- 1Department of Agronomy, Kansas State University, Manhattan, KS, United States
- 2Department of Plant and Soil Sciences, Oklahoma State University, Stillwater, OK, United States
- 3Radiation Detection Technologies, Inc., Manhattan, KS, United States
- 4Semiconductor Materials and Radiological Technologies (S.M.A.R.T.) Laboratory, Kansas State University, Manhattan, KS, United States
During the past decade, cosmic-ray neutron sensing technology has enabled researchers to reveal soil moisture spatial patterns and to estimate landscape-average soil moisture for hydrological and agricultural applications. However, reliance on rare materials such as helium-3 increases the cost of cosmic-ray neutron probes (CRNPs) and limits the adoption of this unique technology beyond the realm of academic research. In this study, we evaluated a novel lower cost CRNP based on moderated ultra-thin lithium-6 foil (Li foil system) technology against a commercially-available CRNP based on BF3 (boron trifluoride, BF-3 system). The study was conducted in a cropped field located in the Konza Prairie Biological Station near Manhattan, Kansas, USA (325 m a.s.l.) from 10 April 2020 to 18 June 2020. During this period the mean atmospheric pressure was 977 kPa, the mean air relative humidity was 70%, and the average volumetric soil water content was 0.277 m3 m−3. Raw fast neutron counts were corrected for atmospheric pressure, atmospheric water vapor, and incoming neutron flux. Calibration of the CRNPs was conducted using four intensive field surveys (n > 120), in combination with continuous observations from an existing array of in situ soil moisture sensors. The time series of uncorrected neutron counts of the Li foil system was highly correlated (r2 = 0.91) to that of the BF-3 system. The Li foil system had an average of 2,250 corrected neutron counts per hour with an uncertainty of 2.25%, values that are specific to the instrument size, detector configuration, and atmospheric conditions. The estimated volumetric water content from the Li foil system had a mean absolute difference of 0.022 m3 m−3 compared to the value from the array of in situ sensors. The new Li foil detector offers a promising lower cost alternative to existing cosmic-ray neutron detection devices used for hectometer-scale soil moisture monitoring.
Introduction
The strong inverse relationship between epithermal neutron intensity 1–2 m above the ground and the hydrogen pool in the upper decimeters of the soil has provided the basis for an emerging non-invasive method for quantifying soil moisture at the hectometer horizontal scale. Cosmic-ray neutron probes (CRNPs) have a large (~12 hectares) footprint that fills the spatial niche between remote sensing soil moisture products (i.e., several kilometers) and traditional point-level (i.e., several decimeters) soil moisture sensors (Zreda et al., 2008; Desilets et al., 2010; Bogena et al., 2015). Stationary CRNPs have been widely adopted to quantify soil moisture conditions in forests (Bogena et al., 2013; Lv et al., 2014; Heidbüchel et al., 2016; Vather et al., 2019, 2020), cropland (Rivera Villarreyes et al., 2011; Zhu et al., 2015) and grassland (Hawdon et al., 2014; Montzka et al., 2017), and have also been used in data assimilation studies to improve soil moisture estimates of a land surface model at the watershed level (Shuttleworth et al., 2013). Because of the large sensing footprint and non-invasive nature of this technology, large-scale (e.g., catchment scale and national scale) environmental monitoring networks in the USA (Zreda et al., 2012), Australia (Hawdon et al., 2014), and the UK (Evans et al., 2016) have adopted CRNPs for monitoring soil moisture conditions. The large sensing footprint and the possibility to develop powerful roving detectors (Desilets et al., 2010) have also enabled the monitoring of soil moisture across landscapes with intermixed land covers with potential for validating remote sensing soil moisture products (Chrisman and Zreda, 2013; Dong et al., 2014; Franz et al., 2015; Montzka et al., 2017; Dong and Ochsner, 2018). However, a primary barrier for the widespread adoption of CRNPs for soil moisture sensing in and beyond the realm of academic research is the cost of the instrument.
Most CRNPs used in soil moisture research consist of gas-filled proportional counters containing either 3He or BF3. Proportional counters are tubes containing a thin axial wire that transmits electrical pulses generated by the interaction of thermal neutrons with the gas in the tube (Zreda et al., 2012; Schrön et al., 2018). Those pulses are translated by pulse counters into counts of thermal neutrons. These proportional counters are typically shielded by a layer of high density polyethylene (HDPE) to suppress incoming thermal neutrons and moderate epithermal neutrons so that they enter the tube as thermal neutrons (Köhli et al., 2018). The limited global supply of 3He, which is a byproduct of nuclear weapons, and the increased demand for this rare isotope from homeland security, nuclear safeguards, oil well exploration, and medical applications (Shea and Morgan, 2011) have dramatically decreased the stock of 3He and consequently elevated the cost of 3He by about 10-fold. Therefore, there is a substantial interest to find readily available and affordable alternatives for neutron detection (Nelson et al., 2012; Weimar et al., 2020). The use of BF3 proportional counters is perhaps the most common alternative to helium-based neutron detectors for soil moisture monitoring in agricultural and hydrological applications (Hawdon et al., 2014; Franz et al., 2015; Evans et al., 2016). But recently, researchers have investigated the use of more affordable sensing technologies for cosmic-ray neutrons detection. For instance, a group of researchers in Italy assembled commercially-available plastic scintillators, flexible neutron detectors, and photomultipliers to create a device called Finapp that is capable of detecting epithermal neutron intensities and gamma-rays for soil moisture monitoring in agricultural fields (Stevanato et al., 2019). Similarly, a group of researchers in Germany have developed stationary and roving CRNPs based on modular boron-lined neutron detectors (Weimar et al., 2020) for monitoring of soil moisture. Boron-lined proportional counter tubes are highly modular and can be used to create systems with high counting rates.
Recently, detectors made from ultra-thin (~30–120 μm) 6Li-enriched metal foils between multiple electrodes have shown promise as a viable alternative to 3He proportional counters (Nelson et al., 2012, 2015; Montag et al., 2019). Lithium-6 is a metal reactive to neutrons (see Nelson et al., 2012 for detailed nuclear reactions), and new lithium foil manufacturing technologies can be leveraged from the actively growing lithium battery industry. This presents a unique opportunity to adapt high-efficiency and low-cost Li foil multi-wire proportional counters for the sensing of field-scale soil moisture (Fersch et al., 2020). The objectives of this research were to (i) assess the ability of a Li foil CRNP to measure field-level soil moisture and (ii) compare the Li foil technology against a commercially-available BF-3 neutron detection system and an array of permanent soil moisture sensors.
Materials and Methods
Study Area
The study was conducted in a cropped field located in the Konza Prairie Biological Station (39.110 N, −96.613 E, altitude 325 m a.s.l.) near Manhattan, KS, USA. The field has an area of 13 hectares and has been managed using conventional tillage for over a decade. The soil is predominantly mapped as the Chase series (Fine, smectitic, mesic Aquertic Argiudolls), rarely flooded with <1% slope. Bulk density by the core method and particle size analysis using the hydrometer method (Gavlak et al., 2003) were determined from six undisturbed soil samples randomly distributed across the field. The average bulk density was 1.33 g cm−3 (SD = 0.036 g cm−3) and the soil textural class was silty clay loam. The field-average clay content in the top 12 cm was 38% (SD = 6%) and the average sand content was 10% (SD = 1%). During the study period, the vegetation at the site was winter wheat (Triticum aestivum L.) (Figure 1), which was planted in October 2019, and was harvested at the end of June 2020. This field is also part of the National Ecological Observatory Network (NEON, KONA site), from which we obtained precipitation, dry biomass at the time of harvest, and soil moisture records (NEON data products are detailed in Supplementary Table 1).
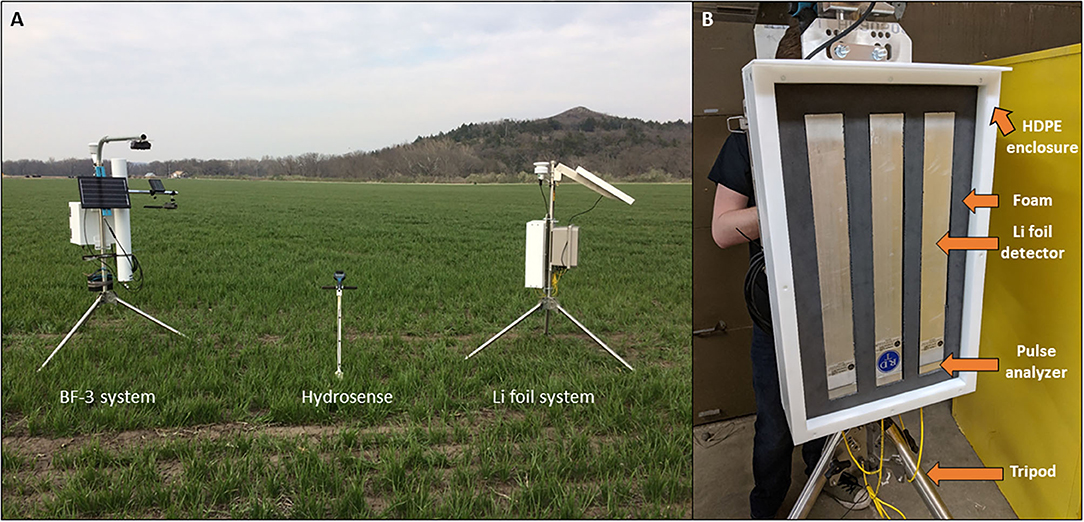
Figure 1. (A) Boron trifluoride system (left), Hydrosense hand-held soil moisture sensor (center), and Li foil system (right) in a field planted with winter wheat at the Konza Biological Research Station near Manhattan, Kansas, USA. The two systems were located about 3.5 m apart near the center of the field. (B) Front view of the Li foil system mounted on a tripod without the front panel of the moderating high density polyethylene (HDPE) enclosure. The image shows the three Li foil modules embedded in vibration dampening foam and the 2.5 cm thick, weatherproof HDPE enclosure. The enclosure is 60 cm in height, 30 cm wide, and 15 cm deep.
