.01.015
Brunel, J.-P., Walker, G. R., and Kennett-Smith, A. K. (1995). Field validation of isotopic procedures for determining sources of water used by plants in a semi-arid environment. J. Hydrol. 167, 351–368. doi: 10.1016/0022-1694(94)02575-V
Calder, I. R. (2001). Canopy processes: implications for transpiration, interception and splash induced erosion, ultimately for forest management and water resources. Plant Ecol. 153, 203–214. doi,: 10.1007/978-94-017-3606-0_16
Cazenave, A., Milly, P. C. D., Douville, H., Benveniste, J., Kosuth, P., and Lettenmaier, D. (2004). Space techniques used to measure change in terrestrial waters. EOS Trans. 85, 59–59. doi: 10.1029/2004EO060006
Coleman, D. C., Edwards, A. L., Belsky, A. J., and Mwonga, S. (1991). The distribution and abundance of soil nematodes in East African savannas. Biol. Fertil. Soils 12, 67–72. doi: 10.1007/BF00369390
Doussan, C., Pagès, L., and Pierret, A. (2003). Soil exploration and resource acquisition by plant roots: an architectural and modelling point of view. Agronomie 23, 419–431. doi: 10.1051/agro:2003027
Dulormne, M., Sierra, J., Bonhomme, R., and Cabidoche, Y. M. (2004). Seasonal changes in tree-grass complementarity and competition for water in a subhumid tropical silvopastoral system. Euro. J. Agron. 21, 311–322. doi: 10.1016/j.eja.2003.10.003
Ehleringer, J. R., and Dawson, T. E. (1992). Water uptake by plants: perspectives from stable isotope composition. Plant Cell Environ. 15, 1073–1082. doi: 10.1111/j.1365-3040.1992.tb01657.x
Ehleringer, J. R., Phillips, S. L., Schuster, W. S. F., and Sandquist, D. R. (1991). Differential utilization of summer rains by desert plants. Oecologia 88, 430–434. doi: 10.1007/BF00317589
Ehleringer, J. R., Roden, J., and Dawson, T. E. (2000). “Assessing ecosystem-level water relations through stable isotope ratio analyses,” in Methods in Ecosystem Science, eds, O. E. Sala, R. B. Jackson, H. A. Mooney, and R. W.Howarth (New York, NY: Springer).
Ellison, D., Futter, M. N., and Bishop, K. (2012). On the forest cover-water yield debate: from demand- to supply-side thinking. Glob. Change Biol. 18, 806–820. doi: 10.1111/j.1365-2486.2011.02589.x
Ellison, D., Morris, C. E., Locatelli, B., Sheil, D., Cohen, J., Murdiyarso, D., et al. (2017). Trees, forests and water: cool insights for a hot world. Glob. Environ. Change 43, 51–61. doi: 10.1016/j.gloenvcha.2017.01.002
Erdmann, T. (2005). “Agroforestry as a Tool for Forest Landscape Restoration,” in Forest Restoration in Landscapes: Beyond Planting Trees, eds S. Mansourian, D. Vallauri, and N. Dudley (New York, NY: Springer).
Etemadi, N., Khalighi, A., Razmjoo, K., Lessani, H., and Zamani, Z. (2005). Drought resistance of selected bermudagrass {Cynodon dactylon (L.) Pers.} accessions. Int. J. Agric. Biol. 7, 612–615. Available online at: http://www.ijab.org
Evaristo, J., Jasechko, S., and McDonnell, J. J. (2015). Global separation of plant transpiration from groundwater and streamflow. Nature 525, 91–94. doi: 10.1038/nature14983
Falkenmark, M., Wang-Erlandsson, L., and Rockstrom, J. (2019). Understanding of water resilience in the Anthropocene. J. Hydrol. 2, 1–13. doi: 10.1016/j.hydroa.2018.100009
FAOSTAT. (2019). Agricultural and permanent meadows and pasture lands for Central America from 2018-2019. Consulted. Available online at: http://www.fao.org/faostat/en/#data/RL/visualize.
