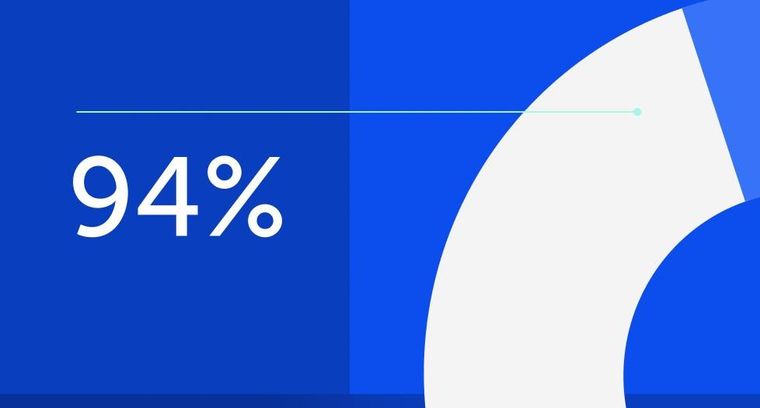
94% of researchers rate our articles as excellent or good
Learn more about the work of our research integrity team to safeguard the quality of each article we publish.
Find out more
POLICY AND PRACTICE REVIEWS article
Front. Water, 12 July 2021
Sec. Water and Built Environment
Volume 3 - 2021 | https://doi.org/10.3389/frwa.2021.671059
This article is part of the Research TopicResiliency of Urban Systems to Water-Related DisastersView all 5 articles
Many publications include references to reliability, risk and resilience, specifically within the context of climate change and rapid urbanization. However, there is a considerable gap between theory and actual implementation by drainage professionals. As such, most drainage professionals will not have an appreciation of a drainage system's response to events in excess of its original design event. This gap is compounded by the desire toward evaluating components such as “critical infrastructure” for events significantly more severe than ever contemplated. This paper, reflecting the combined wisdom and thoughts of various drainage professionals across Canada involved with the creation of the Canadian drainage standards (CSA W204 and W210), provides a treatise of risk and resilience based on the application of the dual drainage principle. It provides a discussion of key factors including climate change; densification; shape, intensity, duration and spatial extent of storm events, as a function of the normalized capacity or drain down/emptying time of the various components of the drainage system. Commentaries are offered, highlighting the role of appropriate setbacks and freeboard, and focusing on those aspects that have historically been ignored. Avenues to increase system resilience are presented including an evolution in passive and active flow controls, the potential beneficial role of natural systems and low impact development practices as a function of system sensitivity, discussing how options may vary across Canada.
Many publications including the recently released Canadian Standard W204 “Flood Resilient Design of New Residential Communities” provide discussion and references to reliability, risk and resilience, specifically within a climate change context. However, the terms flood hazard, vulnerability, and risk can be confusing; their interrelationship is often not well-understood and sometimes they are used interchangeably (Sayers et al., 2013; Florin and Linkov, 2016). As a result, without an adequate basic understanding of the concepts involved, there is a considerable gap between theory and actual implementation by drainage professionals. In practice, most drainage professionals will not have an appreciation of a drainage system's response to storm events in excess of its original design event. This gap is compounded by the desire toward evaluating components such as “critical infrastructure” for events significantly more severe than ever contemplated. In addition, there is often a tendency to simply mimic approaches such as the implementation of Low Impact Development (LID) measures successful in other jurisdictions without having an appreciation whether they are indeed appropriate for the local conditions.
Flood hazard refers to the outcome of a storm event or rainfall on a system or surface usually depicted as flow depth or water level, and velocity on a geographical extent, according to the upcoming Canadian Standard W210 “Prioritization of Flood Risk in Existing Communities” (CSA Group, 2021). CSA W204 describes in an annex the concepts of risk and resilience and how risk of failure is generally understood to be a function of failure probability (likelihood) and failure consequences. Since often a single event can involve several types of failure (e.g., flooding of basements, bridge collapse due to excessive flows) more specific definitions of risk often take into account elements of hazard (what could happen to trigger damage), exposure (what is at stake), vulnerability (the level of sensitivity to a hazard), and resulting consequences (the impact or damage caused by the hazard) (National Research Council, 2012).
Vulnerability is the degree to which a system is susceptible to, or unable to cope with, adverse effects of an event, including climate variability and extremes. In the context of climate, an event could be climate variability, climate extreme, etc., and vulnerability would be a function of the character, magnitude, and rate of climate variation to which a system is exposed, its sensitivity, and its adaptive capacity [adapted from Public Infrastructure Engineering Vulnerability Committee (PIEVC) and CSA W204].
Resilience is defined as the ability of a system, community or society exposed to hazards to resist, absorb, accommodate to and recover from the effects of a hazard in a timely and efficient manner, including through the preservation and restoration of its essential basic structures and functions (Public Infrastructure Engineering Vulnerability Committee (PIEVC), 2020). Risk management includes the suite of engineering and social processes engaged in the design, implementation, and evaluation of strategies to improve understanding, foster disaster risk reduction, and promote improvements in preparedness, response, and recovery efforts (Field et al., 2012). Although it appears clearly that a shift from a reliability-based design approach to a risk-based approach should be considered to provide a more resilient system, especially to explicitly account for climate change impacts, it is recognized that specific technical knowledge and concepts should be more precisely defined and communicated to modify the actual practice (Meyer et al., 2014).
This paper will first present the basic principles of resiliency (section Principles of Resiliency in Urban Stormwater Management) and uncertainties (section Uncertainties in Urban Stormwater Management) in urban stormwater management based on the dual drainage concept, and then propose strategies such as LID to increase resiliency in the face of densification and climate change (section Stormwater Management Strategies to Increase Resiliency). It demonstrates how to apply this knowledge with the help of a case study (section Comparison of Options to Improve Resilience across Canada). Discussions follow on strategies for both new subdivision development and within established communities and how they may vary across Canada, and also address environmental and other resiliency considerations, as well as future research needs (sections Proposed Implementation of Strategies to Increase Resiliency and Concluding Comments and Research Needs). This study will be useful to both drainage practitioners and academic communities.
Considerable concern has been expressed over the last number of years about damages resulting from extreme weather events with flood damages generally having been identified as one of the main consequences. Increasingly, the impacts are known to extend beyond immediate loss of life or physical damages. Long-term impacts include long-term mental impacts and the risk of mortgage defaults given that the actual costs to property owners may be substantially higher than what is covered by insurance. The latter may subsequently impact property values when a sufficiently large number of properties has been impacted.
While alleged “freak” events hitting our communities have been reported by the media, most attention has typically been paid to what can be described as “riverine flooding” rather than “pluvial flooding,” which is the realm of stormwater management practice. Riverine flooding is usually associated with the consequences of high amounts of rainfall and melting snow upstream of our urban communities. Pluvial flooding, on the other hand, deals with the consequences of high amounts of rainfall falling on our urban communities.
Climate change is often portrayed in the media as the main culprit for the flooding events that have occurred over the last few decades. Although temperatures across Canada have increased over the last couple of decades (Bush and Lemmen, 2019), due to the probabilistic nature of precipitation and paucity of our monitoring networks it has so far proven difficult to definitely validate increases in extreme precipitation across Canada. In fact, “extreme precipitation is projected to increase in the future, although the observational record has not yet shown evidence of consistent changes in short-duration precipitation extremes across the country” (Bush and Lemmen, 2019). “The lack of a detectable change in extreme precipitation in Canada, however, is not necessarily evidence of a lack of change—the expected change in response to warming may be small when compared with natural internal variability” (CSA Group, 2019a,b). Regardless, the pluvial flooding impacts seen to date may have been more reflective of the tremendous growth of and densification within our communities, superimposed on the consequences of changes in the use of our buildings (e.g., basement development) as well as past land use, development and stormwater management practice. For example, the June 2020 hail storm event in Calgary, Alberta, Canada that resulted in over $1B in hail damages saw relatively little flood damage in the more recently constructed communities while surrounding older communities incurred considerable flood damages. Regardless, climate science anticipates that precipitation increases will become increasingly noticeable in the coming decades with the impacts exacerbating the consequences of our land use and development decisions.
In the United Kingdom, the Construction Industry Research and Information Association (CIRIA), following a trend in the international scientific community, distinguished four realms with respect to the impacts of heavy rainfall. It suggests that with the introduction of “exceedance measures the response is reduced initially and the recovery threshold is deferred to larger events” (CIRIA, 2014). The “recovery threshold” represents the point where recovery is not feasible anymore leading to a regime shift.