Cosmic-Ray Neutron Detectors
Hourly cosmic-ray neutron intensities and environmental conditions were monitored from 10 April 2020 to 18 June 2020 using two stationary CRNPs. The two stationary CRNPs were co-located (i.e., 3.5 m apart) near the center of the field (Figures 1A, 2) to ensure that most of the sensing footprint of each device was within the field boundaries. The first CRNP consisted of a single gas-filled tube with Boron-10 trifluoride (10BF3, BF-3 system from now on) (Model CRS-1000B, Hydroinnova LLC, Albuquerque, NM) shielded by a high-density polyethylene and connected to a neutron pulse module and a datalogger (Q-NPM and Q-DL-2100, Quaesta Instruments LLC, Tucson, AZ, USA). The BF-3 system was equipped with an air temperature and relative humidity sensor (Model CS215, Campbell Scientific), two internal barometers, and a cellular modem for data telemetry. The second CRNP consisted of three individual modules of 6Li-enriched foils (Li foil system from now on) (Radiation Detection Technologies, Inc., Manhattan, KS) enclosed in a housing made of 2.5 cm-thick high-density polyethylene (Figure 1B). Each Li foil detector had its own neutron pulse module and electronic components that were interfaced with a Raspberry Pi (Model Zero, Raspberry Pi Foundation) micro-computer to log observations hourly. The Li foil system was also equipped with a compact weather station (Model MetSENS300, Campbell Scientific) to record air temperature, air relative humidity, and barometric pressure at hourly intervals. Both systems were mounted on tripods and were powered using 20 W solar panels and 12V DC deep cycle marine batteries (Figure 1A). A battery problem on 5 June 2020 in the BF-3 detector resulted in the loss of 8 days of neutron counts and environmental observations. One of the three detectors of the Li foil system exhibited anomalous hourly neutron counts near the end of the experiment, possibly due to temperature or moisture effects on sensor electronics (Supplementary Figure 1). These outliers were removed before further data processing. For clarity, throughout the manuscript we use the term “Li foil system” to denote the device using all three individual Li foil modules and we reserve the term “Li foil module” to indicate an individual neutron module.
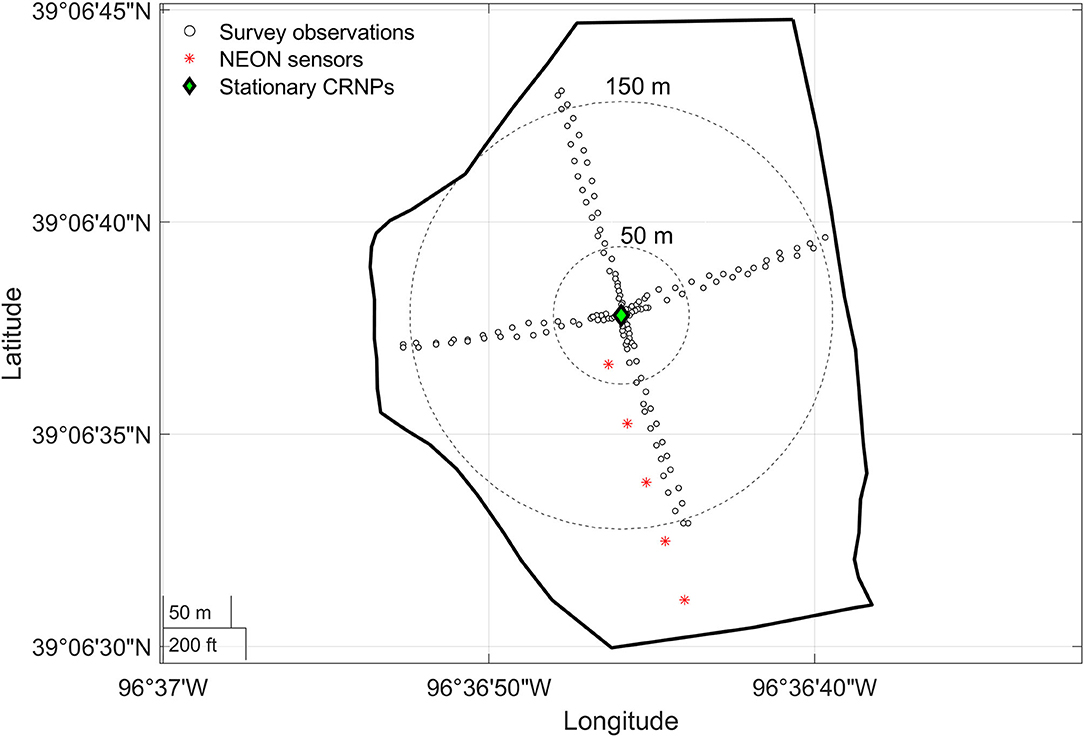
Figure 2. Map of the field located in the Konza Prairie Biological Station showing the location of the stationary cosmic-ray neutron probes (CRNPs) (for clarity, a single marker was used to denote both CRNPs located only 3.5 m apart), the five profile sensors of NEON KONA site, and the soil moisture observations (n = 180) collected using a hand-held soil water reflectometer (Hydrosense II, Campbell Scientific) during the field survey on 21 May 2020. Approximately, 56% of the observations were within a 50 m radius of the CRNPs.
Each individual Li foil module consists of multiple suspended 6Li metal foils (95% enrichment) between banks of anode wires in an aluminum enclosure with an effective area of 885 cm2. Each detector operates at a voltage of 3.3 V and draws about 55–60 mA of current, which results in a power requirement of ~180–200 mW per module (~600 mW for the entire system with three modules tested in this study without including the datalogger). The Li foil modules have an intrinsic thermal neutron detection efficiency (i.e., probability of detecting a thermal neutron) of 39.2% ± 1% (Nelson et al., 2012) and a gamma ray rejection ratio of 10−8 at 50 mR per hour of 137Cs (Montag et al., 2019). The Li foil technology is based on elemental enriched lithium-6 layers rolled into thin Li foils using the same process as Li-ion batteries. The device configuration consists of a four-foil design with two suspended foils and two wall foils (Montag et al., 2019). Each Li foil multi-wire proportional counter is calibrated with a known activity 252Cf source against a calibration standard Li foil counter. A pulse height spectrum is collected from the Li foil calibration standard held in a HDPE sleeve with a 252Cf source placed at a standard distance from the device to achieve >103 measured neutron counts under the full energy peak. Because lithium is an alkali metal that can react with moisture and produce heat, lithium hydroxide, and combustive hydrogen, handling and shipping restrictions typically follow similar regulations as common Li-ion batteries.
Neutron Corrections and Calibration
The observed neutron intensities for each CRNP were corrected using average hourly records of air temperature, relative humidity, and barometric pressure. The differences in weather observations between instruments mounted on each tripod was negligible, but to avoid entering a new source of error during the correction of the neutron intensities for both devices, hourly records of air temperature, relative humidity, and pressure from the different instruments were averaged. Neutron intensities were normalized to a reference pressure of 97.8 kPa using an exponential model (Zreda et al., 2012) and an atmospheric attenuation coefficient of 0.077 kPa−1 (e.g., Dong et al., 2014). The selected reference atmospheric pressure represents the 3 yr average pressure at the Ashland Bottoms station of the Kansas Mesonet (Patrignani et al., 2020), which is located 2.7 km north of the field. Neutron intensities were also corrected for atmospheric water vapor using a correction factor as a function of the absolute air humidity using a reference value of 0 g m−3 (Zreda et al., 2012; Rosolem et al., 2013). To account for variations in the incoming neutron intensity due to changes in solar activity, we used the relative incoming neutron flux for the Irkutsk neutron monitor (IRKT, Irkutsk, Russia, pressure and efficiency corrected), which we obtained from the Neutron Monitor Database (NMDB; http://www01.nmdb.eu/nest/). The IRKT neutron monitor is located at a comparable cutoff rigidity (R = 3.64 GV) and elevation (435 m a.s.l.) as our field. The incoming neutron intensity correction factor is simply the ratio of the measured neutron monitor intensity at any given time to a specified reference intensity. In this study we adopted the neutron counts on 10 April 2020 1700 h UTC (i.e., first hour of the experiment) as the reference intensity. The three normalizing factors were applied to the raw neutron intensities to obtain the final corrected counts (Zreda et al., 2012) following:
where N represents the corrected neutron counts, Nr is the uncorrected neutron counts as obtained from the datalogger, fp is the correction factor for barometric pressure, fw is the correction factor for atmospheric water vapor, and fi is the correction factor for incoming neutron flux based on the reference site with similar elevation and cutoff rigidity.
