- 1Department of Biological Sciences, Idaho State University, Pocatello, ID, United States
- 2Department of Hydrology and Atmospheric Sciences, University of Arizona, Tucson, AZ, United States
Ephemeral and intermittent streams are increasing with climate and land use changes, and alteration in stream water presence or flow duration will likely affect litter decomposition and nutrient dynamics in channel and riparian zones more than uplands. To investigate the influence of varying climate and streamflow regimes on rates of decomposition and associated nutrient dynamics, we used a space-for-time substitution design in which we deployed a common leaf litter across a range of ephemeral to seasonally- intermittent stream reaches (10) and landscape positions (channel, riparian, upland) in Arizona, USA over an 18-month period. We also measured soil physio-chemical properties and nutrient dynamics associated with these reaches and positions. Consistent with expectations, rates of litter decomposition (k) decreased significantly in the channels as cumulative percentage (%) of water presence decreased below 40%. Indeed, differences in cumulative duration of water presence as well as channel bed material silt content explained 80% of the variation in k across flow regimes. In contrast, decay rates of the common litter across sites were surprisingly similar in upland and riparian positions despite large differences in climate, specifically precipitation (160–516 mm). Relatively similar litter nitrogen immobilization and soil moisture in upland and riparian environments helped to explain the lack of difference in k and soil nutrient dynamics in these environments. Collectively, our findings indicate that stream water presence may be a more important indicator of ephemeral and intermittent stream function than streamflow alone and that riparian zones in these dryland regions may be less responsive to changes in climate and associated subsidies of streamflow.
Introduction
Ephemeral and intermittent streamflow are the dominant flow regimes in dryland regions—hyper-arid, arid, semi-arid, and dry sub-humid areas that together encompass >40% of the terrestrial land surface (Reynolds et al., 2007). The occurrence of ephemeral and intermittent streamflow, flow only during and immediately after a rainfall event or flow for short duration, respectively, is likely to increase in distribution and extent with climate change (Seager et al., 2007; Larned et al., 2010; Döll and Schmied, 2012) as well as human water appropriation (Postel et al., 1996; Alley et al., 2002; Larned et al., 2010). In the Southwestern United States (US) (Arizona, New Mexico, Nevada, Utah, Colorado and California), for example, over 81% of streams are classified as ephemeral and/or intermittent (U.S. Geological Survey, 2008; Levick et al., 2008). By 2050, it is projected that regime shifts from perennial to intermittent and ephemeral streamflow will increase by 5.4–7.0% globally under low to high emissions climate change scenarios, mostly in semi-arid regions (Döll and Schmied, 2012).
Intermittent and ephemeral streams have been identified as providing key hydrologic functions and services; they supply water to plants, animals, and drinking water systems in otherwise dry landscapes (Levick et al., 2008). They store and exchange surface and subsurface water (Lane, 1983; Goodrich et al., 1997, 2004), provide hydrologic connectivity and continuity for habitat (Jaeger and Olden, 2012; Jaeger et al., 2014), and recharge and discharge groundwater (Scanlon and Goldsmith, 1997; Scanlon et al., 1999; Heilweil et al., 2004). Despite their broad geographic significance and hydrologic importance, however, key ecological functions in ephemeral and intermittent streams remain poorly understood and characterized (Stanley et al., 1997; Levick et al., 2008; Larned et al., 2010; Datry et al., 2011, 2014). In particular, little is known about how changes in the frequency and duration of streamflow or water presence associated with ephemeral and intermittent streams will alter organic matter and nutrient dynamics and their controls. The few studies conducted have been associated with one or two intermittent streams (Schade and Fisher, 1997; Anderson and Nelson, 2006; Langhans and Tockner, 2006; Sangiorgio et al., 2007; Corman et al., 2016) or lab incubations that show the importance of frequency and duration of wetting of litter and sediment in releasing of carbon dioxide (CO2) and nutrients (Gallo et al., 2014; Datry et al., 2018; Shumilova et al., 2019; Correa-Araneda et al., 2020). Direct measurements of streamflow duration, water presence, and decomposition rates across a strong climate gradient remain lacking. Moreover, linkages among uplands, floodplain or riparian zones and these temporary stream environments remain poorly characterized owing to the complexity and episodic nature of these interactions (Belnap et al., 2005; Welter et al., 2005).
Transitions from perennial to intermittent or ephemeral flow regimes in streams will introduce an ecologically significant terrestrial phase in the stream channel and the surrounding environment (Arce et al., 2019). In perennial streams, the chemical and physical properties of the organic matter (litter quality), detritivores present including invertebrates and fungal biofilms, stream temperature, and water column nutrients are considered to be the primary controls on decomposition (e.g., see Webster and Benfield, 1986; Tank et al., 2010). With transitions to intermittent and ephemeral flow, abiotic processes such as the frequency and duration of streamflow and soil drying and wetting cycles (Datry et al., 2018) as well as soil coverage and UV radiation may become more important factors controlling decomposition rates. These last factors are similar to those identified in the upland dryland literature (Austin and Vivanco, 2006; Throop and Archer, 2007; Barnes et al., 2012, 2015). Thus, it is likely that the relative importance of abiotic and biotic controls on decomposition and nutrient dynamics will teeter back and forth with changes in the terrestrial and aquatic phases associated with different flow regimes in intermittent to ephemeral streams.
Here we examine litter decomposition rates and nutrient dynamics in 10 ephemeral-to- seasonally-intermittent streams in Arizona, USA over an 18-month period. We used a space-for-time substitution and held litter quality constant across sites by deploying oak (Quercus grisea) leaf litterbags, sourced from the wettest site, to investigate the influence of varying climate and associated streamflow conditions on decomposition and nutrient dynamics. At each study site, we deployed litterbags along three cross-sectional transects in upland, riparian, and channel positions to evaluate the role of landscape position in determining decomposition and nutrient dynamics. We also characterized the soil physical and biogeochemical characteristics of the sites, monitored streamflow and water presence using electrical resistance (ER) sensors, and measured seasonal soil moisture and nutrient dynamics across sites and positions to evaluate possible controls on litter decomposition. We hypothesized that decomposition would be more rapid in channels with seasonally-intermittent flow compared to ephemeral flow, and expected that abiotic factors such as longer duration of streamflow and water presence and associated soil moisture would have a stronger influence on rates of decomposition than biotic processes such as nutrient availability and cycling rates. We also expected rates of decomposition to be more rapid in channels and riparian zones compared to upland environments, and differences in climate and subsidies of streamflow and/or soil-water moisture to control organic and nutrient release.