February, E. C., and Higgins, S. I. (2010). The distribution of tree and grass roots in savannas in relation to soil nitrogen and water. South Afric. J. Bot. 76, 517–523. doi: 10.1016/j.sajb.2010.04.001
February, E. C., Higgins, S. I., Bond, W. J., and Swemmer, L. (2013). Influence of competition and rainfall manipulation on the growth responses of savanna trees and grasses. Ecology 94, 1155–1164. doi: 10.1890/12-0540.1
Fisher, J. B., Malhi, Y., Bonal, D., Da Rocha, H. R., De Araujo, A. C., Gamo, M., et al. (2009). The land–atmosphere water flux in the tropics. Glob. Change Biol. 15, 2694–2714. doi: 10.1111/j.1365-2486.2008.01813.x
Garcia Miragaya, J., Flores, S., and Chacon, N. (1994). Soil chemical-properties under individual evergreen and deciduous trees in a protected venezuelan savanna. Acta Oecologica-Int. J. Ecol. 15, 477–484.
Georgiadis, N. J. (1989). Microhabitat variation in an african savanna—effects of woody cover and herbivores in Kenya. J. Trop. Ecol. 5, 93–108. doi: 10.1017/S0266467400003254
Giambelluca, T. W. (2002). Hydrology of altered tropical forest. Hydrol. Process. 16, 1665–1669. doi: 10.1002/hyp.5021
Guenni, O., Marín, D., and Baruch, Z. (2002). Responses to drought of five Brachiaria species. I. Biomass production, leaf growth, root distribution, water use and forage quality. Plant Soil 243, 229–241. doi: 10.1023/A:1019956719475
Harvey, C. A., Chacón, M., Donatti, C. I., Garen, E., Hannah, L., Andrade, A., et al. (2014). Climate-smart landscapes: opportunities and challenges for integrating adaptation and mitigation in tropical agriculture. Conserv. Lett. 7, 77–90. doi: 10.1111/conl.12066
Hasselquist, N. J., Allen, M. F., and Santiago, L. S. (2010). Water relations of evergreen and drought-deciduous trees along a seasonally dry tropical forest chronosequence. Oecologia 164, 881–890. doi: 10.1007/s00442-010-1725-y
Hasselquist, N. J., B, L., Roupsard, O., Malmer, A., and Ilstedt, U. (2018). Canopy cover effects on local soil water dynamics in a tropical agroforestry system: evaporation drives soil water isotopic enrichment. Hydrol. Process. 32, 994–1004. doi: 10.1002/hyp.11482
Heuzé, V., Tran, G., Delagarde, R., and Lebas, F. (2015). Bermuda grass (Cynodon dactylon). Feedipedia, a programme by INRAE, CIRAD, AFZ and FAO. Available online at: https://www.feedipedia.org/node/471 (accessed October 20, 2015).
Heuzé, V., Tran, G., Sauvant, D., and Lebas, F. (2016). Bread grass (Brachiaria brizantha). Feedipedia, a programme by INRAE, CIRAD, AFZ and FAO. Available online at: https://www.feedipedia.org/node/490. (accessed September 9, 2016).
Ilstedt, U., Bargués Tobella, A., Bazié, H. R., Bayala, J., Verbeeten, E., Nyberg, G., et al. (2016). Intermediate tree cover can maximize groundwater recharge in the seasonally dry tropics. Sci. Rep. 6:21930. doi: 10.1038/srep21930
Jackson, N. A., and Wallace, J. S. (1999). Soil evaporation measurements in an agroforestry system in Kenya. Agric. For. Meteorol. 94, 203–215. doi: 10.1016/S0168-1923(99)00013-1
Jackson, P. C., Cavelier, J., Goldstein, G., Meinzer, F. C., and Holbrook, N. M. (1995). Partitioning of water resources among plants of a lowland tropical forest. Oecologia 101, 197–203. doi: 10.1007/BF00317284
Jeltsch, F., Weber, G., and Grimm, V. (2000). Ecological buffering mechanisms in savannas: a unifying theory of long-term tree-grass coexistence. Plant Ecol. 150, 161–171. doi: 10.1023/A:1026590806682
Jobbagy, E. G., and Jackson, R. B. (2004). Groundwater use and salinization with grassland afforestation. Glob. Change Biol. 10, 1299–1312. doi: 10.1111/j.1365-2486.2004.00806.x
Kambatuku, J. R., Crame,R, M. D., and Ward, D. (2013). Overlap in soil water sources of savanna woody seedlings and grasses. Ecohydrology 6, 464–473. doi: 10.1002/eco.1273
Kellman, M. (1979). Soil enrichment by neotropical savanna trees. J. Ecol. 67, 565–577. doi: 10.2307/2259112
Ko, L. J., and Reich, P. B. (1993). Oak tree effects on soil and herbaceous vegetation in savannas and pastures in Wisconsin. Am. Midland Nat. 24, 31–42. doi: 10.2307/2426272
Kowalski, S. M., and Montgomery, D. C. (2010). Design and analysis of experiments: Minitab Companion. New York, NY: John Wiley and Sons, Inc.