Because our detectors were deployed in cropland with actively growing vegetation, we also considered a correction factor to account for the hydrogen pool in the vegetation water content and the plant structural tissue. An approximate value of the wheat biomass was obtained from the NEON data portal for the KONA site (Supplementary Table 1). A total of 18 samples of dry above-ground biomass from herbaceous clippings collected on 15 June 2020 (a few days before harvest) in the same wheat field revealed an estimated maximum dry above-ground biomass of 1.14 (σ = 0.23) kg m−2. Using a large dataset from a network of CRNPs in the Rur catchment, Germany, Baatz et al. (2015) found that values of dry above-ground biomass ~1.0 kg m−2 represent an approximate reduction in the neutron counts of about 0.9%. Thus, we assumed that the impact of winter wheat above-ground biomass was negligible for the purposes of this study.
Conversion of Corrected Neutron Counts to Soil Moisture
Corrected neutron counts were calibrated against measured volumetric water content in the top 12 cm of the soil profile. The surface soil layer is subjected to frequent and abrupt changes in soil water storage caused by incident rainfall, drainage, and evapotranspiration losses. Thus, monitoring soil moisture in this layer presents a good test for comparing the sensitivity of CRNPs to changes in the soil moisture conditions. The lower limit of 12 cm was selected to match the rod length of the hand-held soil water reflectometer used for the intensive soil moisture surveys (see section Field Observations for Calibration). Corrected neutron counts for each device were converted into volumetric water content in the top 12 cm depth using the following calibration function (Desilets et al., 2010; Dong et al., 2014):
where N0was assumed to be a device-specific fitting parameter representing the hourly corrected neutron intensity over dry soil at the same field; wL is the lattice water content (0.033 g g−1), which was approximated as the water released at 1,000°C (i.e., loss on ignition) preceded by drying the soil sample at 105°C; the a0, a1, and a2 are fitting parameters with values of a0 = 0.0808, a1= 0.372, and a2 = 0.115 (Desilets et al., 2010); and ρb is the soil bulk density (1.33 g cm−3). The value of lattice water was obtained from a composite soil sample analyzed by Activation Laboratories Ltd. (Ontario, Canada).
The N0 parameter was obtained by fitting Equation (2) to pair-wise calibration points of corrected neutron intensities and field-average volumetric water content in the top 12 cm obtained from dedicated field-level intensive soil moisture surveys described below. Because field surveys covered a limited range in field-average soil moisture conditions, volumetric water content at five additional points in time were added from an existing array of five profile sensors that are part of the NEON KONA site (Figure 2, Supplementary Table 1). This array of permanent sensors was arranged along a transect spanning from ~30–200 m to the south of the main tower (NEON tower and CRNPs were about 10 m apart) and consists of calibrated capacitance-type sensors (TriSCAN, Sentek, Australia) providing measurements at multiple depths (Roberti et al., 2018). For the permanent array of sensors, the volumetric water content in the top 12 cm was estimated as the average observations of the sensors at 5 and 15 cm depth. This decision was made after testing different depth-weighted averages of volumetric water content obtained from the sensors at 5 and 15 cm from the array of NEON sensors and the intensive soil moisture surveys (Supplementary Figure 2).
To represent the field average soil moisture condition in the top 12 cm for each calibration point, we weighted the observations from each intensive survey and the observations from the array of permanent sensors using the horizontal distance weighting function proposed by Köhli et al. (2015) and extensively tested with field observations by multiple researchers (Heidbüchel et al., 2016; Schrön et al., 2017). The distance to CRNPs was computed based on the geographic coordinates of each field observation and a depth of 6 cm was assumed to represent the 0–12 cm range. Thus, the calibration of the stationary CRNPs consisted of a total of nine calibration points (4 from intensive surveys and 5 from NEON sensor array) spaced at approximately weekly intervals (except for the period with missing data for the BF-3 system between 5 June and 13 June 2020).
Field Observations for Calibration
A total of four field surveys were conducted by intensively (>120 observations) measuring the volumetric water content in the top 12 cm of the soil profile using a calibrated hand-held soil water reflectometer (Figure 1, Hydrosense II CD659 12 cm rods, Campbell Scientific, Logan, UT) (Table 1). The Hydrosense is equipped with a display that includes a Global Positioning System (GPS) receiver (±3 m accuracy) and an internal memory that stores the geolocation and raw sensor variables for each measurement. Volumetric water content was measured approximately every 10 meters along 150 m radial transects in each cardinal direction from the detectors (Figure 2). While less accurate than the thermo-gravimetric method, light-weight and portable soil moisture sensors facilitate intensive, non-destructive field sampling (e.g., Vather et al., 2019). For instance, field surveys using the portable sensor ranged between 123 and 180 observations per survey, a value that is about five to 10 times greater than typical CRNP calibration surveys using soil coring devices (Table 1) (e.g., Montzka et al., 2017; Dong and Ochsner, 2018). In our study, the portable sensor was calibrated by first taking field readings with the sensor and then collecting undisturbed soil samples in the same location with a soil core sampler 5 cm in diameter and 12 cm long (AMS samplers, American Falls, ID, USA). Volumetric water of each soil core was determined in the laboratory using the thermo-gravimetric method. A linear model relating the volumetric water content (θ) measured by soil sampling to the apparent dielectric permittivity (Ka) measured by the sensor (e.g., Ledieu et al., 1986) was fit to the calibration samples (Figure 3), resulting in:
We then determined the soil moisture for each field survey observation by converting the apparent dielectric permittivity into volumetric water content using Equation (3).
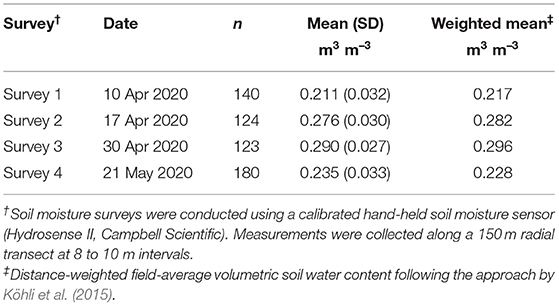
Table 1. Summary of field soil moisture surveys in the top 12 cm of the soil profile, including the number of observations (n), the mean, the standard deviation (SD), and the spatially-weighted mean.
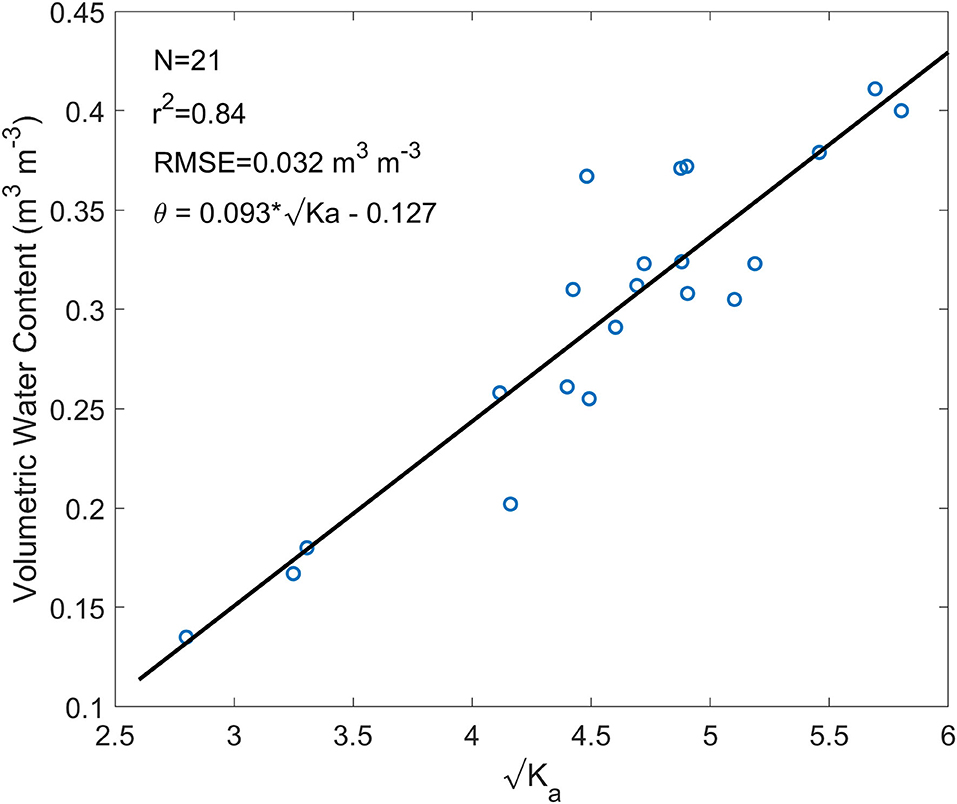
Figure 3. Site-specific calibration of volumetric water content for the 0–12 cm soil layer as measured by soil sampling vs. the square root of the apparent dielectric permittivity (Ka) as measured by the portable soil water reflectometer.