Materials and Methods
Study Sites and Design
All study sites were located on military installations or near them as a part of a program to understand and manage ephemeral and intermittent streams on military lands. We initially selected 13 temporary stream channels or washes in southern Arizona (Figure 1) that spanned a climate gradient, with mean annual precipitation ranging from 160 to 750 mm, and a range of geomorphic conditions. Precipitation across the region is bimodal: summertime convective rainfall (the North American Monsoon) is intense, of short duration, spatially heterogeneous and lasts from mid-late June to mid-late September. A second precipitation period is observed primarily between December and March with rainfall of lower intensity and longer duration arising from widespread storm systems. The most arid study sites were located within the Barry M. Goldwater Air Force Base near Gila Bend, Arizona in the Lower Gila River Basin (Sauceda Wash, SW; and Black Gap, BG; Figure 1B), on semi-consolidated alluvial basin fans (200–300 m elevation). The catchment upstream of the BG reach is 10.2 km2 and ranges in elevation from 324 to 676. The catchment upstream of the SW reach is the largest of our study sites, 326.4 km2, and ranges in elevation from 258 to 1114 m. The 30-year mean annual precipitation (MAP30) at these sites ranges between 160 mm at the lower elevations and 250 mm at the higher elevations. Mean annual temperature (MAT) is 23°C and can range between 5°C to 43°C (US Climate Data; http://www.ncdc.noaa.gov/).
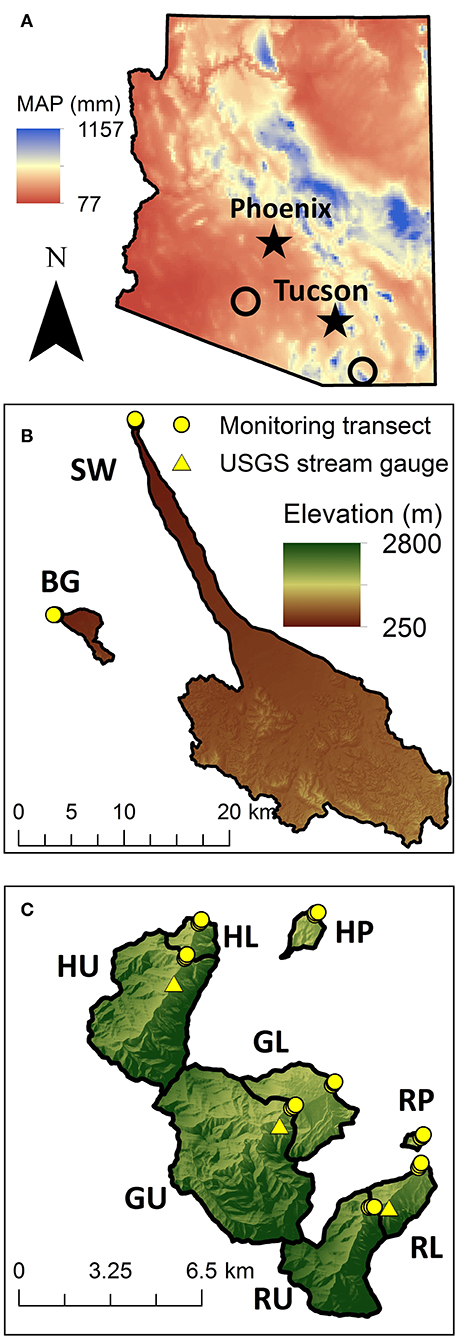
Figure 1. (A) Location of two main study areas in Arizona along a climate gradient with black open circles showing locations of Barry Goldwater and Fort Huachuca military installations. (B) Black Gap (BG) and Sauceda Wash (SW) are located in Barry Goldwater military installation. (C) Huachuca Piedmont (HP), Huachuca Lower Canyon (HL), and Huachuca Upper Canyon (GU) as well as Garden Piedmont (GP), Garden Lower (GL), and Garden Upper (GU) Canyon are located in the Fort Huachuca military installation. Ramsey Piedmont, Lower (RL), and Upper Ramsey (RU) Canyon are located in the Nature Preserve.
Two semi-arid study washes were located on the Santa Rita Experimental Range near Sahuarita, Arizona in the Santa Cruz River Basin (SS and SR). These were center-of-basin braided unconsolidated sandy channels at 947 and 952 m in elevation. The catchment upstream of SS was 1.7 km2 and ranged in elevation from 947 to 1,105 m, while the catchment upstream of SR was 18.0 km2 and ranged in elevation from 952 to 1,748 m. The MAP30 ranges between 350 mm at the lower elevations and 570 mm at the higher elevations (PRISM Climate Group, 2013); while MAT is 22°C and can range between 6 and 38°C. These sites were later dropped from litter decomposition and nutrient analyses because these sites were established later and hence timing of litter deployment was offset from the rest of the sites, although soil and nutrient data are available in Lohse et al. (2020b). These two sites will not be discussed hereafter.
Nine other study sites were located on the Huachuca Mountains at the Fort Huachuca Army Post and Nature Conservancy Ramsey Canyon Preserve near Sierra Vista, Arizona; these catchments drain into the San Pedro River Basin. The study sites were located in three canyons at three distinct elevations in each canyon: Huachuca Canyon (H), Garden Canyons (G), and Ramsey Canyon (R; Figure 1C). The lowest elevation monitoring sites (HP, RP, GP) located at 1,400–1,500 m elevation are piedmont (P), semi-consolidated alluvial channels, and receive 430 to 580 mm MAP. The HP, GP, and RP catchments are the smallest (1.3, 0.5, and 0.3 km2, respectively), and have stream channel densities similar to those of the alluvial basin sites with values ranging between 2.4 and 6.3 km km−2. GP site was later dropped from the analysis after a fire swept through and burned the study area and litterbags, reducing the total site number to ten. Three lower (L) canyon sites (HL, GL, RL; Figure 1C), located at 1,500–1,600 m elevation, are incised streams with non-cohesive alluvial banks; MAP30 ranges between 520 and 650 mm. Finally, three upper (U) canyon sites (HU, GU, RU; Figure 1C) are located at 1,600–1,700 m elevation on cohesive bedrock with moderate alluvium present. These upper sites have MAP30 ranging from 590 to 750 mm and temperature ranges from 1 to 34°C, with MAT of 17°C.
At each of the study sites, we selected a monitoring stream reach of 200 m in length (Figure 2). The catchment upstream of each monitoring stream reach was delineated and characterized using ArcMap 10.2 (ESRI, Redlands, CA). At each reach, we established three cross-sectional transects 100 m apart (0, 100, 200 m) and characterized the channel geometry (Gallo et al., 2020). We established sampling locations at channel, riparian, and upland positions along each of the three transects for measurement of site physical and chemical characteristics, litter decomposition and soil moisture and nutrient dynamics.