Kulmatiski, A., and Beard, K. H. (2013). Woody plant encroachment facilitated by increased precipitation intensity. Nat. Clim. Chang. 3, 833–837. doi: 10.1038/nclimate1904
Kulmatiski, A., Beard, K. H., Verweij, R. J., and February, E. C. (2010). A depth-controlled tracer technique measures vertical, horizontal and temporal patterns of water use by trees and grasses in a subtropical savanna. New Phytol. 188, 199–209. doi: 10.1111/j.1469-8137.2010.03338.x
Labrière, N., Locatelli, B., Laumonier, Y., Freycon, V., and Bernoux, M. (2015). Soil erosion in the humid tropics: a systematic quantitative review. Agric. Ecosyst. Environ. 203, 127–139. doi: 10.1016/j.agee.2015.01.027
Landwehr, J., and Coplen, T. (2004). “Line-conditioned excess: a new method for characterizing stable hydrogen and oxygen isotope ratios in hydrologic systems,” Monaco: Paper presented at the Isotopes in Environmental Studies Aquatic Forum.
Landwehr, J., and Coplen, T. (2006). “Line-conditioned excess: a new method for characterizing stable hydrogen and oxygen isotope ratios in hydrologic systems,: in International Conference on Isotopes in Environmental Studies (IAEA Vienna), 132–135.
Lavelle, P., Decaëns, T., Aubert, M., Barot, S., Blouin, M., Bureau, F., et al. (2006). Soil invertebrates and ecosystem services. Eur. J. Soil Biol. 42, S3–S15. doi: 10.1016/j.ejsobi.2006.10.002
Le Roux, X., and Bariac, T. (1998). Seasonal variations in soil, grass and shrub water status in a West African humid savanna. Oecologia 113, 456–466. doi: 10.1007/s004420050398
Le Roux, X., Bariac, T., and Mariotti, A. (1995). Spatial partitioning of the soil water resource between grass and shrub components in a West African humid savanna. Oecologia 104, 147–155. doi: 10.1007/BF00328579
Lehmann, J., Peter, I., Steglich, C., Gebauer, G., Huwe, B., and Zech, W. (1998). Below-ground interactions in dryland agroforestry. For. Ecol. Manage. 111, 157–169. doi: 10.1016/S0378-1127(98)00322-3
Lin, B. B. (2010). The role of agroforestry in reducing water loss through soil evaporation and crop transpiration in coffee agroecosystems. Agric. Forest Meteorol. 150, 510–518. doi: 10.1016/j.agrformet.2009.11.010
Ludwig, F., Dawson, T. E., De Kroon, H., Berendse, F., and Prins, H. H. T. (2003). Hydraulic lift in Acacia tortilis trees on an East African savanna. Oecologia 134, 293–300. doi: 10.1007/s00442-002-1119-x
Maestre, F. T., Valladares, F., and Reynolds, J. F. (2005). Is the change of plant–plant interactions with abiotic stress predictable? a meta-analysis of field results in arid environments. J. Ecol. 93, 748–757. doi: 10.1111/j.1365-2745.2005.01017.x
Malmer, A., Murdiyarso, D., Bruijnzeel, L. A., and Ilstedt, U. (2010). Carbon sequestration in tropical forests and water: a critical look at the basis for commonly used generalizations. Glob. Chang. Biol. 16, 599–604. doi: 10.1111/j.1365-2486.2009.01984.x
Martínez, L. J., and Zinck, J. A. (2004). Temporal variation of soil compaction and deterioration of soil quality in pasture areas of Colombian Amazonia. Soil illage Res. 75, 3–18. doi: 10.1016/j.still.2002.12.001
Miller, G. R., Chen, X., Rubin, Y., MA, S., and Baldocchi, D. D. (2010). Groundwater uptake by woody vegetation in a semiarid oak savanna. Water Resour. Res. 46. doi: 10.1029/2009WR008902