The CIRIA guidance does not provide the same level of detail in distinguishing between the various flooding mechanisms that may occur as the upcoming CSA Standard W210 which includes (a) riverine flooding; (b) small stream or creek flooding; (c) sanitary sewer flooding; (d) storm sewer flooding; (e) overland flow flooding; and (f) groundwater flooding. Combined sewer systems which are prevalent in the older parts of many cities may be subject to a combination of (c) and (d). “Regime shift” phenomena, although highly undesirable within our urban communities, may occur for “riverine flooding” or “small stream or creek flooding” but will not happen for the other flooding mechanisms. The “recovery threshold” should therefore be interpreted as the point where wholesale damage and loss of life may occur which could result in long-term impacts on our communities. Overall, management approaches and solutions should be specific to the type of flooding that may occur. Even though not explicitly identified as such in the UK guidance, “designing for exceedance” seems to emulate the “Dual Drainage System” approach that was first introduced in Canada in the late 1970s (Wisner and Kassem, 1980; Wisner et al., 1981). These concepts themselves date back further, see for instance the drainage guidelines issued by the Urban Drainage and Flood Control District in 1969 (Urban Drainage and Flood Control District, 2016). The dual drainage system comprises two components: (1) the storm sewer conduits which convey the runoff for the minor storm events up to a 1:2 to 1:5 year return frequency, and (2) the overland drainage system which conveys the runoff from major storm events, usually up to 1:100 year return frequency, in excess of the capacity of the storm sewer system. The overland drainage system component of the “dual drainage system” always exists in our urban communities, whether it was designed for that purpose or not. In older communities, only the conduits in the storm sewer system were usually considered, while the overland drainage system was typically ignored in design practice. This has contributed to the flooding experienced in these older communities, compounded by climate change and the densification that have occurred.
Given its success in protecting our communities from storm sewer and overland flow flooding, a dual drainage approach is one of the central tenets of the recently released CSA Standard W204 (CSA Group, 2018). However, even in Canada, design professionals have little appreciation of how resilience to extreme rainfall can be provided which is specifically acute in view of densification and the anticipated climate change which may lower the level of service offered by our existing drainage infrastructure.
With reference to PVIEC's definition of resiliency, Figure 1 provides a conceptual representation of the flood control performance of a (dual) drainage system as a function of the severity of a storm event, which, in turn, is a function of both the intensity and duration of the “design” storm event in question.
The “level of service” can be defined as that point where the flood control performance of the (dual) drainage system is optimal for the desired severity, which typically is interpreted as “no” or “acceptable” levels of impacts in terms of damage, loss of life, business interruptions and traffic impacts. Preferably, this point is established with the help of a Triple Bottom Line (TBL) analysis, incorporating social, economic and environmental aspects. Unfortunately, given that most stormwater professionals largely focus on achieving the desired objectives associated with the level of service that is to be met, the sensitivity of the system to more severe events is usually unknown.
When evaluating the resiliency of the (dual) drainage system from a flood control perspective, as well as potential options to enhance the resiliency, it is important to first of all consider how both components interact with each other. In Figure 2, the runoff hydrograph is conceptually represented as a triangle. In case of heavy rainfall, part of the runoff is intercepted by the storm sewer system at the catchbasins or other inlets, which act as the connection between the overland drainage and storm sewer systems. The balance of the runoff hydrograph, i.e., the portion above the horizontal line that denotes the capacity of the storm sewer system, needs to be accommodated by the overland drainage system. This excess runoff either needs to be conveyed away as overland flow or needs to be temporarily stored. Examples of this storage include depressions or sags created along roadways or in parking lots, rooftop storage or underground storage. In many occasions runoff cannot be practically conveyed away as overland flow as it may transfer the excess runoff downstream where the flooding impacts might be even worse, or because the force of the moving water associated with the overland flow may wash people and even vehicles away. Book 6 of Australian Rainfall and Runoff—A Guide to Flood Estimation provides an in-depth overview of this phenomenon (Commonwealth of Australia, 2019). In that case, all excess runoff needs to be temporarily stored and slowly released into the storm sewer system after the storm event (Figure 2).
The planning of our communities from a roadway layout, open space/green space and grading perspective is therefore a subtle balance between overland flow conveyance and detention storage. The provision of distributed open space/green space provides the opportunity to move the detention storage requirements from our roadways, thus reducing traffic impacts. The provision of linear open space and retaining ephemeral/intermittent streams provide safety valves in our communities during extreme events. Appropriate contouring is specifically important in steeper communities where it may be more difficult to provide detention storage in sags along the roadways: having the roadways follow the contours is then crucial. However, the grading design of the properties on the downslope side of those roads then becomes crucial as well to ensure that the runoff will not spill into or between homes.
As is well-known, the shape and magnitude of a runoff hydrograph is a function of many factors, including:
• Intensity and duration or shape of the hyetograph;
• Size, shape, slope, imperviousness, and infiltration characteristics of the contributing catchment; and
• Antecedent conditions within the catchment as well as of the available storage, natural or designed.
The amount of runoff to be retained is a function of the above factors, but, as can be seen from Figure 2, also of the capacity of the storm sewer system or downstream system. As can be deduced from Figure 2, this volume to be retained is not a linear relationship as the storage requirements will reflect both (a) the absolute difference in flow rate and (b) the duration of time that the capacity of the receiving system is exceeded. Normalizing the capacity of the storm sewer system or downstream system on a unit area basis by dividing the capacity of the conveyance system at a point of interest by the size of the contributing catchment, i.e., the Unit Area Release Rate or UARR, provides a simple and quick method to evaluate the sensitivity of a drainage system. Given that many communities practically face a storage deficit, a comparison of the required normalized storage capacity as a function of the imperviousness and UARR against the available storage capacity allows for a rapid assessment of whether adequate storage is available within a drainage system, see e.g., Figure 4.3 of Calgary's Stormwater Management & Design Manual (City of Calgary, 2011).
Another way to look at the UARR is that it reflects the drain down or emptying time of detention/retention storage. This very same concept has been used successfully in creating performance guidance for LID measures in Australia (Argue, 2013). When sufficiently large there is little risk of the capacity of the storage unit not being available when the next storm hits. Continuous simulation techniques and frequency analysis of annual maxima are required when the UARR is small.
In the case of the smaller stream/creek flooding mechanism, the minor and major systems are combined. However, the same concept as illustrated in Figure 2 still applies in that runoff in excess of the capacity of the downstream drainage system should be detained to prevent the occurrence of flood damages along the lower reaches of the receiving smaller stream or creek. This detention is typically in the form of dry or wet ponds that accommodate the runoff from an entire community or subdivision.
The increase in peak runoff rate as a function of development is site- and event-specific, varying from relative minor increases in the East and West Coast provinces, both subject to long-duration soaker type storm events, to very significant increases in the semi-arid regions of the British Columbia Interior and western prairies. In many cases, as illustrated conceptually in Figure 3, the UARR of the receiving smaller streams or creeks is an order of magnitude smaller than that of the storm sewer system within the upstream subdivision. In fact, in the semi-arid Calgary area, the difference is in the order of 50 to 100 times the pre-development UARR. As a result, urban communities are more susceptible to localized, short-duration, high-intensity summer thunderstorms as opposed to receiving streams which are more susceptible to widespread, long-duration, high volume but lower intensity synoptic systems, which may be worsened by melt conditions.
From a resiliency perspective, this has significant consequences for the rate and volume that the drainage system has to be able to accommodate and, in turn, the type of drainage infrastructure that is feasible or required. As shown in Figure 1, the minor or storm sewer system accommodates the runoff from the more frequent, less severe events while the major system kicks in when the capacity of the storm sewer system is exceeded. In practice, little guidance is available on how a dual drainage system can be made more resilient.
In view of the fact that the minor system is typically composed of a closed pipe system with fixed dimensions, and hence has an upper capacity limit, the only practical way to minimize the risk of the storm sewer system from overloading is through the installation of flow controls at all inlets. This means that the major system will be the main conduit to provide the necessary resiliency either by conveying excess runoff away, or by detaining the excess runoff.