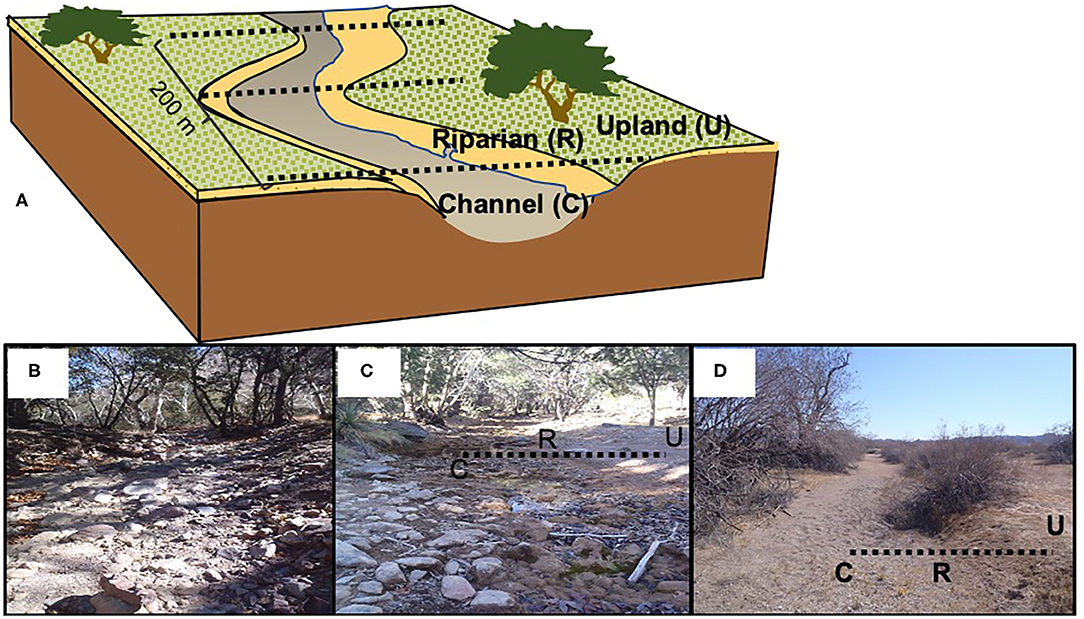
Figure 2. (A) Study site design with location of monitoring stream transects and associated channel, riparian, and upland position. Examples of channel, riparian and upland positions at (B) HU, Huachuca Upper Canyon; (C) GL, Garden Lower; and (D) BG, Barry Goldwater.
Site Physical and Chemical Characteristics
The physical and chemical properties of the <2 mm soils or sediments at all positions were characterized (n = 99 total). In brief, we cored soils (0–5 cm depth) in the field, brought them back to the lab, air dried and passed them through a 2 mm sieve. We determined particle size distributions (% sand, % silt, and % clay) and soil texture using a modified pipette method (Gee and Bauder, 1986). We determined water holding capacity (WHC) following methods by Dane et al. (2002). We made a 2:1 water to soil paste and determined soil pH with a probe calibrated using pH 10.01, 7.00, and 4.01 buffer solutions (Thomas, 1996). We measured soil C and N and isotopes of C and N on an Elemental Combustion System 4010 (Costech Analytical Tech, Inc, USA) attached to a Delta V Advantage Mass Spectrometer (Thermo Scientific, Germany) at the Center for Archaeology, Materials, and Applied Spectroscopy at Idaho State University, Pocatello, Idaho. In brief, we dried soils at 55°C for 24–48 h prior to grinding soil. We placed a 20 g soil subsample, which had been picked for roots, into a ball mill grinder and ground the soils until a fine powder was attained. We removed soil inorganic carbon by acid fumigation prior to analysis and then packed samples into 5 × 9 mm tin capsules. We report values for δ15N in parts per thousand (%0) relative to atmospheric N2 using the equation: δ (%0) = [(Rsample/Rstd) - 1] × 1,000 where Rsample = - ratio heavy to light isotope (15N/14N) of a sample. We are report values for δ13C in parts per thousand (%0) relative to the Vienna PeeDee Belemnite standard. Both δ15N and δ13C values had an analytical precision of ≤ 0.2 that was determined from repeated analyses of standard materials. We also collected a separate set of soils from all sites and locations to determine soil bulk density (g cm−3) using a modified version of the excavation method (Grossman and Reinsch, 2002; Gallo et al., 2014). In brief, we excavated the soil mass of an 8 cm diameter circle to 10 cm depth with a trowel. We determined the volume of the void by lining it with a plastic bag and filling it with water. We sieved the soils (<2 mm) collected from this void, dried them for soil moisture, and then weighed them for mass to determine the fraction of fine earth <2 mm. Particle density was assumed to be 2.65 g/cm3. Fine and coarse fractions were determined from bulk density sampling.
Precipitation and Streamflow and Water Presence
To classify site climatic and flow conditions, we measured precipitation using the nearest, established tipping bucket rainfall gauges, streamflow presence using an electrical resistance (ER) sensor method described by Jaeger and Olden (2012) and determined soil-water presence using an ER sensor method by Gallo et al. (2020). In brief, we installed electrical resistance sensors (TidbiT v2 UTBI-001 data logger, Onset Corporation, Bourne, MA) at the thalweg of each cross-sectional transect to identify surface water presence frequency and duration. The ER sensors were modified as outlined in Blasch et al. (2002), with 2 electrical leads exposed to identify the onset and cessation of runoff, where the onset of runoff was marked by the sudden and rapid increase in the relative electrical conductivity (EC) signal to a less negative or a positive number. The termination of streamflow was marked by a similarly sudden shift in the EC signal back to a more negative signal. In contrast to the streamflow presence signal, the surface water presence signal had a distinct inflection point that marked a shift from a steep EC signal recession to a less steep EC signal recession. Because it was difficult to distinguish EC signals indicating water ponding versus soil moisture presence, we defined all EC signals indicative of water presence, including streamflow, as “water presence.” Given that we were interested in identifying maximum length of time that water might be present in the stream either as ponded water, shallow soil water or streamflow available for biological activity, we used the maximum percent (%) water presencetransect observed at each monitoring reach as the reach % water presence. We identified five distinct streamflow regime classes: dry-ephemeral, wet-ephemeral, dry-intermittent, wet-intermittent, and seasonally-intermittent groups (Gallo et al., 2020). Here we report cumulative precipitation, and monthly and cumulative streamflow and water-presence associated with date of litterbag collection; data are available in Lohse et al. (2020a).
Litter Decomposition
To test the influence of climatic and flow variables on decomposition, litter quality and quantity was held constant across sites. Given that streams are transitioning from wetter to drier flow regimes, we collected litter at the wettest site, upper Ramsey Canyon (RU) and deployed them at each site across all positions. At RU, we collected senesced oak (Quercus grisea) leaves as oak woodlands with conifers dominate the upper canyons in this region (Stromberg et al., 2015; Corman et al., 2016); we also collected a smaller subset of senesced Arizona sycamore (Platanus wrightii) leaves. Litter was collected in separate plastic bags, brought back to the lab, and dried at 40°C to obtain a constant water weight prior to packing. We note that mean average summer temperatures ranged from 32–42°C across sites so that drying at 40°C was not outside of the extremes. Nylon mesh litterbags (10 × 15 cm, 1 mm nylon mesh) were packed with 4 g dried leaf material. Every 35th bag was packed and then transferred to a pre-dried envelope for wet-dry correction and initial chemical analysis where samples were dried to 55°C. Initial carbon (C) and nitrogen (N) contents were determined on a Fisons NA-1500 elemental analyzer (Fison Instruments, Milan, Italy). Initial litter C:N-values for oak were 32.2 ± 0.55 and for sycamore 85.7 ± 9.4 (mean ± standard error (SE).