Mooney, H., Cropper, A., and Reid, W. (2005). Confronting the human dilema: How can ecosystems provide sustainable services to benefit society? Nature 434, 561–562. doi: 10.1038/434561a
Mordelet, P., Menaut, J.-C., and Mariotti, A. (1997). Tree and grass rooting patterns in an African humid savanna. J. Vegetat. Sci. 8, 65–70. doi: 10.2307/3237243
Moreira, M. Z., da, S. L., Sternberg, L., and Nepstad, D. C. (2000). Vertical patterns of soil water uptake by plants in a primary forest and an abandoned pasture in the eastern Amazon: an isotopic approach. Plant Soil 222, 95–107. doi: 10.1023/A:1004773217189
Muchagata, M., and Brown, K. (2003). Cows, colonists and trees: rethinking cattle and environmental degradation in Brazilian Amazonia. Agric. Syst. 76, 797–816. doi: 10.1016/S0308-521X(02)00015-X
Mulugeta, G. (2014). Evergreen agriculture: agroforestry for food security and climate change resilience. J. Nat. Sci. Res. 4, 80–90. ISSN 2224-3186 (Paper) ISSN 2225-0921 (Online).
Niemeyer, R. J., Fremier, A. K., Heinse, R., Chavez, W., and Declerck, F. A. J. (2014). Woody vegetation increases saturated hydraulic conductivity in dry tropical Nicaragua. Vadose Zone J. 13:0025. doi: 10.2136/vzj2013.01.0025
Nippert, J. B., and Knapp, A. K. (2007). Linking water uptake with rooting patterns in grassland species. Oecologia 153, 261–272. doi: 10.1007/s00442-007-0745-8
Peel, M. C., Finlayson, B. L., and McMahon, T. A. (2007). Updated world map of the Köppen-Geiger climate classification. Hydrol. Earth Syst. Sci., 11, 1633–1644. doi: 10.5194/hess-11-1633-2007
Peters, M., Franco, L. H., Schmidt, A., and Hincapié, B. (2010). Especies Forrajeras Multipropósito Opciones para Productores del Trópico Americano CIAT-Cali. Colombia. 66.
Phillips, D. L., and Gregg, J. W. (2003). Source partitioning using stable isotopes: coping with too many sources. Oecologia 136, 261–269. doi: 10.1007/s00442-003-1218-3
Picarro, P. (2012). Micro-Combustion ModuleTM (MCM): Elimination of Organics. Datasheet (accessed August 28, 2015).
Priyadarshini, K. V. R., Prins, H. H. T., De Bie, S., Heitkönig, I. M. A., Woodborne, S., Gort, G., et al. (2015). Seasonality of hydraulic redistribution by trees to grasses and changes in their water-source use that change tree–grass interactions. Ecohydrology 16:24. doi: 10.1002/eco.1624
Querejeta, J. I., EstradA-Medina, H., Allen, M. F., and Jimenez-Osornio, J. J. (2007). Water source partitioning among trees growing on shallow karst soils in a seasonally dry tropical climate. Oecologia 152, 26–36. doi: 10.1007/s00442-006-0629-3
Rattan, L., and Stewart, B. A. (2013). Principles of Sustainable Soil Management in Agroecosystems. Boca Raton: Press-Taylor and Francis Group.
Rhoades, C. (1996). Single-tree influences on soil properties in agroforestry: lessons from natural forest and savanna ecosystems. Agroforestr. Syst. 35, 71–94. doi: 10.1007/BF02345330
Rossatto, D. R., Da SilvEIRA Lobo Sternberg, L., and Franco, A. C. (2013). The partitioning of water uptake between growth forms in a Neotropical savanna: do herbs exploit a third water source niche? Plant Biol. 15, 84–92. doi: 10.1111/j.1438-8677.2012.00618.x
Sala, O. E., Golluscio, R. A., Lauenroth, W. K., and Soriano, A. (1989). Resource partitioning between shrubs and grasses in the Patagonian steppe. Oecologia 81, 501–505. doi: 10.1007/BF00378959
Sandström, K. (1998). Can forests “provide” water: widespread myth or scientific reality? Ambio 27, 132–138.