The practice of storm sewer system design is typically straightforward, often utilizing simple sizing techniques such as the Rational Method and Manning's equation with limited consideration of the actual detailed hydraulics within these systems. Application of the Rational Method inevitably results in a decrease of the normalized capacity of the storm sewer system when moving downstream. It is therefore only acceptable for small catchments that do not have detention storage. A design based on a UARR approach at least overcomes this specific disadvantage of the Rational Method; however, the performance of the system will still be at the mercy of the extent to which the actual hydraulics have been considered.
The energy losses associated with bends and junctions can be considerable, increasing when the pipe is flowing full (Hager, 2010; Ma et al., 2017). Often conduits may be sufficiently steep that they actually are in a supercritical flow regime. The conditions required to ensure that the flow can actually enter these steeper conduits is often ignored. In addition, this may lead to unintended hydraulic jumps which may lead to sudden pressurized flow and flooding but also to undesirable hydraulic transients when air gets trapped in the sewer system (Liu et al., 2020; Qian et al., 2020). The transition from steeper to flatter pipes is a well-known location where hydraulic jumps might occur; however, they may also occur at bends or at disturbances in the system created by the deposition of gravel and sediments, debris or even misaligned joints (Tang et al., 2020). Unfortunately, most rainfall-runoff models used in storm sewer design practice currently do not properly account for supercritical flow conditions which constitutes a significant gap in design practice.
Surcharge conditions of the storm sewer system are not necessarily unacceptable as long as the system has been constructed properly so that there will not be water exfiltrating and subsequently infiltrating at the joints, leading to voids around the pipe and possible collapse, and as long as it will not lead to flood damages within properties whose weeping tiles are connected to the storm sewer system. Other considerations are that the surcharge conditions may lead to the reduced interception by catchbasins or unintended hydraulic transients within the system. For this reason, CSA W204 recommends that the “conduits in the minor system should not surcharge for the major design event flows”; however, it recognizes that surcharge at times cannot be avoided.
In practice, little guidance exists in drainage manuals pertaining to the dimensioning of the overland drainage system to accommodate the runoff from more severe storm events. City of Calgary (2011) provides some guidance with respect to either the design flow rate to be used for the sizing of overland emergency escape routes at storm ponds (accommodating the runoff from an entire community or subdivision) or additional freeboard to be provided at roadway sags in case the spill route is not along a roadway.
Three components are crucial in the creation of a resilient overland drainage system:
1. Continuous flow route;
2. Appropriate freeboard to building entrance elevations; and
3. Appropriate setbacks.
It is paramount to evaluate the performance of an overland drainage system downstream from a community or pond to a point that can be considered an “adequate outlet.” An adequate outlet is a location where there are no more downstream capacity bottlenecks, when considered on a normalized basis. Examples include large rivers or lakes.
It is difficult to create continuous flow routes in flat areas. Options to provide resiliency in those instances include:
• Provide additional capacity in storm ponds (e.g., provide a 1:500 year capacity);
• Prohibit basement development;
• Increase the discharge into the downstream storm sewer system; or
• Optimize the discharge from storage facilities (e.g., real-time control with operated gates or vortex devices instead of orifice plates).
Freeboard represents the vertical separation between the water level for the design event and the entrance elevation to a building or other structure. Supplementary Table 1 summarizes various factors that are or could/should be accounted for when setting freeboard levels. As shown in Supplementary Table 1 many of these factors are usually (e.g., risk acceptance, precipitation uncertainty and operational uncertainty) or have been historically ignored (e.g., climate uncertainty and community density uncertainty) thus reducing the overall resiliency of the drainage system.
In many instances, freeboard is only in the order of 150 mm (6”) to 300 mm (12”). In Calgary, the freeboard is increased in some instances:
• Overland emergency route via a walkway rather than via a public roadway; or
• In the absence of a proper overland emergency escape route from storm ponds.
The potential consequences of ignoring these uncertainties are discussed below.
Setbacks represent a horizontal separation from a water course or water body to a point of interest, being a building, structure or property line. They are usually applied in the case of natural systems, e.g., rivers, creeks, lakes, and wetlands. Setbacks are typically not applied in the case of man-made drainage infrastructure, except possibly along the perimeter of stormwater ponds and wetlands. Many urban drainage models operate on a 1-dimensional link—junction/node basis with the risk of flooding evaluated based on the water levels in the junctions/nodes, thus ignoring the width of flow within the links. The advent of 2-dimensional modeling techniques provides for an enhanced analysis that reduces these risks which are specifically relevant for narrow overland flow paths.
Floodplain mapping of riverine and small stream/creek systems is usually based on the premise that the cross-section remains fixed in time, often ignoring the morphological evolution of these systems. In the case of encroachment of urban development, extensive and expensive hard protection will then be required at some moment in time to arrest the evolution of the stream. Bioengineering approaches may provide a more environmentally sensitive approach to the protection works; however, they do not take away from the need to fix the stream in place.
Setback policies that prohibit development within the so-called meander belt reduce the risk of stream movement impacting adjacent buildings or infrastructure as long as the overall geometry of the meander belt is maintained when the upstream watershed is developed. This means that the hydro-modification of our development and stormwater management practices should be considered as well, which is typically governed by the more frequent day-to-day runoff events. In summary, in the case of specifically the smaller streams and creeks, flood protection should not only consider the infrequent, extreme events but also the more frequent, day-to-day runoff events through appropriate stormwater management in the uplands.
Supplementary Table 1 presented several factors that are usually or have historically been ignored as part of the analysis of drainage systems:
• Risk acceptance;
• Precipitation uncertainty;
• Climate change uncertainty;
• Densification uncertainty; and
• Operational uncertainty.
During the preparation of CSA W204, two methods were discussed to account for the climate change uncertainty:
1. A stress test approach; and
2. Accommodating precipitation based on updated Intensity-Duration-Frequency (IDF) curves and climate databases that incorporate climate change.
A stress test approach is commonly used when one wants to evaluate the response of a system to certain stressors and make adjustments, as needed. The Committee responsible for the preparation of CSA 204 decided to adopt the second approach given the challenge to clearly define when adjustments would have to be made, and to simplify and streamline the design, review and approval process. This still leaves practitioners sufficient discretion to what extent they desire to incorporate climate change estimates, albeit within the climate data used for analysis purposes. The proposed increases in precipitation depths and intensities reflecting climate change vary from about 10% to over 50%, depending on the time line, climate scenario adopted, and methodology used (e.g., global or regional circulation models or application of Clausius-Clapeyron principle).
To illustrate the sensitivity of a dual drainage system and provide insights as to how resiliency can be added to our urban communities, a stress test analysis of several of the above factors as well as the shape of the design storm events was conducted. Figure 4 illustrates the layout of the recently designed community in northeast Calgary which was used to evaluate the performance of the dual drainage system within the subdivision as well as the performance of the downstream storm pond.
CSA W204 recommends a 1:100 year design event for the analysis of a dual drainage system that is to service new residential communities in Canada. However, the level of service does not necessarily need to be the same for each and every type of impact. For instance, it may be lower for traffic impacts depending on the type of roadway, and the amount and speed of traffic. Conversely, it may be higher for “critical infrastructure” that is vital to the continuance of our communities (Alberta Infrastructure, 2017). The rainfall depths corresponding to the 1997 IDF curves, as shown in Table 1, were used as part of the stress test analysis.
Table 1. Rainfall depths (mm) for design storm events in Calgary (City of Calgary, 2011; GHD, 2020).
Given that precipitation estimates are derived from frequency analysis they are subject to uncertainty, and this uncertainty is greater for longer return periods (i.e., further away from mean or median values). While this has usually been ignored in stormwater management analysis, understanding the estimated uncertainty is important for application of the resulting estimates for project design. Confidence intervals present a range of statistical estimates where the true values are reasonably expected to lie, and illustrate the reliability of estimates of a fitted frequency distribution. The upper and lower boundary values of the confidence interval are called confidence limits. The size of the confidence interval depends on the confidence level, for instance 99, 95, or 90%. Typically, a 95% confidence interval is used. Table 1 includes the upper level confidence limits for the updated 2020 IDF curve. Given that these values are less than the rainfall depths for the different design events analyzed as part of the risk analysis, they were not included in the stress test analysis.
From a stormwater management perspective, climate change simply represents changes in the climate data used for the analysis. Table 1 presents up-to-date 2020 IDF curve estimates and estimates representing anticipated conditions in the 2050s and 2080s, based on the RCP8.5 climate scenario.