On 10 January 2010, we placed nine randomly selected oak litterbags in each position x transect x site combination (upland, riparian, and channel positions, 3 transects, 10 sites for 810 total). Only one replicate per site for sycamore was deployed due to limited litter material (nine litter bags per landscape position, 27 per site, 288 total). We do not focus on this litter type hereafter except to note that decomposition rates of sycamore were slightly lower than oak but did not differ significantly within the channels (Stromberg et al., 2015). All litterbags were collected at time 0, 1 day, 4 weeks, 3, 6, 12, and 18 months. Following collection from the field, litter was cleaned to remove residual soil, dried at 55°C for 12–24 h and then weighed. Litter samples were ground to a fine powder (pass through no. 40 sieve) and stored in vials. Ash free dry mass (AFDM) was determined on 1.0 g of homogenized subsample by combusting it at 450°C for four hours and reweighing the mass after combustion. Another subsample of the ground litter was analyzed for C and N content by packing 6.0 mg of ground litter into tin capsules and analyzing it on the elemental analyzer. Oak and sycamore litter data are available in Lohse et al. (2020a).
Soil Moisture and Nutrient Dynamics
To evaluate possible moisture and biological controls on rates of decomposition, we monitored seasonal soil moisture and soil exchangeable mineral N pools and transformation rates using both soil extractions and incubations, wet up experiments, as well as in-situ ion exchange resins bags. Specifically, surface soils from each position were collected from 0–5 cm depth during winter/spring (January–March), pre-monsoon (May–June) and post-monsoon (August–September) seasons from June 2010 to September 2012, periods that maximized thermal and hydrologic contrasts (99 samples per season). Soils were collected with a core sampler, stored in plastic bags in coolers, returned to the laboratory, and subsampled for analyses. In the lab, we sieved the soils to <2 mm, and then dried one 25 g soil subsample at 105°C to determine gravimetric soil moisture. To determine exchangeable mineral N pools, we extracted a 10 g subsample with 50 ml of 2N potassium chloride (KCl), ammonium () and nitrate (). To determine rates of net mineralization and nitrification, we incubated another soil subsample under aerobic conditions for 7 days in the dark and estimated rates based on difference between initial and 7-day exchangeable mineral N pools, plus , and only, respectively (Hart et al., 1994). Finally, we performed wet-up experiments on pre-monsoon dry soils to evaluate the potential rates of nutrient release after monsoon wetting. In brief, we wet soils with 18.2 mOhm water to 60 % WHC moisture conditions, incubated these soils for 7 days in the dark, extracted them, and analyzed them for exchangeable mineral N pools.
To assess in-situ nutrient availability and release in response to seasonal dynamics, we utilized exchange resin bags, which have been shown to be a useful integrator of chemical flux over time (Binkley and Matson, 1983). We deployed two sets of exchange resin bags, one for cations (Dowex 50W−8X; H+form) and one for anions (Dowex 1–8X; C1− form). Bags (6 × 5 cm) were constructed on inert silk screen mesh-monopolyester, #85 and filled with 3.0 +/– 0.05 g resin and charged overnight in 2M sodium chloride (NaCl) for anion and 2 M Hydrochloric acid (HCl) for cation bags. One cation and anion exchange resin bag each was placed in the soil at a depth of 3–5-cm along the three transects at each site at the three landscape positions. Resin bags were deployed in upland, riparian and channel positions during the seasonal periods, winter/spring (January–March), pre-monsoon (May–June) and monsoon/post-monsoon (August–September) to contrast thermal and hydrologic contrasts as above. Bags were retrieved at the end of each period, and new bags of both cation and anion exchange resins were deployed in the same positions. In the lab, resin bags were washed free of soil using 18.2 mOhm water. The resins were then placed in centrifuge tubes for extraction in 0.5 M NaCl (40 ml) for anions and 2 M NaCl in 0.1 M HCl for cations. Extracts for and in associated matrices (KCl, NaCl, NaCl in HCl) were analyzed on a SmartChem discrete autospectrophotometer (DA) (Unity Scientific Instruments, Inc., Brookfield, CT) at Idaho State University. We used a salicylate method (AMM-003-A) to analyze for as N (hereafter referred to as ) and a nitrate reduction method with a cadmium metal column (NO3-001-A) to analyze for as N (hereafter referred to as ). We also report resin and soil soluble reactive phosphorus data in Lohse et al. (2020b).
Statistical Analyses
Statistical analyses were performed in JMP 11 (SAS, Carey, NJ). Changes in mass remaining, where litter AFDM at time (t) (Mt) is divided by the initial AFDM (M0) (Mt/M0), litter N remaining as percent, soil moisture, and nutrient dynamics were analyzed using repeated measures ANOVA. Loss of litterbags downstream in several channel positions (HU, GU) resulted in an unbalanced design and precluded statistical comparisons among sites and locations on 18-month time periods so that analyses for litter were performed up to 12 months. Flow regime (F) and landscape position (P) were considered main effects with interaction terms. Welch's ANOVA were used for main effects tests where variances were unequal. Post-hoc Tukey tests were performed to determine significant differences among flow regimes. Decay constants (k) were generated from regressions where the natural log transformation of Mt/M0 was plotted against time since deployment (up to 18 months). Step-wise multiple linear regression models were performed to evaluate possible climate, streamflow condition, and nutrient availability controls on the decay rates (k, d−1), in the absence of variation in litter quality and quantity as a common litter from the wettest site (RU) was deployed across sites. Transformations were performed to meet assumptions of normality and homoscedascity.
Results
Soil Physical and Chemical Characteristics
Soil physio-chemical characteristics varied significantly with flow regimes and positions (Table 1). Soil bulk density (<2 mm fraction) was significantly higher in the dry-ephemeral washes (1.99 ± 0.15 g/cm3) compared to other positions and flow regimes (range: 0.85–1.64 g/cm3) (p < 0.05). Average sand content was high across sites, ranging from 70 to 94% whereas silt and clay made up a smaller and more variable percentage, 2–26% silt and 1.5–8.7% clay. Percent soil organic carbon (C) was significantly lower in channels than in other positions, and tended to be more similar in riparian and upland positions. Across flow regimes, soil C was lowest in the dry-ephemeral channels and increased substantially in the wet-intermittent to seasonally-perennial channels, 0.3–6.3%. Sediment nitrogen (N) was very low in most of the channel positions (0.03–0.07%) except in the seasonally-perennial channels, where % N was higher, 0.37 %. The upland and riparian soil carbon to nitrogen ratios (C:N) were generally higher than channel sediments, particularly under dry and wet-intermittent conditions where ratios were >40.