Sankaran, M., Ratnam, J., and Hanan, N. P. (2004). Tree–grass coexistence in savannas revisited—insights from an examination of assumptions and mechanisms invoked in existing models. Ecol. Lett. 7, 480–490. doi: 10.1111/j.1461-0248.2004.00596.x
Sarmiento, G. (1992). Adaptive strategies of perennial grasses in South American savannas. J. Vegetat. Sci. 3, 325–336. doi: 10.2307/3235757
Scholes, R. J., and Archer, S. R. (1997). Tree-grass interactions in savannas. Annu. Rev. Ecol. Syst. 28, 517–544. doi: 10.1146/annurev.ecolsys.28.1.517
Seghieri, J., CH, F., and Pontanier, R. (1995). Plant Phenology in relation to water availability: herbaceous and woody species in the savannas of northern cameroon. J. Trop. Ecol. 11, 237–254. doi: 10.1017/S0266467400008713
Semmens, B. X., Ward, E. J., Parnell, A. C., Phillips, D. L., Bearhop, S., Inger, R., et al. (2013). Statistical basis and outputs of stable isotope mixing models: comment on Fry. Mar. Ecol. Prog. Ser. 490, 285–289. doi: 10.3354/meps10535
Smit, G. N., and Rethman, N. F. G. (2000). The influence of tree thinning on the soil water in a semi-arid savanna of southern Africa. J. Arid Environ. 44, 41–59. doi: 10.1006/jare.1999.0576
Smith, J. A., Mazumder, D., Suthers, I. M., and Taylor, M. D. (2013). To fit or not to fit: evaluating stable isotope mixing models using simulated mixing polygons. Methods Ecol. Evol. 4, 612–618. doi: 10.1111/2041-210X.12048
Strang, R. M. (1969). Soil moisture relations under grassland and under woodland in the Rhodesian highveld—Com. For. Rev. 48, 26–40.
Szott, L., Ibrahim, M., and Beer, J. (2000). The Hamburger Connection Hangover: Cattle, Pasture Land Degradation and Alternative Land Use in Central America. CATIE, 7.
USDA (1999). “Soil taxonomy: a basic system of soil classification for making and interpreting soil surveys,” in Agriculture (Washington, DC: Department of Agriculture).
van Dijk, A., and Keenan, R. J. (2007). Planted forests and water in perspective. For. Ecol. Manage. 251, 1–9. doi: 10.1016/j.foreco.2007.06.010
van Geldern, R., and Barth, J. A. C. (2012). Optimization of instrument setup and post-run corrections for oxygen and hydrogen stable isotope measurements of water by isotope ratio infrared spectroscopy (IRIS). Limnol. Oceanography-Methods 10, 1024–1036. doi: 10.4319/lom.2012.10.1024
van Wijk, M. T., and Rodriguez-Iturbe, I. (2002). Tree-grass competition in space and time: Insights from a simple cellular automata model based on ecohydrological dynamics. Water Resour. Res. 38, 18–11. doi: 10.1029/2001WR000768
Verchot, L. V., Van Noordwijk, M., Kandji, S., Tomich, T., Ong, C., Albrecht, A., et al. (2007). Climate change: linking adaptation and mitigation through agroforestry. Mitigat. Adaptat. Strat. Glob. Change 12, 901–918. doi: 10.1007/s11027-007-9105-6
Verweij, R. J. T., Higgins, S. I., Bond, W. J., and February, E. C. (2011). Water sourcing by trees in a mesic savanna: Responses to severing deep and shallow roots. Environ. Exp. Bot. 74, 229–236. doi: 10.1016/j.envexpbot.2011.06.004
Villalobos-Vega, R., Goldstein, G., Haridasan, M., Franco, A. C., Miralles-Wilhelm, F., Scholz, F. G., et al. (2011). Leaf litter manipulations alter soil physicochemical properties and tree growth in a Neotropical savanna. Plant Soil 346, 385–397. doi: 10.1007/s11104-011-0860-5
Walker, B. H., Ludwig, D., Holling, C. S., and Peterman, R. M. (1981). Stability of semi-arid savanna grazing systems. J. Ecol. 69, 473–498. doi: 10.2307/2259679
Walker, B. H., and Noy-Meir, I. (1982). Aspects of the stability and resilience of savanna ecosystems. Ecolo. Stud. 42, 556–590. doi: 10.1007/978-3-642-68786-0_26
Walter, H., Burnett, J. H., and Mueller-Dombois, D. (1971). Ecology of Tropical and Subtropical Vegetation. Edinburg: Oliver and Boyd.