Statistics and Error Metrics
Time series of neutron intensity were de-noised using an 11 h Savitzky-Golay filter (polynomial degree 3, SG) (Savitzky and Golay, 1964), which has been shown to better preserve the timing of sharp changes in soil moisture caused by rainfall events than does a simple moving average filter (Franz et al., 2020). The volumetric water content from each CRNP was compared to the average value from the array of soil moisture sensors from the NEON network using the mean absolute difference (MAD) (Willmott and Matsuura, 2005). When comparing time series of volumetric water content, we use the term “difference” as opposed to “error” to highlight that both the CRNPs and the array of sensors from the NEON network likely contain measurement errors. In all cases, the error metrics were computed after applying the 11 h SG filter to the time series of corrected neutron counts. Volumetric water content was estimated from the filtered time series of corrected neutron counts.
Results and Discussion
The field test of a novel Li foil CRNP for soil moisture monitoring spanned a wide range of environmental conditions from 10 April to 18 June 2020 (~69 days). During this period, air temperature varied between −5 and 35°C, the relative humidity ranged from 20 to 100%, and the barometric pressure variations spanned about 70% of the typical range for the location (Supplementary Figure 3). The site also received 287 mm of rainfall over the study period spread across 25 days, with daily totals ranging from 0.5 to 60 mm. Overall, the observation period was adequate to test the performance of the new Li foil system against a commercially-available BF-3 system under typical spring and summer weather conditions for the central U.S.
Calibration
The apparent dielectric permittivity measured by the Hydrosense probe was linearly related to the volumetric water content with r2 = 0.84 and MAD = 0.032 m3 m−3 across the range from 0.13 to 0.41 m3 m−3 (Figure 3). The error of the calibration curve is similar to previous studies using hand-held sensors in the region (Cosh et al., 2005; Dong et al., 2014) and in an agricultural field in northern Germany (Rivera Villarreyes et al., 2011). Intensive soil moisture surveys using similar portable soil water reflectometers (e.g., Hydrosense II, 20 cm rod model) have been used to calibrate a roving CRNP in grassland and forest fields in South Africa (Vather et al., 2019). The study by Vather et al. (2019) did not include a site-specific sensor calibration because the fields were dominated by sandy soils, in which the manufacturer's calibration is recommended, but in our study, the presence of silty clay loam soils required a custom calibration equation. In general, the manufacturer's calibration tended to overestimate the volumetric water content in our field, particularly in moist soil conditions. For instance, using the manufacturer's calibration, a value of 0.429 m3 m−3 (Ka = 28.1) obtained from the transect conducted on 17 April 2020 changed to 0.365 m3 m−3 after applying site-specific calibration. Site-specific calibration of electromagnetic soil moisture sensors may be particularly beneficial in fine textured soils with moderate to high levels of clay content (>20% clay).
The resulting field-averaged volumetric water content in the top 12 cm from the Hydrosense surveys ranged from 0.211 to 0.290 m3 m−3 (Table 1). The within-survey standard deviation averaged ~0.031 m3 m−3 across all surveys, the standard error of the mean averaged ~0.0026 m3 m−3 (0.031/), and the low to moderate horizontal spatial variability was reflected by the coefficient of variation being <15% (Table 1). Contrary to our initial expectations, the distance-weighted field-average volumetric water content from the intensive soil moisture surveys were only marginally (<1%) different compared to a simple arithmetic average. Two potential reasons for this similarity are the relatively homogeneous soil moisture conditions across each field survey and the large number of observations in each survey that, to some extent, implicitly account for the radial distance weights due to the higher concentration of observations near the CRNPs (Figure 2).
The calibration of the stationary Li foil and BF-3 systems using the dataset of intensive (i.e., >120 observations) soil moisture surveys and data from the array of permanent in situ soil moisture sensors resulted in N0 = 3,767 cph for the Li foil system and N0 = 3,486 cph for the BF-3 system (Table 2, Figure 4). The two-way standard error of the mean for each calibration observation and the point cloud using the permanent array of soil moisture sensors reveal the variability implicit during the calibration process (Figure 4). A portion of that variability is likely due to the fact that the 12 cm maximum depth of the calibration data was less than the sensing depth of the CRNP, which may have reached 20-25 cm deep given the soil moisture conditions during this study (Supplementary Figure 5; Köhli et al., 2015). Under these circumstances, calibration observations spanning a range of soil moisture conditions may be necessary to provide a robust determination of the N0 parameter. Although the existing array of only five soil moisture sensors used here may be sub-optimal, previous studies have successfully calibrated stationary CRNPs using existing arrays of in situ soil moisture sensors at a comparable depth. For instance, Rivera Villarreyes et al. (2011) also used short periods of hourly volumetric water content data from an array of 16 soil moisture sensors (10 cm depth) distributed across an agricultural field in northern Germany to calibrate a stationary 3He-based detector.
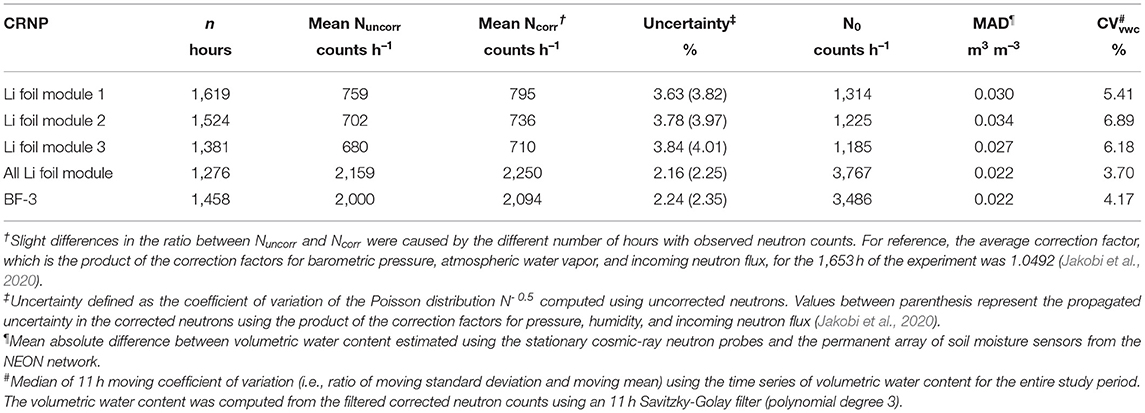
Table 2. Summary of mean uncorrected (Nuncorr) and corrected (Ncorr) neutron intensities, uncertainty, device-specific parameters (N0), mean absolute difference (MAD), and coefficient of variation of estimated volumetric water content (CVvwc) for the Li foil and BF-3 stationary systems.
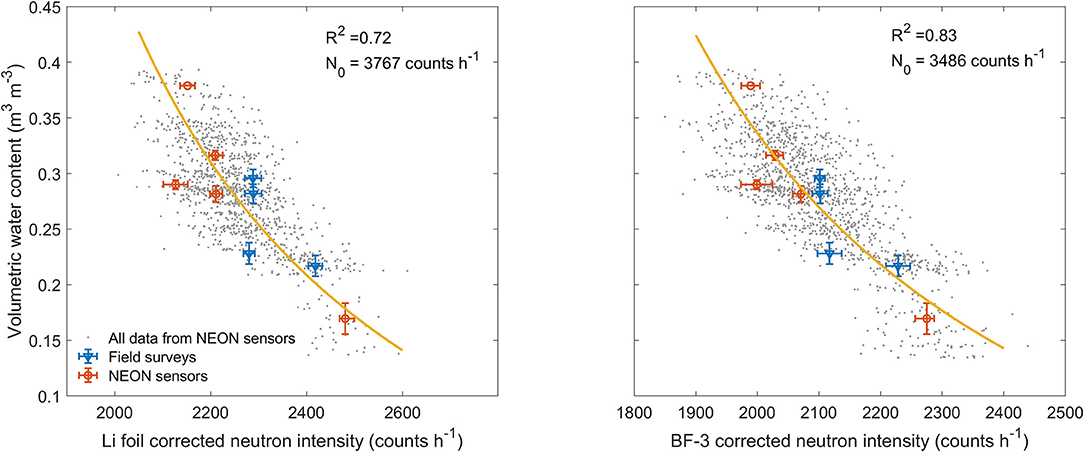
Figure 4. Calibration curve of the Li foil (left) and BF-3 (right) systems. The calibration was accomplished using the average volumetric water content in the 0–12 cm soil layer of four field surveys and five additional points of average volumetric water content obtained from a transect of five soil moisture sensors located across the NEON KONA site. The volumetric water content of the NEON sensors is the average of the sensors at 5 and 15 cm depth. All observations of volumetric water content from the NEON sensors were added in the background to show the variability of the data but were not included in the curve fitting exercise. Mean neutron counts for each calibration point are the arithmetic average of a 12 h period of corrected unfiltered neutron intensities. Error bars represent ±1 standard error of the mean.