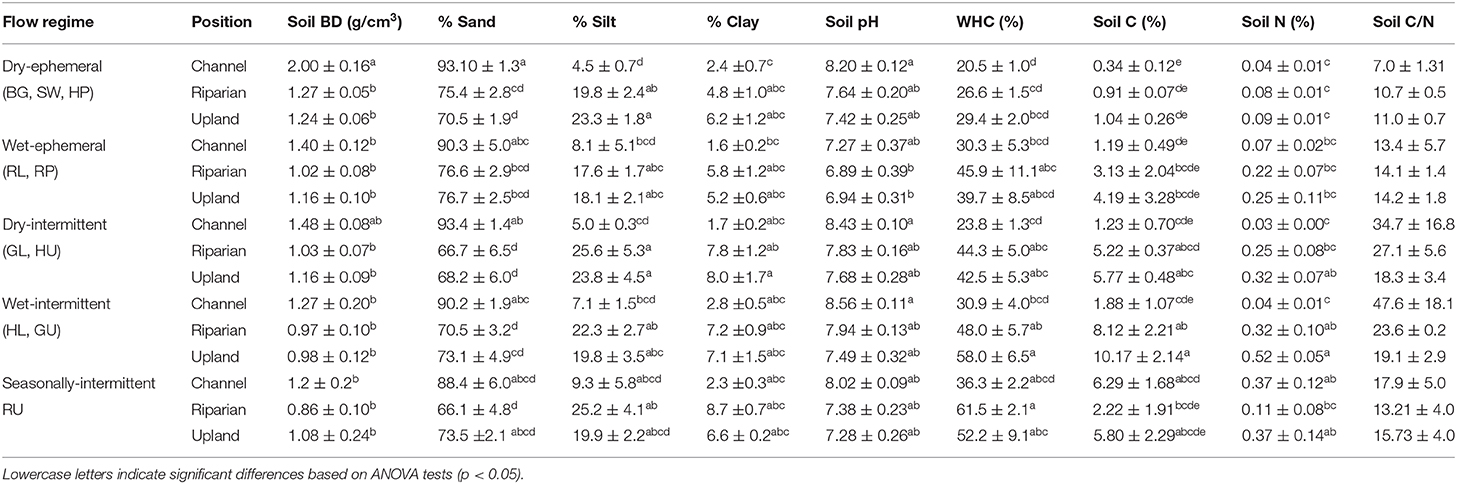
Table 1. Soil physiochemical characteristics including soil bulk density (BD) (<2 mm), percent (%) sand, silt, and clay %, water holding capacity (WHC) as %, and soil carbon (C) and nitrogen (N) %, and C/N of channel, riparian, and upland positions across flow regimes.
Precipitation and Streamflow and Water Presence
Precipitation was bimodal across all sites with higher precipitation with cumulative precipitation ranging from 111 mm at the dry-ephemeral to 516 mm at seasonally-intermittent site over the 18-month (January 2011–June 2012) litter deployment [Supplementary Figure 1; also see Gallo et al. (2020) for mean monthly distribution]. All but one group, the seasonally-intermittent flow regime, exhibited a bimodal streamflow similar to that of the regional rainfall (Figures 3A,B). Cumulative streamflow days varied dramatically from <1 to 434 days across the sites over the 18-month deployment, representing 0.2–85% of available days (Figure 3C). Cumulative daily stream water presence varied from 11 to 433 days, 2.5–85.5 % as annual water presence (Figure 3D). The most responsive sites to rainfall in terms of soil-water presence were the dry- and wet-ephemeral sites and had higher observed water presence relative to streamflow compared to the other sites (Figure 3D).
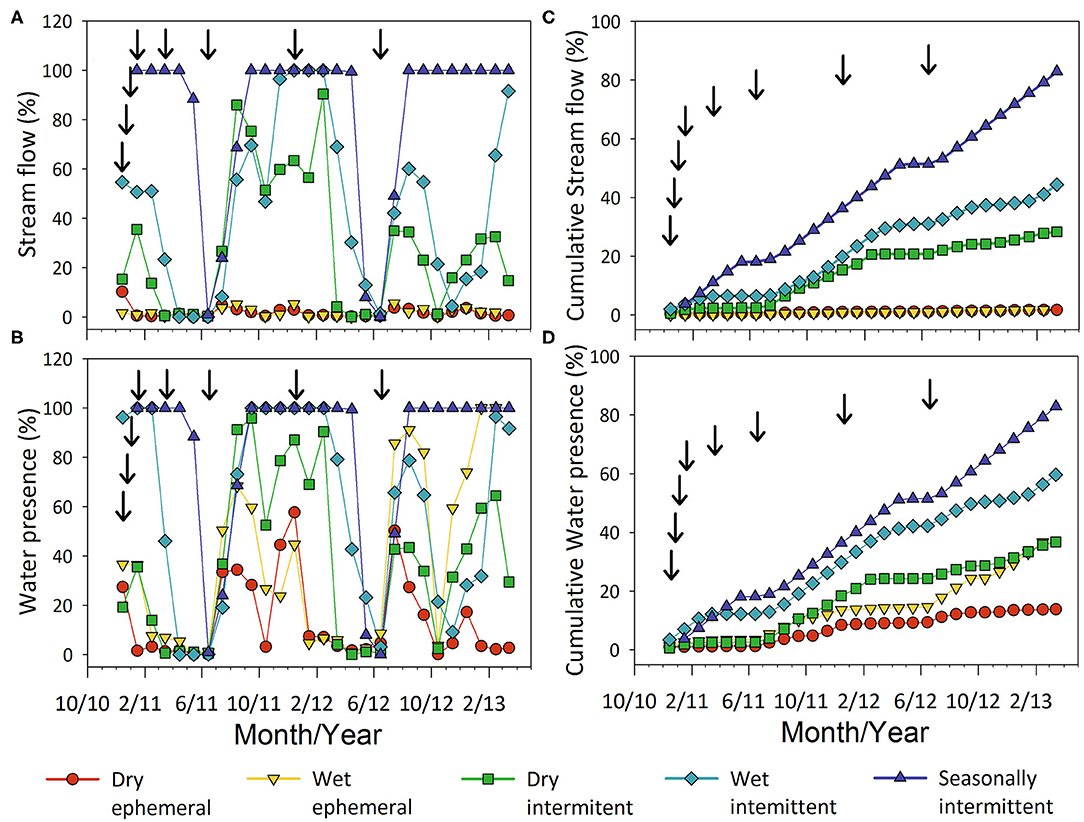
Figure 3. Monthly percent of sites with streamflow and stream water presence at dry-ephemeral (BG, SW, HP, GP), wetephemeral (RP, RL), dry-intermittent (HU, GL), wet-intermittent (HL, GU), seasonally-intermittent (RU) locations. Mean monthly values for each flow regime category are shown in (A,B). Arrows indicate litterbag collections. Cumulative streamflow and water presence percentages as a fraction of the total days over a monitoring periods are show in (C,D).
Decomposition Dynamics
Litter mass of oak decreased significantly with time across all sites, with landscape position exerting a significant influence on rates of decomposition across sites (Figure 3; RMANOVA: flow regime: F4, 75 = 9.28, p < 0.0001; position: F2, 75 74.45, p < 0.0001; flow regime*position: F8, 75 9.96, p < 0.0001; time: F3, 73 182.28; time*flow regime: F12, 193 3.85, p < 0.0001; time*position F6, 146 21.17, p < 0.0001 time*position*flow regime; F24, 212 = 3.82, p < 0.0001). Oak litter in upland and riparian positions had >50 % of mass remaining as ash free dry mass at the end of the 18th month deployment and did not differ significantly across sites and flow regimes (Figures 4A,B). In contrast, mass loss in the channels differed significantly across sites and flow regimes (Figure 4C). Indeed, these differences among flow regime in channels emerged as early as 4 weeks after deployment based on univariate ANOVA and post-hoc Tukey tests (Figure 4C). In seasonally- and wet-intermittent channels, <20 % of the oak litter mass remained after 18 months compared to other flow regimes that had >50 % remaining, percentages similar to those observed in the upland and riparian positions across sites (Figure 4C).