As identified above, the amount of imperviousness is a major factor to be considered as part of stormwater management analysis. Usually, this amount is assumed to be fixed in time, with densification only being considered in older communities that are subject to redevelopment and infill development. Figure 5 illustrates the potential impacts due to densification and climate change.
As shown in Figure 5, the amount of runoff to be accommodated by the dual drainage system increases as a result of densification and climate change expectations. There is a significant difference in that policy decisions as a function of the type of re- or infill development that occurs may reduce the impacts of densification; however, the entire drainage system will be subjected to the changes in precipitation when they occur.
In view of the fact that modern subdivisions already feature much higher imperviousness ratios than those built in the last century, this aspect was left out for the stress test analysis. Additional discussion is presented below as part of the evaluation of how to achieve resilience in existing communities.
While the analysis of natural water courses may reflect the variation of vegetation during the course of the year and over the years, the analysis of dual drainage systems is usually based on the premise that the system is clean and operates as intended. Observations of operational staff, however, point at situations where catchbasins and other inlets may be clogged or where extensive depositions of gravels and other sediments in the storm sewer system may result in significant capacity reductions. Examples include the deposition of gravels and pipes in the pipes with standing water, upstream of wet ponds (Tang et al., 2020). Other potential problem spots are box culvert sections, especially when downstream of areas with extensive gravel surfaces. Besides the need for frequent inspections and subsequent cleaning, provisions to capture these materials upstream of potential problem locations, and the installation of backwater valves in service connections should be considered.
Some examples of how operational uncertainties have been incorporated in the analysis of dual drainage systems exist, for instance:
• As part of the derivation of the emergency spill conditions at storm ponds, in Calgary it is assumed that the control structure at these storm ponds is not operational during the entire storm event;
• The analysis of dual drainage systems on behalf of the insurance industry ignores the contribution of the storm sewer system, focusing entirely on the overland drainage system.
Operational uncertainties were not included as part of the stress test analysis.
In addition to the total rainfall depth and the duration of the design storm event, the shape of the hyetograph is also an important aspect. In many communities a Chicago design storm distribution is used, whether appropriate or not. As part of the stress test analysis, several design storms distributions were used including the Chicago, the Huff, the SCS Type II and AES Normal distributions (Watt, 1989). Supplementary Figure 1 illustrates that the selection of the design storm distribution is important and can outweigh the effects of other factors such as densification and climate change. Above all, the selection should be representative of the region in question. Given that the distributions were last evaluated over 30 years ago in many regions, it may be advisable to revisit them, especially in the face of climate change impacts.
Integrated stormwater management intends to optimize multiple objectives including flood control, water quality control, minimization of hydro-modification, accommodating growth, and effective asset management. All of this should occur with a lens of climate change, while maximizing the vibrancy of our communities and achieving secondary objectives such as public health and biodiversity. LID (also known as sustainable urban drainage or water sensitive urban design) is an integrated stormwater management strategy that seeks to mitigate the impacts of increased runoff and stormwater pollution by managing runoff as close to its source as possible. It comprises a set of site design strategies that minimize runoff by providing distributed, small scale structural practices that mimic natural or pre-development hydrology through the processes of infiltration, evapotranspiration, harvesting, filtration, and detention of stormwater (CSA Group, 2018). LID measures such as bioretention areas and bioswales, soil cells, infiltration trenches, permeable pavement, green roofs, trees and shrubs as part of resilient landscaping techniques including rain gardens, and use of harvested rainwater or captured stormwater are being implemented to protect the water quality of receiving water bodies or minimize hydro-modification impacts but the question has arisen to what extent they may contribute to flood control as well. These LID measures should not only have resiliency to safely pass the runoff from severe storm events without being destroyed or requiring extensive repairs, but, by their nature, LID measures also feature detention and retention. While, as illustrated in Supplementary Figure 2, their primary focus is on the more frequent, less severe events, not on the infrequent, severe events that are the focus of flood control, inherently, the detention and retention that are part of the LID measures may also function during the extreme events provided their detention/retention capacity is available as a function of their emptying/drain-down time.
Seven main aspects should be considered pertaining to the analysis, design and operation of LID measures:
1. The precipitation patterns and amounts that are prevalent within the area in question;
2. Seasonal timing of high precipitation amounts;
3. Opportunities to reduce the amount of runoff;
4. The size of the LID measures relative to the size of the area draining into them;
5. Opportunities for detention/retention storage;
6. The emptying/drain-down time of the LID measures; and
7. The fate of the runoff that leaves the LID measures.
Each of these aspects is discussed below.
Given their nature of first of all minimizing contaminant loadings or minimizing hydro-modification effects, both of which are largely associated with the smaller, more frequently occurring storm events, LID measures can contribute to controlling pluvial flooding, provided that the I/P ratio, where “I” stands for the size of the tributary catchment area, and “P” stands for the footprint of the LID measure in question, see below, is sufficiently low and the normalized percolation rate, see also below, is sufficiently high. Opportunities to control small stream/creek flooding are smaller, unless the I/P ratio is sufficiently low and normalized percolation rate is greater than the precipitation intensity provided that the entire watershed is not saturated.
In those areas where the timing of the highest seasonal precipitation occurs in the summer months, thus coinciding with the highest evapotranspiration rates, those retention practices that utilize evapotranspiration provide maximum flood control opportunities. Examples include practices such as rain gardens (intensive) green roofs, soil cells, rainwater harvesting, and stormwater capture and use, which are ideal in the semi-arid regions of the British Columbia Interior or Alberta. The use of these practices is less beneficial along the West and East Coasts of Canada.
As can be seen from Figure 5, reducing the amount of runoff may be beneficial in reducing the amount of runoff to be accommodated by the overland drainage system. The extent to which runoff reduction is beneficial depends on the degree that it is implemented. In those areas where extensive runoff reduction is being practiced to minimize contaminant loadings or to minimize hydro-modification effects, the benefits will be greatest. Examples include resilient landscaping techniques and rain gardens, provided that the I/P ratio is sufficiently low; intensive green roofs; bioretention, soil cells and permeable pavement, all without underdrains and on the premise that the I/P ratio is also sufficiently low and the normalized percolation rate is sufficiently high; infiltration trenches provided that the I/P ratio is also sufficiently low and the normalized percolation rate is sufficiently high; and rainwater harvesting and stormwater capture techniques provided that the demand is sufficiently high. Continuous simulation techniques should be utilized to analyze the benefits of these practices and support the evaluation of what should be assumed for antecedent conditions in case of single event analysis.
A key parameter for the evaluation of the suitability of LID measures is the ratio between the footprint or capacity of the LID measure and the size of the tributary catchment area. One way to express this is the I/P ratio. The higher the I/P ratio, the greater the hydrologic and potential contaminant loadings, specifically if the latter are “rate-governed” such as is the case for readily erodible surfaces (e.g., dirt or gravel). Note that the maintenance frequency should increase as a function of the I/P ratio. Note also that one cannot simply extrapolate LID performance data across the country; rather, the performance data should be normalized based on the differences in precipitation depths. Those practices that have low I/P ratios are usually more suitable to contribute to pluvial flood control. Practices with high I/P ratios will only contribute to small stream/creek flood control if the normalized percolation rate, see below, is sufficiently high.
As identified above, storage is vital to flood control. In the case of LID measures, the higher the I/P ratio, the lower the amount of storage available on a normalized basis. As such, the use of bioretention areas, soil cells and infiltration trenches may be limited in controlling pluvial flooding if the I/P area becomes too large. These practices may offer some benefits to controlling small stream/creek flooding if the I/P ratio is sufficiently small and the normalized percolation rate is sufficiently high, provided that the entire watershed is not saturated. LID measures that have intrinsic retention storage such as rainwater harvesting and stormwater capture can be very effective for specifically pluvial flood control provided that the water demand is sufficiently high.
Also, as identified above, the emptying or drain-down time of stormwater management storage measures is vital as to their potential performance. As illustrated in Figure 3 above, a relatively small storage capacity can be adequate to control pluvial flooding provided that the UARR is large. The same storage capacity would be inadequate to control pluvial flooding if the UARR is small given the potential that it may not have drained down when the next event hits the catchment. Continuous simulation techniques will assist in evaluating this risk.