Neutron Intensity
The normalized (N/N0) uncorrected neutron intensities for the Li foil and the BF-3 systems were highly correlated (r2 = 0.91, Figure 5), with virtually no discernible bias between the two devices (Mean difference = 0.001). The time series of normalized corrected neutron count rates for the new Li foil system and the BF-3 system were comparable, with both instrument neutron counts sharply decreasing with increasing soil moisture conditions caused by rainfall events and slowly increasing with drying of the soil profile between rainfall events (Figure 6). Normalized neutron count rate for the Li foil system ranged from 0.53 to 0.69 and for the BF-3 system ranged from 0.49 to 0.7 (Figure 6). The effective range of the Li foil system was slightly lower compared to that of the BF-3 system. For instance, during the 5 h precipitation event between 24 and 25 May 2020 that totaled 114 mm, the lowest ratio for the Li foil system was 0.54 and the lowest ratio for the BF-3 system was 0.52. The reason for this minor discrepancy is unclear, but we speculate that it could be attributed to calibration errors, a lower sensitivity of the Li foil system to soil with temporary ponded water, or microphonic errors. In the Li foil system microphonic errors are mitigated by packing the individual modules in vibration dampening foams and by specialized firmware that identifies microphonic noise and rejects the associated counts, but it is possible that our prototype and associated electronics need further fine-tuning to reduce abnormal counts. Considering the entire time series of corrected neutron counts, the Li foil system ranged between 1,994 and 2,610 counts per hour, while the BF-3 system ranged between 1,720 and 2,439 counts per hour. The Li foil system consisting of three individual Li foil modules yielded an average corrected neutron count rate of 2,250 counts per hour, which was about 7.5% higher than that of the shielded single-detector BF-3 system with an average of 2,094 counts per hour (Table 2). Analysis of the mean neutron counts for the individual Li foil detectors showed a difference of about 10% between detectors. While there could be a self-shielding effect between Li foil detectors, we speculate that this difference is mostly attributed to the calibration process with 252Cf.
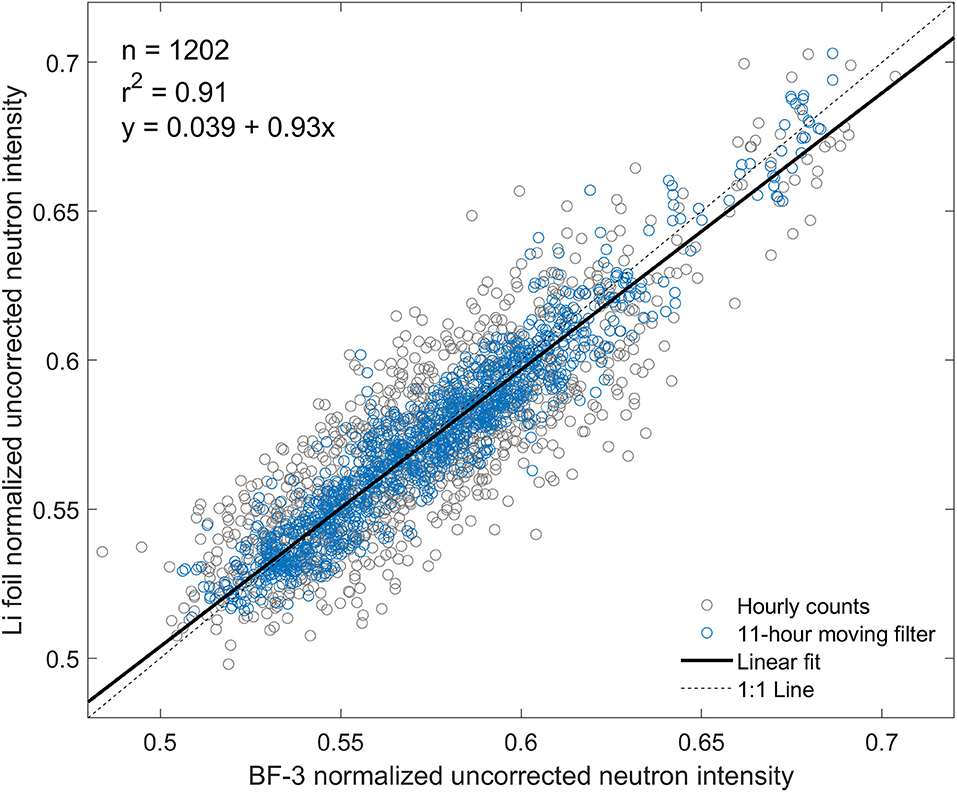
Figure 5. Correlation between the normalized (N/N0) uncorrected neutron counts of Li foil and BF-3 stationary cosmic-ray neutron systems from 10 April 2020 to 18 June 2020 in a cropland field located near Manhattan, KS, USA. Gray markers in the background represent hourly neutron intensity and the blue markers represent the filtered counts using an 11 h Savitzky-Golay filter (polynomial degree 3).
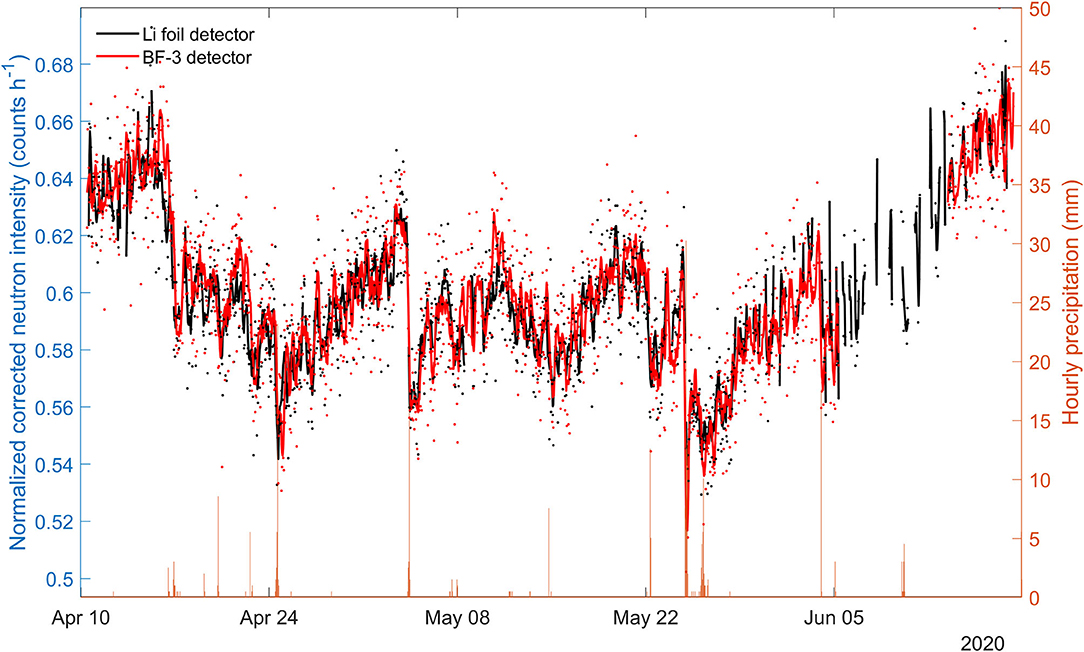
Figure 6. Temporal dynamics of normalized (N/N0) corrected cosmic-ray neutron counts from the Li foil and BF-3 systems from 10 Apr 2020 to 18 Jun 2020 in a cropped field located in the Konza Prairie Biological Station near Manhattan, KS, USA. The point-cloud represents hourly neutron intensity for each system and the lines represent the 11 h moving Savitzky-Golay filter (polynomial degree 3). Hourly precipitation totals are shown as vertical bars.