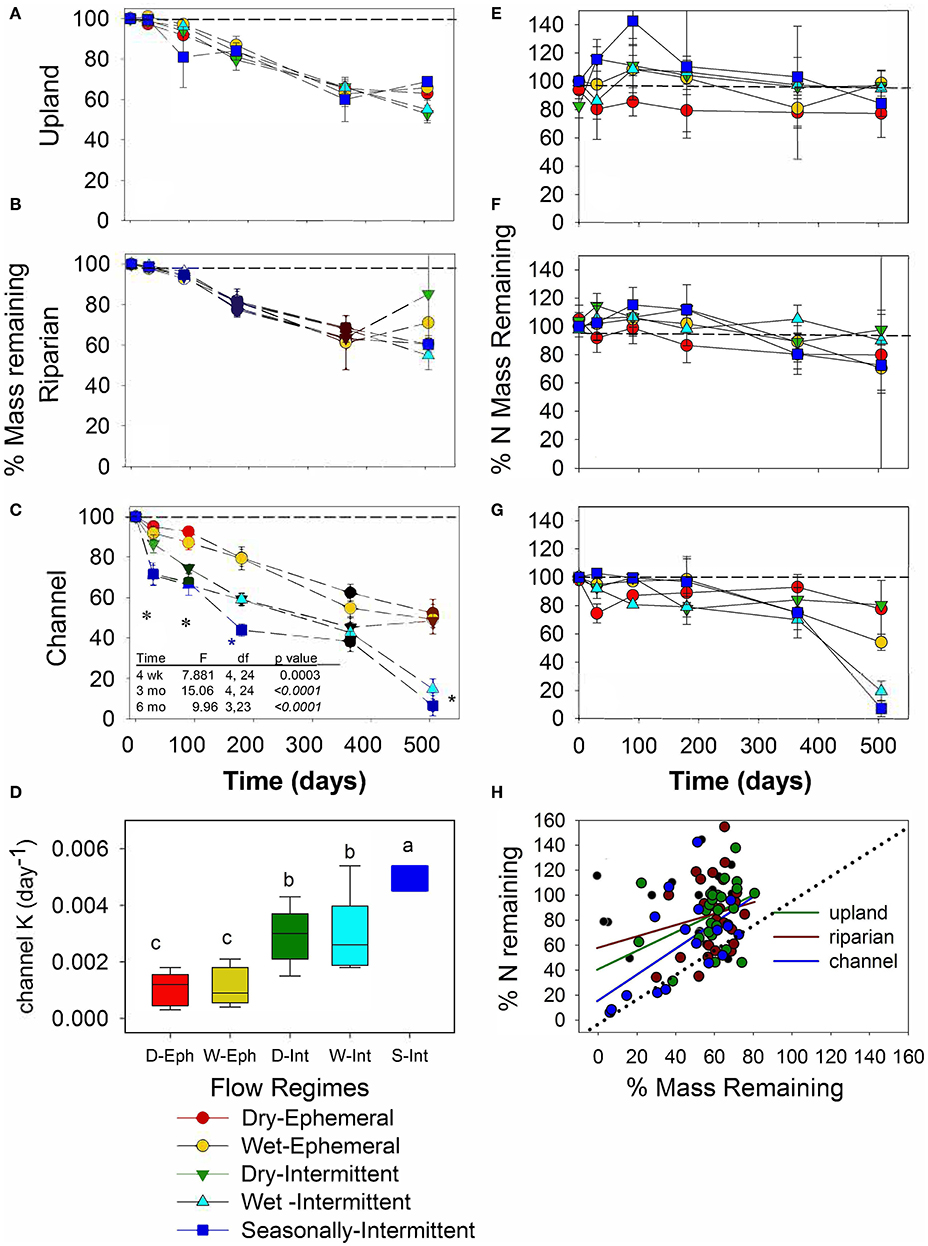
Figure 4. Mass remaining as percent (%) of initial oak litter mass over time (days since deployment) for 10 study sites in the (A) upland, (B) riparian and (C) channel positions, and (D) oak leaf decay (k) rates shown for the channel position by flow regime (D-Eph, Dry-ephemeral; W-Eph, Wet-Ephemeral; D-Int, Dry-intermittent; W-Int, Wet-Intermittent; and S-Int, Seasonally-Intermittent). Asterisks in (C) indicate significant differences in mass loss among sites at different time points as tested with ANOVA and post-hoc Tukey tests (p < 0.05). Different letters in (D) indicate significant differences in decay rate among flow regimes (p < 0.05). Mass is expressed in ash free dry mass. Percent N mass remaining of initial oak litter N over time (day since deployment) for the different flow regimes in the (E) upland, (F) riparian, and (G) channel positions. (H) Relationship between %N remaining and % mass remaining shows that upland and riparian locations showed net gain while N loss in channel positions was proportional to mass loss.
A single exponential decay model fit the oak litter data well (mean r2 0.9, median 0.94). Oak leaf decay rates (k) ranged from 0.0003 to 0.0054 d−1, and ANOVA and post-hoc Tukey tests conducted on channel locations showed that decay rates were significantly higher in channels with seasonally-intermittent compared to dry- and wet-intermittent streamflow and significantly higher than those with ephemeral flow (Welch's ANOVA F4, 9.45 38.17, p < 0.0001; Figure 4D).
Oak litter N dynamics as measured by percent N remaining varied significantly with flow regime and interactions with time and generally showed net gains of N mass in upland and riparian positions whereas litter in channel positions showed net losses of N mass (Figure 4) (RMANOVA: flow regime: F5, 78 = 3.79, p = 0.004; position; F2, 78 = 2.05, p = 0.1344, flow regime*position F10, 78 = 0.56, p = 0.84; time: F3, 76 = 2.49, p = 0.06; time*flow regime; F15, 210 = 2.1, p = 0.01). Specifically, oak litter % N mass remaining increased as much as 20–40% from the initial value of 1.5 ± 0.04% N in the riparian and upland positions, respectively, especially at the wetter sites (Figures 4E,F). The exception was the dry-ephemeral sites that showed declines in litter N in the uplands and to a lesser extent, riparian positions. In the channels, the % oak N remaining generally decreased across all flow regimes (Figure 4G).
Over the 18-month study period, correlations between % mass remaining and % N remaining differed across landscape positions and were highly variable especially in upland and riparian zones. Litter in upland and riparian positions lost proportionally less N than total mass and yielded weak relationships with slopes less than 1, 0.45 ± 0.35 for the upland and 0.74 ± 0.56 for the riparian position. In contrast, % N remaining was proportional to mass loss in the channel (Figure 4H), with a slope of 1.03 ± 0.37.