Percolation into the subsoils provides a different avenue for an LID measure to empty or drain down. Similar to the UARR, a normalized rate should be used based on the I/P ratio of the LID measure. Assuming a fully paved catchment without any attenuation, a 70 L/s/ha UARR, which is the minimum rate permitted in Calgary for the development of new subdivisions, translates into a normalized intensity of about 25 mm/hour. Conversely, a 2 L/s/ha UARR reflecting the capacity of some of the creeks in the Calgary area would then translate into a normalized intensity of about 0.7 mm/hour. Practically, this means that the normalized percolation rate needs to be at least 0.7 mm/hour for LID measures to be effective in controlling small stream/creek flooding in the Calgary area.
A last item to be considered is the fate of the runoff leaving the LID measures. In the case of measures that have an underdrain the infiltrating runoff will flow toward the storm sewer system. This means that these features will have little to no benefit in controlling small stream/creek flooding. They may provide some pluvial flooding benefits provided that the underdrain is equipped with a flow control.
In the case of LID measures that rely on percolation into the subsoils the ultimate fate of the infiltrating runoff should be established, if possible. Appropriate separation setbacks should be provided to ensure that this runoff will not make its way into the backfill surrounding a building or into the sanitary system via utility trenches. In addition, the downward flux should be similar to pre-development conditions to minimize the risk of slope instability downslope of the site in question. The provision of moisture proofing and weeping tiles may minimize the impacts on buildings; however, the percolating runoff would then still make its way relatively quickly to the downstream storm sewer system. Increases in inflow and infiltration into the sanitary system should be avoided.
Practitioners should have a good appreciation of all of these factors when evaluating the potential benefits of the various types of LID for their region. For instance, Table 2, adapted from City of Calgary (2011), suggests that even rain gardens can be remarkably effective in contributing to flood control for short duration, high-intensity storm events, solely based on the amount of storage that can be provided within the 100 mm ponding depth provided that the I/P ratio is appropriate. In this case the I/P ratio was assumed to be equal to 3, meaning that the ponding depth provides storage to retain at least the first 25 mm of runoff from the area draining into the rain garden, assuming that the rain over the rain garden equals the runoff from the tributary hard area and ignoring the storage that would be provided over the area covered by the side slopes. Additional storage might be possible when the storage within the soil mass and percolation into the subsoils were considered as well. The latter was ignored in recognition of the fact that subsoils are often heavily compacted as part of modern earthmoving and building practices.
Table 2. Potential efficiency of rain gardens in Calgary (adapted from City of Calgary, 2011).
However, the potential benefits of rain gardens for flood control decrease when the I/P ratio increases or in the case of long-duration storm events with high rainfall depths, especially if they occur, in the case of the West Coast, during the fall and winter months when evapotranspiration rates are minimal and the entire watershed may be saturated. While these storm events usually feature lower intensities than observed during summer thunderstorms, thus not resulting in extensive flooding within the uplands of our urban communities, the resulting runoff may result in small stream or creek flooding. Only if the percolation rate into the subsoils is comparable to the UARR of the receiving streams, prorated to the I/P ratio, will these rain gardens provide effective benefits.
For comparison purposes, Figure 6 illustrates the normalized amount of storage available within various types of source control measures as a function of their respective I/P ratios. For comparison purposes, green roofs, which usually have an I/P ratio = 0, have a normalized storage capacity ranging from about 31 mm for a 100 mm thick extensive roof to about 93 mm for a 300 mm thick intensive roof.
Figure 6. Normalized Storage Capacity available within Source Control Measures; Assumptions: Rain Garden 100 mm ponding; 300 mm thick soil; porosity—field capacity = 0.13; Bioretention 300 mm ponding; 450 mm thick media; porosity—field capacity = 0.13; Soil Cell no ponding; 1,200 mm thick media; porosity—field capacity = 0.13; Permeable Pavement no ponding; 600 mm thick rock; void ratio = 0.40.
From Figure 6 it is clear that LID provisions can provide significant amounts of (normalized) storage, even substantially greater than rain gardens; however, where rain gardens have little to no incremental costs over typical landscaping practice, other source control measures can have substantial costs associated with them as a function of their composition. As touched on, a TBL analysis should be conducted as to which gray and green infrastructure provisions, or combination thereof, has the greatest value to the community in question.
A combination of resilient landscaping practices, green roofs and rainwater harvesting has been used successfully in for instance Germany and France to address pluvial flooding and combined sewer overflows, specifically for redevelopment in older communities. On the other hand, given the large volumes that need to be accommodated to protect downstream water resources, resilient landscaping and infiltration practices are needed to provide small stream and creek flood control. If the infiltration rate is relatively small, as will be the case in large parts of Canada, detention and retention ponds will be needed.
These concepts are further illustrated in Figure 7 and Table 3 which relates the type of LID and other stormwater management measures to their I/P ratio and drain down/emptying time. The exact boundaries of the applicability of these features will vary across the country; however, the illustration should apply in all regions except that the flood control benefits of LID for small stream/creek protection in case of the long duration, high rainfall depth events will be limited along the East and West Coast provinces.
The retention and role of natural systems, specifically wetlands and smaller streams and creeks, as part of the stormwater management system has received considerable attention in recent years. Even though the financial aspects of natural systems in terms of asset value and operation and maintenance needs have been historically largely ignored, most of their functionality has actually been incorporated all along. In fact, more wetlands have been lost in agricultural settings than in urbanizing areas, simply reflecting the spatial extent of agriculture vs. urban development.
Wetland functions include (a) flood control, (b) water quality enhancement, (c) groundwater recharge, (d) habitat, (e) biodiversity, and (f) recreational aspects. Of these functions, flood control, water quality enhancement and recreational aspects are essential components of modern-day stormwater management, while progressive approaches also address habitat and groundwater recharge. As such, retention of existing wetlands in urban areas only provides more resiliency if the dynamic storage of flood control would have otherwise been reduced for extreme events. In many communities existing, permanent wetlands are retained to create vibrant communities which provide habitat and biodiversity granted that the amount and quality of stormwater entering them do not inadvertently lead to degradation of these existing wetlands. Urban wetlands need urban stormwater for their survival, however, the quantity and quality of that stormwater needs to be controlled, specifically for day-to-day events.
Likewise, the need to stay within the morphologic and environmental carrying capacity is also acute for smaller streams and creeks. These water courses indeed provide significant overland conveyance; specifically, the ephemeral and intermittent ones have historically been ignored as to their capacity to provide emergency relief during extreme storm events. However, they cannot be seen with a purely utilitarian mindset, and will not replace urban storm sewers. Hydro-modification changes may significantly reduce the resiliency of these systems, making them more prone to accelerated movement during extreme storm events, thus jeopardizing infrastructure and structures adjacent to them. Retention of riparian areas along these streams is important from both a flood control and environmental protection perspective; however, riparian areas should not be seen as replacing the need for adequate water quality treatment within the urban uplands. They themselves have limits in their carrying capacity, from a hydrologic and environmental perspective. As such, any changes over pre-development or existing development conditions may unravel these areas, thus severely impacting their resiliency.
The analysis of the stress test case study was conducted for the Cornerstone subdivision in northeast Calgary, which is a 146.45 ha relatively flat, residential subdivision serviced by a dual drainage system, and draining into a storm pond that has a discharge rate of 2.5 L/s/ha, ultimately discharging into Nose Creek. The analysis of the dual drainage system upstream of the storm pond was conducted with a 1 h duration design event with Chicago distribution in accordance with City of Calgary guidelines. Longer durations were not deemed necessary as the storm sewer system had originally been designed using a UARR in excess of 70 L/s/ha. The analysis of the pond was conducted with a 24 h duration design event, also with a Chicago distribution. Although longer duration events may in reality not display the peaky nature reflected in a Chicago distribution, this conservative design event has historically been used in Calgary as it allows for the analysis of both the peak overland and storm sewer system flows and potential backwater conditions from the storm pond in one single analysis.
For all scenarios examined, impacts on the storm sewer system were found to be limited reflecting the widespread provision of inlet control devices in catchbasins and the installation of flow controls at multi-family residential and industrial/commercial/institutional (ICI) properties. First, for the various storm events outlined in Table 1, the operation of the overland flow system was examined with respect to the depth of ponding in low points or sags (see Figure 8A), the magnitude of the overland flow (see Figure 8B), the combination of the depth and velocity of flow reflecting the risk of being swept away by the force of moving water, and the overland flow hydrograph into the storm pond (see Figure 9A).