The comparable neutron count rates for the BF-3 system and the new Li foil system is relevant because neutron intensities follow Poisson statistics, meaning that measurement precision increases with neutron counts. The coefficient of variation for the Poisson distribution is defined by N−0.5 (Knoll, 2000; Zreda et al., 2012), therefore, the resulting uncertainty in the corrected neutron counts for the Li foil system was 2.25% and for BF-3 system was 2.35% (Table 2). The SG filter was effective to remove part of the high-frequency noise while preserving the sharp changes during rainfall events. However, close examination of the time series of corrected neutron counts revealed that the SG filter tended to preserve more of the high frequency noise between rainfall events compared to using a moving average filter with the same window length (see Supplementary Figure 4). A moving average filter, however, has the undesirable effect of delaying the timing of soil moisture peaks associated with rainfall events (Franz et al., 2020). There may be benefits to further development of improved filters for smoothing inherently noisy neutron count data.
Since the systems were deployed 3.5 m apart, minor discrepancies in the uncorrected neutron intensities could be attributed to soil moisture variations in the surrounding soil, the random nature of neutron scattering, instrument materials and electronics, and instrument form factor (Schrön et al., 2018). On a few occasions (e.g., 13 April 2020 0900 h), the Li foil system seemed to exhibit slightly larger neutron count variations compared to the BF-3 system. A potential cause for these oscillations could be temperature effects in the Li foil modules or supporting electronics. However, due to the sporadic occurrence of the spikes, we were unable to confirm the temperature effect on sensor electronics. Temperature effects have been reported during field testing of a prototype of a novel cosmic-ray neutron sensor based on scintillators called Finapp, where air temperature was also inversely correlated to neutron counts (Stevanato et al., 2019, 2020). In that case, the researchers behind the Finapp device resolved temperature-related issues by fine tuning the high voltage power supply (Stevanato et al., 2020). On the other hand, the BF-3 system is a well-established instrument that shows minimal temperature effects, and the internal walls of the enclosure are covered with insulated panels that reduce temperature fluctuations. The interior of the Li foil enclosure was not insulated and future models of Li foil-based systems may need to improve hardware components, enclosure insulation, fine tuning the power supply, or adopting firmware corrections to detect spurious neutron counts. The Li foil system used in this study was an advanced prototype, and we expect that improvements in the manufacturing and configuration of the neutron modules and associated electronics will be implemented based on this and related research studies.
Volumetric Water Content
Both CRNPs produced comparable soil moisture time series with a MAD = 0.017 m3 m−3 between the two systems (Figure 7). The estimated volumetric water content with the CRNPs clearly responded to the precipitation time series and closely followed the average soil moisture observed with the array of in situ sensors. Compared with the average of the in situ sensors, both the Li foil and BF-3 systems had a MAD = 0.022 m3 m−3. Occasionally, the volumetric water content estimated with the Li foil and BF-3 systems reached near-saturation conditions or even exceeded the estimated soil porosity of 49.8% after large rainfall events. For instance, after a rainfall event totaling nearly 80 mm on 25 May 2020, the estimated volumetric water content with the Li foil system reached a maximum value of 0.443 m3 m−3, but the BF-3 system estimated a value of 0.546 m3 m−3. This short time in which the BF-3 system resulted in a volumetric water content exceeding the soil porosity might be attributable to temporary local ponding in the vicinity (i.e., tripod area) of the detector or due to errors in the calibration of the BF-3 system. Although we did not make direct observations of soil hydraulic properties, silty soils under frequent tillage events (i.e., disking) in this region are prone to soil crusting and low aggregate stability, which can greatly reduce the rate of soil water infiltration and soil water redistribution (Stone and Schlegel, 2010).
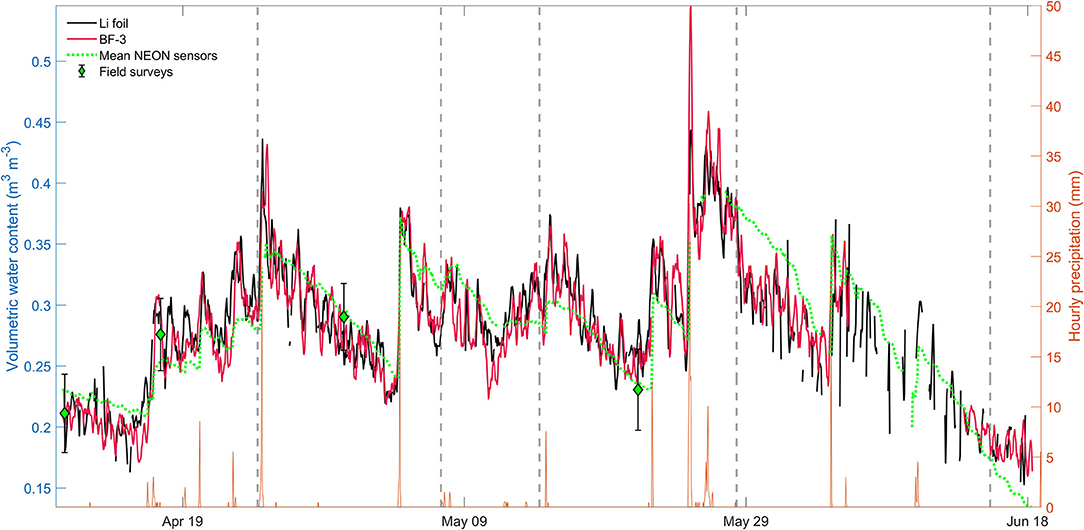
Figure 7. Estimated field-average volumetric water content using a Li foil cosmic ray neutron probe (CRNP), and a BF-3 CRNP, an array of five in situ sensors from the NEON network, and four field surveys in which water content was measured using a calibrated hand-held soil water reflectometer. The data from the field surveys were used for calibration of the CRNPs. Vertical dashed lines indicate the start of 12 h periods during which data from the array of NEON sensors were also included in the CRNP calibration to cover a wider range of volumetric water content. The lines for the CRNPs represent the 11 h moving Savitzky-Golay filter (polynomial degree 3).
The close agreement between the estimated soil moisture from the CRNPs and the soil moisture from the array of in situ sensors and the intensive field surveys provides support to our assumption that land covers with ~1 kg m2 of above-ground dry biomass have a negligible impact on the neutron counts. Errors in soil volumetric water content due to vegetation effects have often been associated with CRNP measurements in vegetation types having greater above-ground biomass, such as maize and forests (Hornbuckle et al., 2012; Franz et al., 2013; Baatz et al., 2015). However, localized discrepancies between the time series of volumetric water content suggest that either vegetation alone or the combined effect of vegetation and rainfall interception by the canopy could be introducing some errors in the final volumetric water content that would affect both CRNPs equally.
Because cost is an important factor affecting the adoption of cosmic-ray sensing technology, we expanded our analysis to determine the variability of count rates and estimated volumetric water contents using a decreasing number of Li foil modules. In other words, we compared combinations of one or two modules against the Li foil system with three modules and the BF-3 system. Using the continuous time series of volumetric water content from the array of in situ sensors as a benchmark, a single Li foil module with an average of 747 corrected neutron counts per hour resulted in a MAD = 0.030 m3 m−3 and average 11 h moving CV = 6.2% (Table 2). As expected, including data from a second Li foil module doubled the average corrected neutron counts, which resulted in a 14% improvement in the precision (MAD = 0.024 m3 m−3, mean of the three possible combinations of two Li foil detectors) compared to a single module, and the variability of the estimated volumetric water content was reduced with an average moving CV = 4.6%. The inclusion of a third Li foil module further reduced the uncertainty of corrected neutron counts (MAD = 0.022 m3 m−3) and the variability in the estimated volumetric water content (CV = 3.7%), although these reductions were less pronounced than those which occurred when stepping from one to two modules. Based on our findings, Li foil monitoring systems with two modules reaching an average of about 1,500 corrected neutron counts per hour may provide a good balance between cost and performance, and may provide adequate accuracy (MAD ~0.024 m3 m−3) for many agricultural and hydrological applications. The precision would likely be greater in higher altitude regions or regions characterized by drier soil moisture regimes, but in lower altitude regions or regions with wetter soils, the count rate provided by two Li foil detectors may be too low for some applications.
At present time, the Li foil system tested in this study costs about US$4.4 per average uncorrected hourly count (Supplementary Table 2). This cost includes the three thermal detectors, sensor electronics, and moderating HDPE enclosure. In comparison, the BF-3 system tested in this study costs about US$8.4 per average uncorrected hourly count and a system based on 3He, with an average of 750 uncorrected counts per hour, costs about US$26.5 per average uncorrected hourly count. All estimates include the cost of the thermal detectors, pulse counters, sensor electronics and datalogger, and moderating HDPE enclosure. These costs are based on prior purchases by the authors and provide only a gross approximation because important differences exist between these sensing systems regarding build quality, data logging systems, and telemetry options. Compared to a similar 3He system, the Li foil technology tested in this study could reduce costs by about 80%.