Environmental Controls on Decomposition in Channels
Given no significant differences in k in riparian and upland positions, a stepwise multiple linear regression was conducted to elucidate environmental controls on the k in channels across flow regimes, and only significant factors with p < 0.05 were retained in the model. Results showed that cumulative days of water presence and % silt of the channel bed material explained 80% of the variation in the k in channels across flow regimes (Whole model, F2, 16 28.39, r2 = 0.80, = 0.77, RMSE = 0.0007, p < 0.0001; Figure 5A). Log transformed cumulative days of streamflow and water presence days were highly correlated (r = 0.95; Figure 5B) and the correlation indicated that these terms could possibly be used interchangeably. However, there were more days of water presence compared to streamflow detected at the dry end of the spectrum of flow regimes (i.e., DE regime), a range of 86 compared to 8.75 days, and therefore cumulative days of water presence was selected as a potentially more sensitive measure of water availability in these systems. Cumulative days of water presence was more normal distributed and thus reduced heteroscedascity in the multiple regression. Cumulative days of water presence alone explained 74% of the variation in decay rates (Figure 5C). Cumulative days of stream water presence and streamflow were also modestly correlated to cumulative precipitation (r = 0.62–0.78), but cumulative precipitation did not load in the model. Other biophysical variables considered such as % N remaining, pH, water holding capacity, litter % N, C:N, soil C and N, and soil C:N did not load into the stepwise regression model as significant factors. Lignin and other litter quality characteristics were held constant across all sites because of the common litter source and therefore not considered. Finally, soil temperature was not considered as a main factor in the regression models because site/position specific data were not available.
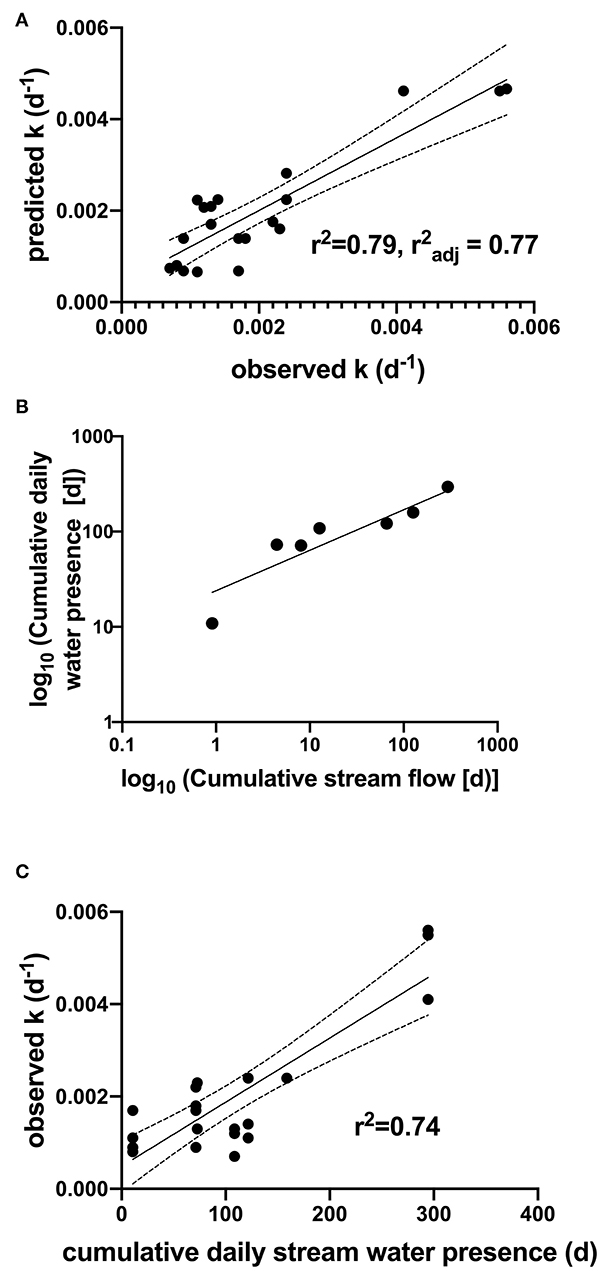
Figure 5. (A) Cumulative daily stream water presence and % silt explain 79% of variation in decomposition rates (d−1) in channels across flow regimes, (B) Log transformed cumulative daily stream presence is highly correlated to log transformed cumulative stream water flow days but detects water availability at lower flow conditions, (C) cumulative daily stream presence explain 74% of total variation in decomposition rates (k).
Soil Moisture and Nutrient Dynamics
Soil moisture dynamics followed stream and soil water presence patterns and varied significantly with flow regime, position, and season (RMANOVA: flow regime: F4, 89 = 35.52, p < 0.0001; position: F2, 84 26.92, p < 0.0001; flow regime*position: F8, 89 8.21, p < 0.0001; time: F5, 85 37.89, p < 0.0001; time*flow regime: F20, 282 6.11, p < 0.0001; time*position F10, 170 2.34, p = 0.003; time*position*flow regime; F40, 373 = 3.73, p < 0.0001; Figures 6A–C). In-situ gravimetric soil moisture was 2–6 times higher in the seasonally-intermittent compared to the dry-intermittent to ephemeral stream channels (Figure 6A). Soil moisture dynamics were more muted and less variable in the riparian and upland positions but peaked following the monsoon season, especially under seasonally-intermittent and wet-intermittent flow conditions (Figures 6B,C).
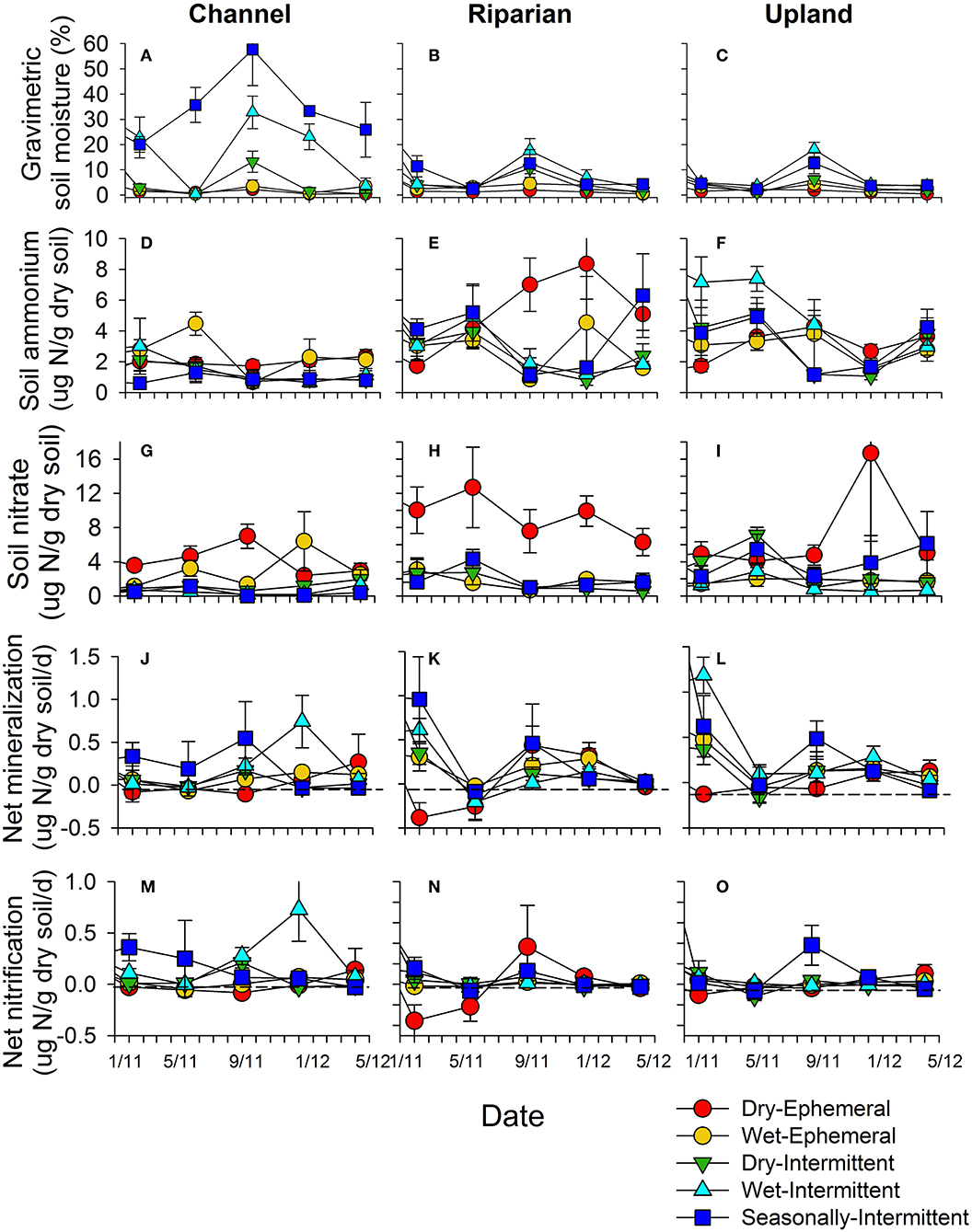
Figure 6. Soil moisture (A–C), ammonium (D–F), and nitrate (G–I) pools, and in-situ net mineralization (J–L) and net nitrification rates (M–O) associated with different flow regimes and positions (channel, riparian, and upland) over the course of the decomposition experiment.