Figure 8A suggests that the maximum depth of ponding in sags and other low points will only increase by about 10 to 20 cm, which is manageable from a freeboard perspective. Figures 8B,C shows a definite increase in the amount of overland flow, especially at the downstream end of the overland drainage system; however, there are only a few places where the risk of people being swept away by the force of moving water exceeds Alberta's provincial guidelines.
Figure 9A illustrates that the overland flow into the storm pond was virtually zero for the original 1:100 year design scenario. While the peak flow rate into the storm pond increased significantly, the volume under the hydrographs was actually not that great, ranging from about 5,450 or 37 m3/ha (3.7 mm) for the 1:200 year event to about 8,820 or 60.2 m3/ha (6.0 mm) for the 1:500 year event, and about 10,800 or 74 m3/ha (7.4 mm) for the 1:1,000 year event. Comparison of these increases in runoff volumes with the normalized detention/retention storage capabilities of LID shown in Figure 6 suggests that distributed storage and runoff volume control (e.g., through the implementation of LID) within the upstream catchment can be another effective avenue in providing resiliency to more severe storm events. This is specifically of interest in catchments that are steeper than the one featured in this stress test analysis. In steeper catchments opportunities for dynamic storage within roadway sags will be smaller compared to flatter catchments increasing the risks of excessive overland flows sweeping people away.
The analysis was not repeated for the climate change scenarios as they would have yielded a similar response. As to be expected, Figure 9B and Table 4, presenting the results for the downstream storm pond, again based on the application of a 24 h design storm with Chicago distribution, illustrate that the storage requirements in the storm pond will increase for the more severe storm events, and by extension, climate change scenarios.
Options to accommodate the additional runoff to storm ponds include:
• increasing the footprint of the pond;
• making the pond deeper, either by increasing the freeboard or by lowering the Normal Water Level, thus increasing the active storage depth;
• increasing the discharge rate for extreme events, if possible, or by changing the type of flow control from a typical orifice type to a vortex type allowing for a more continuous discharge rate that is closer to the maximum permissible discharge rate into the downstream system;
• real-time control of discharges, for instance by lowering the water level in the pond prior to a large storm event occurring; and
• runoff volume control/retention storage within the upstream catchment, for instance through the implementation of LID which would mean a move toward a water retaining landscape rather than the currently typical water shedding landscape. The water shedding should be limited in the vicinity of the structures—protection measures such as lining used for instance for planters could be considered.
Supplementary Figure 3 suggests that the benefits of enhancing the performance of the flow control in the storm pond are limited in case of the Calgary case study. This is a consequence of the UARR only being 2.5 L/s/ha. The benefits should be more pronounced for instances where the UARR is greater.
The usefulness of additional detention storage in the upstream catchment will be limited as the discharge rate from the storm pond is governed by the capacity of the downstream creek into which this storm pond ultimately discharges, unless this additional storage would have the same UARR as the storm pond itself. Real-time control of the operation of the additional detention storage may be beneficial to ensure that the capacity will not have been utilized during the earlier stages of the storm event.
When comparing opportunities to add resiliency to urban drainage systems across Canada one has to account for the differences in climate regions driving the nature of the governing design events and the potential UARR to the receiving water bodies. Using the Calgary case study as a starting point one can evaluate the sensitivities and hence resiliency opportunities in other regions when one considers these differences.
Stormwater management analysis in Calgary is based on the application of Chicago distribution type storm events, with duration of 1 h (new subdivisions), 4 h (established communities) and 24 h (storm ponds and any community subject to backwater conditions from a storm pond). Given the low UARR of Calgary's receiving water bodies (i.e., only in the order of 1 to 2 L/s/ha), the shape of the hyetograph is of little significance for the analysis of storm ponds. The Chicago distribution, which reflects the peaky nature observed for short-duration events, is embedded in longer duration events so that the peak flow rates, pond filling and backwater conditions from a storm pond can be analyzed with only one design storm event. The short 1 h duration event used for the analysis of the dual drainage upstream of storm ponds in case of new subdivision development reflects the relatively short drain-down time of roadway sags which usually is only in the order of 15 to 20 min given that the design UARR for the minor system is typically in excess of 70 L/s/ha. The 4 h event for established communities reflects that they typically show a much lower UARR (i.e., 10 to 20 L/s/ha) and hence experience longer drain-down times.
As described above, the Cornerstone case study represents a relatively flat community with flow controls in all catchbasins. As demonstrated there will only be a limited increase in the depth and velocity of flow along the streets for events in excess of the 1:100 year condition. The most downstream overland flow segments of the 145 ha catchment shows a substantial increase in flow rate; however, the associated incremental increase in runoff volume is relatively small (i.e., only in the order of 5 to 10 mm) for these short-duration, high-intensity convective summer thunderstorms. The main impacts of the more severe events will be associated with the downstream pond.
In Quebec a 3 h duration design storm with Chicago distribution is typically used for the analysis of the dual drainage system upstream of a storm pond. Figures 10A,B, which compares rainfall amounts across Canada, shows that precipitation depths tend to be substantially greater in central Canada compared to the western provinces (Government of Canada, 2015). Nevertheless, even though there are downsides to the use of IDF curves in that they are an amalgamation of different storm types, for the convective summer thunderstorms one would expect to see a similar response in Ontario and Quebec to more severe storm events with respect to the performance of the dual drainage system upstream of the pond. In spite of the differences in rainfall depths, given the similarity in storm behavior, this suggests that either an increase in freeboard, an increase in UARR and/or distributed retention storage using LID could increase the resiliency for new subdivisions, upstream of the storm ponds. The benefits of LID are expected to be less in central Canada compared to the western prairies reflecting the greater precipitation depths.
Figure 10. Comparison of IDF Curve Data of 1:100 Year Event (Government of Canada, 2015). (A) Short Duration: 5 min to 24 hours and (B) Long Duration 5 min to 30 days.
As discussed above, impacts on established communities are expected to be more severe compared to new subdivisions given that they have a lower UARR to start with, therefore being more sensitive to longer duration events, and typically have a poorly laid out overland drainage system. Similarly to new subdivisions, given the greater precipitation depths, impacts of climate change and densification may be more pronounced for established communities in central Canada.
With respect to the sizing of storm ponds, the permissible release rate of 10 L/s/ha (1:10 year event) to 30 L/s/ha (1:100 year event) to receiving water bodies used in Quebec is considerable greater than the rate in use in the western prairies. One downside to the use of short-duration IDF curves (i.e., the 5 min to 24 h duration ones) is that they do not cover the response of the drainage system to longer duration storm events, which would specifically be of concern pertaining to the small stream/creek flooding. In the Calgary area, the use of continuous simulation approaches covers this eventuality. In Quebec, the performance of storm ponds is analyzed for both the short-duration 3 h event with Chicago distribution as well as a longer-duration 24 h event, albeit with a SCS distribution. Figure 11 presents the resulting water levels for the case study pond subjected to these two Quebec events; the permissible discharge rate from the storm pond was increased to reflect typical Quebec discharge conditions. Even though the rainfall amounts in Quebec are substantially greater than those observed in Alberta, i.e., ~124 vs. 90 mm for the 24 h duration events used to generate Figure 11, the normalized storage capacity is smaller reflecting the substantial difference in UARR. Secondly, the difference in UARR is also reflected in the differences of how quickly the ponds empty which will be much faster in Quebec. Another difference between the prairie provinces and central Canada is the analysis of the response of small streams and creeks to Regional Storms such as Hurricane Hazel, reflecting that central Canada will be subject to hurricanes or their remnants, which tend to dump large volumes of rain. There is no equivalency to those types of events in the prairie provinces.
For comparison, currently, convective summer thunderstorms that are common east of the Rocky Mountains are not common in Greater Vancouver, and do not tend to be embedded in the longer-duration storm events. As a result, the more gradual AES or SCS Type II distribution storm events with 12 to 24 h duration are used for analysis purposes. The minor system design is typically based on a 1:5 year event for the low-slope (<3%) communities in Vancouver, increasing to a 1:10 year event for the high slope (>3%) communities along the north slopes. This approach is actually similar to Calgary's approach where a higher UARR is recommended for steeper catchments given the challenges to provide surface storage in roadway sags.