Wang, J., Lu, N., and Fu, B. (2019). Inter-comparison of stable isotope mixing models for determining plant water source partitioning. Sci. Total Environ. 666, 685–693. doi: 10.1016/j.scitotenv.2019.02.262
Ward, D., Wiegand, K., and Getzin, S. (2013). Walter's two-layer hypothesis revisited: back to the roots! Oecologia 172, 617–630. doi: 10.1007/s00442-012-2538-y
Wassenaar, T., Gerber, P., Verburg, P. H., Rosales, M., Ibrahim, M., and Steinfeld, H. (2007). Projecting land use changes in the Neotropics: the geography of pasture expansion into forest. Glob. Environ. Change 17, 86–104. doi: 10.1016/j.gloenvcha.2006.03.007
Weiler, M., and Naef, F. (2003). Simulating surface and subsurface initiation of macropore flow. J. Hydrol. 273, 139–154. doi: 10.1016/S0022-1694(02)00361-X
Weltzin, J. F., and Coughenour, M.B. (1990). Savanna tree influence on understory vegetation and soil nutrients in northwestern Kenya. J. Vegeta. Sci. 1, 325-334. doi: 10.2307/3235707
Weltzin, J. F., and McPherson, G. R. (1997). Spatial and temporal soil moisture resource partitioning by trees and grasses in a temperate savanna, Arizona, USA. Oecologia 112, 156–164. doi: 10.1007/s004420050295
White, J. W. C., Cook, E. R., Lawrence, J. R., and Broecke,R, W. S. (1985). The D/H ratios of sap in trees—implications for water sources and tree-ring D/H ratios. Geochim. Cosmochim. Acta 49, 237–246. doi: 10.1016/0016-7037(85)90207-8
Xiong, Y., Xia, H., Li, Z. A., Cai, X. A., and Fu, S. (2008). Impacts of litter and understory removal on soil properties in a subtropical Acacia mangium plantation in China. Plant Soil 304, 179–188. doi: 10.1007/s11104-007-9536-6
Zepner, L., Karrasch, P., Wiemann, F., and Bernard, L. (2020). ClimateCharts.net—an interactive climate analysis web platform. Int. J. Digit. Earth 18:129112. doi: 10.1080/17538947.2020.1829112
Keywords: niche partitioning, groundwater recharge, land management, ecosystem, tree-grass coexistence, isosource mixing model
Citation: Benegas L, Hasselquist N, Bargués-Tobella A, Malmer A and Ilstedt U (2021) Positive Effects of Scattered Trees on Soil Water Dynamics in a Pasture Landscape in the Tropics. Front. Water 3:736824. doi: 10.3389/frwa.2021.736824
Received: 05 July 2021; Accepted: 03 September 2021;
Published: 20 October 2021.
Edited by:
María Poca, CONICET Institute of Applied Mathematics San Luis (IMASL), ArgentinaReviewed by:
Pedro A. Hervé-Fernández, Universidad Adolfo Ibáñez, ChileCopyright © 2021 Benegas, Hasselquist, Bargués-Tobella, Malmer and Ilstedt. This is an open-access article distributed under the terms of the Creative Commons Attribution License (CC BY). The use, distribution or reproduction in other forums is permitted, provided the original author(s) and the copyright owner(s) are credited and that the original publication in this journal is cited, in accordance with accepted academic practice. No use, distribution or reproduction is permitted which does not comply with these terms.
*Correspondence: Laura Benegas, bGF1cmEuYmVuZWdhc0BjYXRpZS5hYy5jcg==
Disclaimer: All claims expressed in this article are solely those of the authors and do not necessarily represent those of their affiliated organizations, or those of the publisher, the editors and the reviewers. Any product that may be evaluated in this article or claim that may be made by its manufacturer is not guaranteed or endorsed by the publisher.
Research integrity at Frontiers
Learn more about the work of our research integrity team to safeguard the quality of each article we publish.