One limitation of our study is the fact that the sensing depth of the CRNPs likely extended below the depth of the in situ measurements during the field calibration (i.e., 12 cm), and this discrepancy in sensing depths likely influenced the volumetric water content estimated with the CRNPs. To assess the magnitude of the discrepancy in sensing depth, the effective sensor penetration depth (D86, cm), defined as the depth within which 86% of neutrons probed the soil (Köhli et al., 2015), was computed as a function of the scaled radial distance from the sensor (r*, meters), the soil water equivalent (θT = θ + wL), and the soil bulk density (ρb) following the approach described in Appendix A of Schrön et al. (2017):
where p0 = 8.321, p1 = 0.14249, p2 = 0.96655, p3 = 0.01, p4 = 20, and p5 = 0.0429. Across the study period, the resulting penetration depth for both sensors ranged from 14 to 25 cm at a radial distance of 10 m from the sensors and from 11 to 18 cm at a radial distance of 150 m from the sensors (Supplementary Figure 5). The median sensing depth was 18 cm at 10 m from the sensors and 13 cm at 150 m from the sensors, depths that are only 6 and 1 cm deeper than the sampling depth used during the field calibration of the CRNPs, suggesting that the calibration depth used in this study likely captured the main field-level soil moisture dynamics sensed by the CRNPs. Our results are aligned with previous studies, in which CRNPs have been effectively calibrated using data from depths as shallow as 0–5 cm (Dong et al., 2014).
Conclusions
We presented field data for a novel cosmic-ray neutron proportional counter based on 6Li-enriched foil for soil moisture monitoring. The Li foil monitoring system was capable of providing comparable uncorrected neutron counts and volumetric water content estimates to a neutron detector based on boron trifluoride, which has been widely used for monitoring soil moisture in hydrological, ecological, and agricultural applications. The data indicate that a Li foil detector capable of averaging ~1,500 neutron counts per hour could produce soil water content estimates with acceptable accuracy for many agricultural and hydrological applications. The results of this study suggest some promising avenues for further research to enhance the stability and design of Li foil based CRNPs, which show good potential to increase the affordability and facilitate wider use of cosmic-ray neutron sensing by researchers and end users alike, enabling them to better monitor and manage soil water.
Data Availability Statement
The original contributions presented in the study are included in the article/Supplementary Material, further inquiries can be directed to the corresponding author/s.
Author Contributions
AP contributed to field data collection, data processing, numerical and statistical analyses, and article writing. TO contributed with manuscript writing and data analysis. BM contributed with field data collection and technical support for the new sensing technology. SB contributed with technical support for the new soil moisture sensing technology. All authors contributed to manuscript revision, read, and approved the submitted version.
Funding
This study was based in part upon work supported by the National Science Foundation through the National Ecological Observatory Network program, which sponsored by the National Science Foundation and operated under cooperative agreement by Battelle Memorial Institute. This project was partly supported by the Agriculture and Food Research Initiative Competitive Grant no. 2019-68012-29888 from the USDA National Institute of Food and Agriculture, by the Kansas Agricultural Experiment Station (contribution 21-321-J), by the National Science Foundation EPSCoR First Awards program (award no. 1001302), by Hatch Project OKL03123, and by the Division of Agricultural Sciences and Natural Resources at Oklahoma State University.
Conflict of Interest
This research study was conducted in collaboration with Radiation Detection Technologies. SB and BM are part of Radiation Detection Technologies.
The remaining authors declare that the research was conducted in the absence of any commercial or financial relationships that could be construed as a potential conflict of interest.
Acknowledgments
The authors would like to thank Dustan Ridder and John Flavin for working with us during farming operations and field activities at the study site. We also acknowledge the NMDB database (www.nmdb.eu) founded under the European Union's FP7 programme (contract no. 213007), and the PIs of individual neutron monitors at: Irkustk (Institute of Solar-Terrestrial Physics, ISZF, Russian Academy of Sciences, Irkutsk, Russia).
Supplementary Material
The Supplementary Material for this article can be found online at: https://www.frontiersin.org/articles/10.3389/frwa.2021.673185/full#supplementary-material
References
Baatz, R., Bogena, H. R., Hendricks Franssen, H. J., Huisman, J. A., Montzka, C., and Vereecken, H. (2015). An empirical vegetation correction for soil water content quantification using cosmic ray probes. Water Resour. Res. 51, 2030–2046. doi: 10.1002/2014WR016443
Bogena, H. R., Huisman, J. A., Baatz, R., Hendricks Franssen, H. J., and Vereecken, H. (2013). Accuracy of the cosmic-ray soil water content probe in humid forest ecosystems: the worst case scenario. Water Resour. Res. 49, 5778–5791. doi: 10.1002/wrcr.20463
Bogena, H. R., Huisman, J. A., Güntner, A., Hübner, C., Kusche, J., Jonard, F., et al. (2015). Emerging methods for noninvasive sensing of soil moisture dynamics from field to catchment scale: a review. Wiley Interdiscip. Rev. Water 2, 635–647. doi: 10.1002/wat2.1097
Chrisman, B., and Zreda, M. (2013). Quantifying mesoscale soil moisture with the cosmic-ray rover. Hydrol. Earth Syst. Sci. 17, 5097–5108. doi: 10.5194/hess-17-5097-2013
Cosh, M. H., Jackson, T. J., Bindlish, R., Famiglietti, J. S., and Ryu, D. (2005). Calibration of an impedance probe for estimation of surface soil water content over large regions. J. Hydrol. 311, 49–58. doi: 10.1016/j.jhydrol.2005.01.003
Desilets, D., Zreda, M., and Ferré, T. P. A. (2010). Nature's neutron probe: land surface hydrology at an elusive scale with cosmic rays. Water Resour. Res. 46, 1–7. doi: 10.1029/2009WR008726
Dong, J., and Ochsner, T. E. (2018). Soil texture often exerts a stronger influence than precipitation on mesoscale soil moisture patterns. Water Resour. Res. 54, 2199–2211. doi: 10.1002/2017WR021692
Dong, J., Ochsner, T. E., Zreda, M., Cosh, M. H., and Zou, C. B. (2014). Calibration and validation of the COSMOS rover for surface soil moisture measurement. Vadose Zone J. 13, 1–8. doi: 10.2136/vzj2013.08.0148
Evans, J. G., Ward, H. C., Blake, J. R., Hewitt, E. J., Morrison, R., Fry, M., et al. (2016). Soil water content in southern England derived from a cosmic-ray soil moisture observing system – COSMOS-UK. Hydrol. Process. 30, 4987–4999. doi: 10.1002/hyp.10929
Fersch, B., Francke, T., Heistermann, M., Schrön, M., Döpper, V., Jakobi, J., et al. (2020). A dense network of cosmic-ray neutron sensors for soil moisture observation in a highly instrumented pre-Alpine headwater catchment in Germany. Earth Syst. Sci. Data 12, 2289–2309. doi: 10.5194/essd-12-2289-2020
Franz, T. E., Wahbi, A., Zhang, J., Vreugdenhil, M., Heng, L., Dercon, G., et al. (2020). Practical data products from cosmic-ray neutron sensing for hydrological applications. Front. Water 2:9. doi: 10.3389/frwa.2020.00009
Franz, T. E., Wang, T., Avery, W., Finkenbiner, C., and Brocca, L. (2015). Combined analysis of soil moisture measurements from roving and fixed cosmic ray neutron probes for multiscale real-time monitoring. Geophys. Res. Lett. 42, 3389–3396. doi: 10.1002/2015GL063963
Franz, T. E., Zreda, M., Rosolem, R., Hornbuckle, B. K., Irvin, S. L., Adams, H., et al. (2013). Ecosystem-scale measurements of biomass water using cosmic ray neutrons. Geophys. Res. Lett. 40, 3929–3933. doi: 10.1002/grl.50791
Gavlak, R., Horneck, D., Miller, R. O., and Kotuby-Amacher, J. (2003). Soil, Plant and Water Reference Methods for the Western Region. WCC-103 Publication, Fort Collins, CO.
Hawdon, A., McJannet, D., and Wallace, J. (2014). Calibration and correction procedures for cosmic-ray neutron soil moisture probes located across Australia. Water Resour. Res. 50, 5029–5043. doi: 10.1002/2013WR015138
Heidbüchel, I., Guntner, A., and Blume, T. (2016). Use of cosmic-ray neutron sensors for soil moisture monitoring in forests. Hydrol. Earth Syst. Sci. 20, 1269–1288. doi: 10.5194/hess-20-1269-2016
Hornbuckle, B., Irvin, S., Franz, T., Rosolem, R., and Zweck, C. (2012). “The potential of the COSMOS network to be a source of new soil moisture information for SMOS and SMAP,” in International Geoscience and Remote Sensing Symposium (IGARSS) (IEEE), 1243–1246.
Jakobi, J., Huisman, J. A., Schrön, M., Fiedler, J., Brogi, C., Vereecken, H., et al. (2020). Error estimation for soil moisture measurements with cosmic ray neutron sensing and implications for rover surveys. Front. Water 2:10. doi: 10.3389/frwa.2020.00010
Knoll, G. F. (2000). Radiation Detection and Measurement, 3rd Edn. Book. General Properties of Radiation Detectors (New York, NY; Chichester; Weinheim; Brisbane; Singapore: John Wiley & Sons, Inc), 95–103.