Flow monitoring in Greater Vancouver has demonstrated that the increase in the peak runoff rate in the receiving streams for post-development conditions over pre-development conditions is only in the order of about 30% for the long-duration “soaker” events when the watershed is already saturated regardless (Kerr Wood Leidal Associates Ltd, 1999). As a result, given the relatively short distance to the receiving streams the approach is typically to discharge the runoff safely to those streams with few ponds. Given the saturated conditions in the watershed for these types of events, LID would have little to no benefits from a flood control perspective, and the main resiliency emphasis should therefore be on appropriate freeboard, appropriate setbacks and a continuous drainage system. LID, however, is still needed from a water quality control and hydro-modification perspective as local creeks have experienced significant impacts. As well, LID will also be effective in lessening the impact of minor storms allowing for climate resiliency in the drainage system.
Alberta will show a significantly greater increase in the response for the longer duration events given that the runoff was minimal for pre-development conditions. As a result, the Prairies will see a relatively greater need for runoff rate and volume control compared to e.g., Greater Vancouver, also resulting in larger facilities (when normalized). On the other hand, a similar behavior as experienced along the West Coast for the longer duration regional events may be expected in Ontario, Quebec and the East Coast provinces. The semi-arid interior of British Columbia may see a similar behavior as observed in the western prairies; however, rain on snow events become more significant when one moves further north.
It is noted that, although not observed yet, there is some fear in Greater Vancouver that climate change may result in higher intensity bursts during the longer duration events in the winter months. Any detention storage should be operated such that it is effectively available when needed, i.e., that has not been filled during the early stages of these longer duration events. Real-time control approaches might be the only feasible option to accommodate this scenario.
In summary, in addition to the more traditional detention storage and storm sewer upgrades, LID may have a beneficial role from a flood control perspective, specifically for the dual drainage system in those parts of Canada where the short duration convective summer thunderstorms are governing for the design of the dual drainage system. The benefits of LID diminish—again from a flood control perspective—in the wetter parts of Canada, unless LID is defined as including the large-scale retention ponds used in Calgary for capture and use of stormwater. However, their use is most beneficial in the drier parts of Canada.
With reference to CSA W204, resiliency in new subdivisions development can be enhanced as follows:
• Incorporate climate change considerations as essential input to the analysis by:
° Updating IDF curves to reflect climate change; and
° Updating weather databases for continuous simulation to reflect change
• Consider future densification that may occur;
• Ensure that overland drainage routes are continuous to an adequate outlet;
• Increase the amount of freeboard by 10 to 20 cm;
• Implement appropriate setbacks along smaller streams and creeks;
• Increase the footprint or the capacity of storm ponds;
• Optimize the discharge regime from storm ponds, including real-time control as precipitation may not be evenly distributed;
• Move away from a water-shedding landscape to a water-retaining landscape by implementing resilient landscaping practices; and
• Implement runoff volume control/retention within the catchment to minimize hydro-modification and enhance water quality.
Challenges in older communities include
• A relatively low storm sewer capacity when expressed on a UARR basis, typically much lower than what is seen nowadays in new subdivisions. The system was likely designed based on the Rational Method using runoff coefficient that were reflective of the original low density development;
• The absence of a properly laid out overland drainage system, implemented before the advent of dual drainage principles;
• A lack of freeboard with respect to the entrance to buildings or structures (e.g., doors, windows, parkade entrances);
• Increased imperviousness due to densification; and
• Move toward zero-lot-line development which
° eliminates infiltration, if any;
° reduces originally available sag storage within the road right-of-way; if any, and
° increases risk of runoff entering buildings at low depths of flow/ponding.
These challenges are not only experienced as part of the stormwater system but will also extend to a higher risk of the sanitary system being overloaded due to excessive inflow & infiltration, specifically in areas where sanitary manholes are located in sags without any inflow prevention, or where weeping tiles and downspouts are inadvertently connected to the (public) sanitary system. In some instances, basement flooding caused by overland flow ingression may, in turn, result in the sanitary system being overloaded via the basement drains.
Practically, these deficiencies manifest themselves as storage/conveyance deficits. An order of magnitude estimate of the storage deficit can be found by comparing the required storage capacity using normalized storage relationships as a function of UARR and average imperviousness (such as in use in Calgary) against the available storage capacity based on LiDAR data and surveyed or estimated building entrance elevations. The same normalized storage relationships can subsequently be used to quickly home in on potential relief measures, before elaborate modeling is conducted. Incidentally, problem locations often appear to coincide with the pathway of the original drainage courses that had been covered up when the area in question was first developed (Walesh, 1999).
Opportunities to enhance the resiliency in these older communities will need to be a combination of
• Improved conveyance;
• Detention storage; and
• Runoff volume reduction and retention
The upcoming CSA Standard W210 provides an extensive summary of options that can be considered in enhancing the resiliency of our established communities in the light of the various flooding mechanisms.
The strategies advocated in several of the so-called Cloudburst programs in Europe are inherently not out of the ordinary. Many European cities are serviced by combined sewer systems that had been constructed in an era prior to the adoption of the dual drainage principles. The measures proposed are anticipated to be effective in that they reflect what has been common practice in Canada for the last couple of decades. That said, the proposed multi-purpose use of open and green space will be crucial to enhancing resiliency; single-purpose utilization of space in our established communities is inappropriate given the storage deficit that already exists even before climate change considerations.
The impacts of densification is greater than the mere change in imperviousness. The runoff from downspouts tended to be dispersed and absorbed within the surrounding landscaped areas. However, the soil compaction and destruction of the original soil structure resulting from construction activities also reduced the infiltration potential of the soil, exacerbating an already significant increase in the amount of runoff. Depending on the level of imperviousness the impacts of densification can be even more pronounced than climate change. However, there is a distinct difference in that appropriate land development policies can address the impacts of densification whereas the higher precipitation amounts associated with climate change will need to be accommodated by the drainage system regardless, and on top of any densification effects that may occur. Therefore, it is paramount that these land development policies address all instances where densification occurs, including not only the transformation from single-family residential development into multi-family residential and ICI development, but also the transformation of the original low-density single-family residential development into higher density forms of the same.
Flood control resiliency inherently has environmental benefits in that floods can unleash great volumes of contaminated runoff, thus threatening our water supplies as well as downstream habitat. Locating high risk activities such as chemical storage areas or wastewater treatment plants outside of flood prone areas significantly reduces environmental risks.
In reverse, as demonstrated above, land use, development and stormwater management provisions that provide water quality enhancement or minimize hydro-modification may have positive benefits from a flood control perspective as well, depending on their nature and extent of implementation.
Other resiliency considerations that are related to stormwater management include the notion that:
• Densification and climate change worsen the urban heat island effect that our communities are being subjected to; and
• Climate change may also worsen droughts.
Solutions include greening our communities through
• Implementation of green roofs;
• Enhancing the urban forestry through e.g., soil cells and tree trenches;
• Prohibiting the use of potable water for landscaping purposes;
• Implementing resilient landscaping with appropriate, deep-rooting, native vegetation; and
• Implementing rainwater harvesting and capture of storm water.
All of these approaches may contribute, in varying degrees, to flood control as well. Water balance analyses are needed to evaluate the susceptibility of these systems to extended dry and wet periods to ensure they will be properly designed and operated.
In summary, the following concluding comments are offered:
• The implementation of a dual drainage system approach is paramount to creating resilient stormwater management systems, with flow controls in all connections to the storm sewer system, and appropriate overland drainage system with continuous flow routes, appropriate freeboard and appropriate setbacks. Use of this approach in new subdivision development in combination with appropriate climate change considerations will avoid most of the problems experienced in older, established communities;
• Practitioners should have a thorough understanding of the flooding mechanisms that are important for their region; what events contribute to flooding and how flooding may be influenced by the different kinds of stormwater management provisions;
• Region-specific normalized performance data allows for rapid evaluation of the sensitivity and resiliency of drainage systems as well as of potential relief measures;
• Given the differences in topography and climate conditions and resulting differences in receiving water impacts one needs to exercise caution in extrapolating findings from one region to another region. Practitioners have to thoroughly understand the similarities and differences.
• The main benefits of LID are for water quality improvement and hydro-modification. A transition to a water-retaining landscape and implementation of LID will help in providing resiliency; however, their prime flood control benefit will be for less severe storm events and those components of the dual drainage system governed by the sub 4 h summer thunderstorms.