Köhli, M., Schrön, M., and Schmidt, U. (2018). Response functions for detectors in cosmic ray neutron sensing. Nucl. Instruments Methods Phys. Res. Sect. A Acceler. Spectr. Detect. Assoc. Equip. 902, 184–189. doi: 10.1016/j.nima.2018.06.052
Köhli, M., Schrön, M., Zreda, M., Schmidt, U., Dietrich, P., and Zacharias, S. (2015). Footprint characteristics revised for field-scale soil moisture monitoring with cosmic-ray neutrons. Water Resour. Res. 51, 5772–5790. doi: 10.1002/2015WR017169
Ledieu, J., De Ridder, P., De Clerck, P., and Dautrebande, S. (1986). A method of measuring soil moisture by time-domain reflectometry. J. Hydrol. 88, 319–328. doi: 10.1016/0022-1694(86)90097-1
Lv, L., Franz, T. E., Robinson, D. A., and Jones, S. B. (2014). Measured and modeled soil moisture compared with cosmic-ray neutron probe estimates in a mixed forest. Vadose Zone J. 13, 1–13. doi: 10.2136/vzj2014.06.0077
Montag, B. W., Bellinger, S. L., Edwards, N. S., Lage, J., Nelson, K. A., Henson, L. C., et al. (2019). Recent progress in the commercialization of the Li Foil multi-wire proportional counter neutron detectors. Radiat. Phys. Chem. 155, 158–163. doi: 10.1016/j.radphyschem.2018.08.003
Montzka, C., Bogena, H. R., Zreda, M., Monerris, A., Morrison, R., Muddu, S., et al. (2017). Validation of spaceborne and modelled surface soil moisture products with Cosmic-Ray Neutron Probes. Remote Sens. 9:103. doi: 10.3390/rs9020103
Nelson, K. A., Bellinger, S. L., Montag, B. W., Neihart, J. L., Riedel, T. A., Schmidt, A. J., et al. (2012). Investigation of a lithium foil multi-wire proportional counter for potential 3He replacement. Nucl. Instruments Methods Phys. Res. Sect. A Acceler. Spectr. Detect. Assoc. Equip. 669, 79–84. doi: 10.1016/j.nima.2011.12.003
Nelson, K. A., Edwards, N. S., Kusner, M. R., Mayhugh, M. R., Montag, B. W., Schmidt, A. J., et al. (2015). A modular large-area lithium foil multi-wire proportional counter neutron detector. Radiat. Phys. Chem. 116, 165–169. doi: 10.1016/j.radphyschem.2015.03.044
Patrignani, A., Knapp, M., Redmond, C., and Santos, E. (2020). Technical overview of the Kansas Mesonet. J. Atmos. Oceanic Technol. 37, 2167–2183. doi: 10.1175/JTECH-D-19-0214.1
Rivera Villarreyes, C. A., Baroni, G., and Oswald, S. E. (2011). Integral quantification of seasonal soil moisture changes in farmland by cosmic-ray neutrons. Hydrol. Earth Syst. Sci. Discuss. 15, 3843–3859. doi: 10.5194/hess-15-3843-2011
Roberti, J. A., Ayres, E., Loescher, H. W., Tang, J., Starr, G., Durden, D. J., et al. (2018). A robust calibration method for continental-scale soil water content measurements. Vadose Zone J. 17, 1–19. doi: 10.2136/vzj2017.10.0177
Rosolem, R., Shuttleworth, W. J., Zreda, M., Franz, T. E., Zeng, X., and Kurc, S. A. (2013). The effect of atmospheric water vapor on neutron count in the cosmic-ray soil moisture observing system. J. Hydrometeorol. 14, 1659–1671. doi: 10.1175/JHM-D-12-0120.1
Savitzky, A., and Golay, M. J. E. (1964). Smoothing and differentiation of data by simplified least squares procedures. Anal. Chem. 36, 1627–1639. doi: 10.1021/ac60214a047
Schrön, M., Köhli, M., Scheiffele, L., Iwema, J., Bogena, H. R., Lv, L., et al. (2017). Improving calibration and validation of cosmic-ray neutron sensors in the light of spatial sensitivity. Hydrol. Earth Syst. Sci. 21, 5009–5030. doi: 10.5194/hess-21-5009-2017
Schrön, M., Zacharias, S., Womack, G., Köhli, M., Desilets, D., Oswald, S. E., et al. (2018). Intercomparison of cosmic-ray neutron sensors and water balance monitoring in an urban environment. Geosci. Instrument. Methods Data Syst. 7, 83–99. doi: 10.5194/gi-7-83-2018
Shea, D. A., and Morgan, D. (2011). “The helium-3 shortage: Supply, demand, and options for congress,” in Helium-3: Critical Uses and Supply Challenge, 1–27. Available online at: https://fas.org/sgp/crs/misc/R41419.pdf
Shuttleworth, J., Rosolem, R., Zreda, M., and Franz, T. (2013). The COsmic-ray Soil Moisture Interaction Code (COSMIC) for use in data assimilation. Hydrol. Earth Syst. Sci. 17, 3205–3217. doi: 10.5194/hess-17-3205-2013
Stevanato, L., Baroni, G., Cohen, Y., Lino, F. C., Gatto, S., Lunardon, M., et al. (2019). A novel cosmic-ray neutron sensor for soil moisture estimation over large areas. Agriculture 9:202. doi: 10.3390/agriculture9090202
Stevanato, L., Polo, M., Lunardon, M., Marinello, F., Moretto, S., and Baroni, G. (2020). “Towards the optimization of a scintillator-based neutron detector for large non-invasive soil moisture estimation,” in 2020 IEEE International Workshop on Metrology for Agriculture and Forestry, MetroAgriFor 2020 – Proceedings (Trento: IEEE), 196–200. Available online at: https://ieeexplore.ieee.org/document/9277582
Stone, L. R., and Schlegel, A. J. (2010). Tillage and crop rotation phase effects on soil physical properties in the west-central great plains. Agron. J. 102, 483–491. doi: 10.2134/agronj2009.0123
Vather, T., Everson, C., and Franz, T. E. (2019). Calibration and validation of the cosmic ray neutron rover for soil water mapping within two South African land classes. Hydrology 6:65. doi: 10.3390/hydrology6030065
Vather, T., Everson, C. S., and Franz, T. E. (2020). The applicability of the cosmic ray neutron sensor to simultaneously monitor soil water content and biomass in an acacia mearnsii forest. Hydrology 7:48. doi: 10.3390/hydrology7030048
Weimar, J., Köhli, M., Budach, C., and Schmidt, U. (2020). Large-scale boron-lined neutron detection systems as a 3He alternative for cosmic ray neutron sensing. Front. Water 2:16. doi: 10.3389/frwa.2020.00016
Willmott, C. J., and Matsuura, K. (2005). Advantages of the mean absolute error (MAE) over the root mean square error (RMSE) in assessing average model performance. Clim. Res. 30, 79–82. doi: 10.3354/cr030079
Zhu, Z., Tan, L., Gao, S., and Jiao, Q. (2015). Observation on soil moisture of irrigation cropland by cosmic-ray probe. IEEE Geosci. Remote Sens. Lett. 12, 472–476. doi: 10.1109/LGRS.2014.2346784
Zreda, M., Desilets, D., Ferré, T. P. A., and Scott, R. L. (2008). Measuring soil moisture content non-invasively at intermediate spatial scale using cosmic-ray neutrons. Geophys. Res. Lett. 35, 1–5. doi: 10.1029/2008GL035655
Keywords: soil moisture, large-scale monitoring system, cosmic-ray neutron probe, vadose zone, lithium foil, in situ soil moisture, proximal sensing
Citation: Patrignani A, Ochsner TE, Montag B and Bellinger S (2021) A Novel Lithium Foil Cosmic-Ray Neutron Detector for Measuring Field-Scale Soil Moisture. Front. Water 3:673185. doi: 10.3389/frwa.2021.673185
Received: 27 February 2021; Accepted: 07 June 2021;
Published: 07 July 2021.
Edited by:
Heye Reemt Bogena, Julich-Forschungszentrum, GermanyReviewed by:
Martin Schrön, Helmholtz Centre for Environmental Research (UFZ), GermanyMarkus Köhli, Heidelberg University, Germany
Marek Zreda, University of Arizona, United States
Copyright © 2021 Patrignani, Ochsner, Montag and Bellinger. This is an open-access article distributed under the terms of the Creative Commons Attribution License (CC BY). The use, distribution or reproduction in other forums is permitted, provided the original author(s) and the copyright owner(s) are credited and that the original publication in this journal is cited, in accordance with accepted academic practice. No use, distribution or reproduction is permitted which does not comply with these terms.
*Correspondence: Andres Patrignani, YW5kcmVzcGF0cmlnbmFuaSYjeDAwMDQwO2tzdS5lZHU=