• Established communities have storage capacity deficits reflecting past development and land use practices. All avenues to provide additional storage and improve conveyance should be considered, with multi-purpose utilization of any open space/green space and land use policies that ensure that densification does not result in a reduced level of service;
• Natural systems such as wetlands and small streams/creeks may contribute to the resiliency of our drainage systems; however, their utilization should be within their innate carrying capacity from a hydro-modification and water quality loadings perspective; and
• Benefit-cost and TBL analysis should be conducted to inform all decision making.
In addition, the following research needs and action items are identified:
• Confirmation of temporal and spatial distribution of storm events, specifically in view of climate change;
• Proper analytical representation of the hydrologic aspects of LID including appropriate antecedent conditions when using single-event analysis;
• Enhance quantification of and provide guidance with respect to use of uncertainty principles in stormwater management;
• Guidance to properly account for supercritical flow conditions in stormwater management design;
• Creation of normalized performance data of LID and other stormwater measures reflecting regional topography and climate, and as function of emptying/drain-down times; and
• Creation of nation-wide TBL tools.
BvD provided the concepts discussed and direction to the rainfall-runoff modeling overseen by CK. WZ and CK contributed to the creation of the figures. All authors provided scope and discussion and contributed to the manuscript writing.
The views expressed in this paper are those of the authors alone, and not the organizations for which they work.
RM was employed by the company Dillon Consulting Limited. CJ and CK were employed by the company Kerr Wood Leidal Associates Ltd., and GR was employed by the company Lasalle NHC.
The remaining authors declare that the research was conducted in the absence of any commercial or financial relationships that could be construed as a potential conflict of interest.
The Supplementary Material for this article can be found online at: https://www.frontiersin.org/articles/10.3389/frwa.2021.671059/full#supplementary-material
Alberta Infrastructure (2017). Flood Risk Management Guidelines for the Location of New Facilities. Edmonton, AB: Alberta Infrastructure.
Argue, J. R. (Ed.). (2013). Water Sensitive Urban Design: Basic Procedures for ‘Source Control of Stormwater. A Handbook for Australian Practice. Urban Water Resources Centre, University of South Australia. Available online at: https://www.unisa.edu.au/contentassets/d8b261f5e4c84572b2cda9e97a4e69aa/johnargue-wsud-basic-procediures-for-source-control-student-edition.pdf (accessed February 15, 2021).
Bush, E., and Lemmen, D. S. (Eds.). (2019). Canada's Changing Climate Report. Government of Canada, Ottawa, ON, 444. Available online at: https://www.nrcan.gc.ca/sites/www.nrcan.gc.ca/files/energy/Climate-change/pdf/CCCR_FULLREPORT-EN-FINAL.pdf doi: 10.4095/314614
City of Calgary (2011). Stormwater Management and Design Manual. Calgary, AB. Available online at: https://www.calgary.ca/content/dam/www/pda/pd/documents/urban-development/bulletins/2011-stormwater-management-and-design.pdf
Commonwealth of Australia (2019). Australian Rainfall and Runoff - A Guide to Flood Estimation. Available online at: http://www.arr-software.org/arrdocs.html (accessed February 14, 2021).
CSA Group (2019b). Technical Guide - Development, Interpretation, and Use of Rainfall Intensity-Duration-Frequency (IDF) Information: Guideline for Canadian Water Resources Practitioners, CSA PLUS 4013:19.
Field, C., Barros, V., Stocker, T. F., Qin, D., Dokken, D. J., Ebi, K. L., et al. (2012). Managing the Risks of Extreme Events and Disasters to Advance Climate Change Adaptation. IPCC Special Report. Cambridge University Press.
Florin, M.-V., and Linkov, I. (Eds.). (2016). IRGC Resource Guide on Resilience. Lausanne: EPFL International Risk Governance Center (IRGC).
GHD (2020). Climate Data for Hydrologic and Hydraulic Analysis, Technical Memorandum #3: IDF Curves.
Government of Canada (2015). Short-Duration Rainfall Intensity-Duration-Frequency. Available online at: https://open.canada.ca/data/en/dataset/15aa455c-9f9f-4405-8eee-4445d02fd35c (accessed February 7, 2021).
Kerr Wood Leidal Associates Ltd (1999). Stormwater Management Strategy for Mission / Wagg Creek System. Report to City of North Vancouver, BC.
Liu, L., Shao, W. Y., and Zhu, D. Z. (2020). Experimental study on stormwater geyser in a vertical shaft above a junction chamber. ASCE J. Hydraul. Eng. 146. doi: 10.1061/(ASCE)HY.1943-7900.0001660
Ma, Y. Y., Zhu, D. Z., Rajaratnam, N., and van Duin, B. (2017). Energy dissipation in circular drop manholes. ASCE J. Irrig. Drain. Eng. 143:04017047. doi: 10.1061/(ASCE)IR.1943-4774.0001241
Meyer, M., Flood, M., Keller, J., Lennon, J., McVoy, G., Dorney, C., et al. (2014). Volume 2: Climate Change, Extreme Weather Events and the Highway System: Practitioner's Guide and Research Report. NCHRP Report 750, Transportation Research Board, Washington, DC.
National Research Council (2012). Disaster Resilience: A National Imperative. The National Academies Press, Washington, DC.
Public Infrastructure Engineering Vulnerability Committee (PIEVC) (2020). PIEVC Program. Available online at: https://pievc.ca (accessed February 12, 2021).
Qian, Y., Zhu, D. Z., Liu, L., Shao, W. Y., Edwini-Bonsu, S., and Zhou, F. (2020). Numerical and experimental study on mitigation of storm geysers. ASCE J. Hydraul. Eng. 146. doi: 10.1061/(ASCE)HY.1943-7900.0001684
Sayers, P., Li, Y. G., Galloway, E., Penning-Rowsell, F., Shen, K., Wen, Y., et al. (2013). Flood Risk Management: A Strategic Approach. Paris: UNESCO.
Tang, Y. B., Zhu, D. Z., Rajaratnam, N., and van Duin, B. (2020). Sediment depositions in a submerged storm sewer pipe. ASCE J. Environ. Eng. 146. doi: 10.1061/(ASCE)EE.1943-7870.0001799
Urban Drainage Flood Control District (2016). Urban Storm Drainage Criteria Manual: Volume 1 Management, Hydrology, and Hydraulics. Denver, CO. Available online at: https://www.mhfd.org/wp-content/uploads/uploads/vol1%20criteria%20manual/00_front%20docs%20and%20Preface_V1.pdf
Walesh, S. G. (1999). Street Storage System for Control of Combined Sewer Surcharge - Retrofitting Stormwater Storage Into Combined Sewer Systems. National Risk Management Research Laboratory, US EPA, Cincinnati, OH.
Watt, E. W. (Ed.). (1989). Hydrology of Floods in Canada: A Guide to Planning and Design. National Research Council of Canada, Ottawa, ON.
Keywords: stormwater management, resilience, risk, climate change, densification, dual drainage, natural system, low impact development
Citation: van Duin B, Zhu DZ, Zhang W, Muir RJ, Johnston C, Kipkie C and Rivard G (2021) Toward More Resilient Urban Stormwater Management Systems—Bridging the Gap From Theory to Implementation. Front. Water 3:671059. doi: 10.3389/frwa.2021.671059
Received: 22 February 2021; Accepted: 28 May 2021;
Published: 12 July 2021.
Edited by:
Hamidreza Shirkhani, National Research Council Canada (NRC-CNRC), CanadaReviewed by:
Sumit Purohit, Pacific Northwest National Laboratory (DOE), United StatesCopyright © 2021 van Duin, Zhu, Zhang, Muir, Johnston, Kipkie and Rivard. This is an open-access article distributed under the terms of the Creative Commons Attribution License (CC BY). The use, distribution or reproduction in other forums is permitted, provided the original author(s) and the copyright owner(s) are credited and that the original publication in this journal is cited, in accordance with accepted academic practice. No use, distribution or reproduction is permitted which does not comply with these terms.
*Correspondence: Bert van Duin, YmVydC52YW5kdWluQGNhbGdhcnkuY2E=
Disclaimer: All claims expressed in this article are solely those of the authors and do not necessarily represent those of their affiliated organizations, or those of the publisher, the editors and the reviewers. Any product that may be evaluated in this article or claim that may be made by its manufacturer is not guaranteed or endorsed by the publisher.
Research integrity at Frontiers
Learn more about the work of our research integrity team to safeguard the quality of each article we publish.