Soil nitrogen pools and processes also varied significantly with flow regime, position, and season. Soil exchangeable concentrations varied significantly with time and interactions with flow regime and position (RMANOVA: flow regime: F4, 88 = 0.71, p = 0.587; position: F2, 88 14.59, p < 0.0001; flow regime*position: F8, 88 2.425, p = 0.02; time: F5, 84 11.09, p < 0.0001; time*flow regime: F20, 279 4.05, p < 0.0001; time*position F10, 168 2.79, p = 0.003; time*position*flow regime; F40, 368 = 1.44, p = 0.04; Figures 6D–F). Post-hoc ANOVA tests showed that upland and riparian positions were significantly elevated in compared to channel positions (Welch test: F2, 428 48.75 p < 0.0001). In contrast, only flow regime had a significant main effect on soil exchangeable concentrations (RMANOVA: flow regime: F4, 89 = 7.76, p < 0.0001; position: F2, 89 1.08, p = 0.34; flow regime*position: F8, 89 0.87, p = 0.54; time: F5, 85 1.38, p = 0.23). In particular, soil exchangeable was consistently and significantly elevated (4–8 times higher) under dry-ephemeral stream conditions relative to other flow regimes (Welch F4, 254 21.36, p < 0.0001; Figures 6G–I).
Rates of net mineralization and nitrification were much more variable across space and time, though they were generally positive under seasonally-intermittent flow conditions relative to others indicating nutrient release rather than immobilization (Figures 6J–L). In contrast to these field dynamics, controlled laboratory wetting experiments of pre-monsoon soils showed more consistent patterns in rates of N cycling. Indeed, experimental wetting of pre-monsoon soils resulted in 2–5 times higher potential rates of net mineralization and nitrification than pre-monsoon rates across flow regimes (Figure 7). The exception to this pattern was the seasonally-intermittent channel sites where rates of potential net mineralization and nitrification were comparable to pre-monsoon conditions (Figures 6, 7).
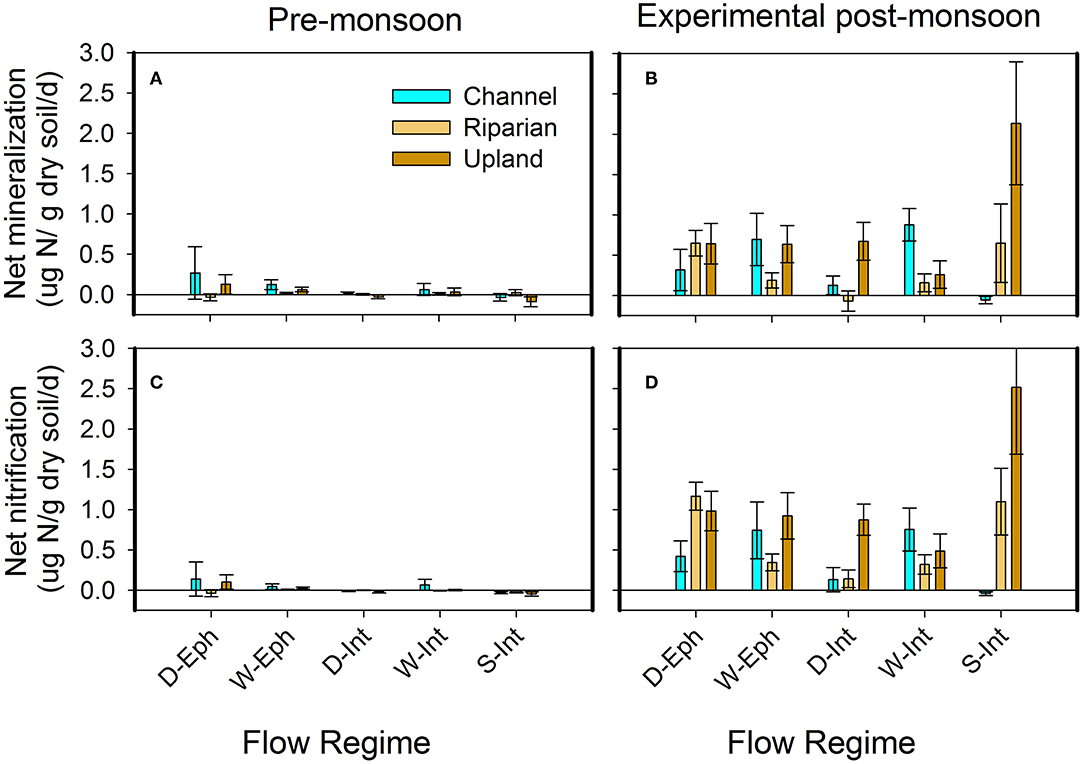
Figure 7. Pre-monsoon rates of net mineralization (A), potential rates of net mineralization in response to experimental wetting (B), pre-monsoon rates of net nitrification (C), and potential rates of net nitrification in response to experimental wetting (D) across flow regimes, D-Eph, Dry ephemeral; W-Eph, Wet-ephemeral; D-Int, Dry-intermittent; W-Int, Wet-Intermittent; and S-Int, Seasonally-Intermittent and positions.
Nutrient availability and potential loss as measured by resin bags during the monsoon season showed similar patterns as wet up experiments (Figure 8). Resin and concentrations were similarly high across flow regimes in upland and riparian positions following monsoon events. Similar to field data (Figure 6), resin was higher in the channel positions in the dry ephemeral sites compared to the wet intermittent and seasonally intermittent sites. Notably, channel sediments in the wetter sites were low in nutrient availability (Figure 8).
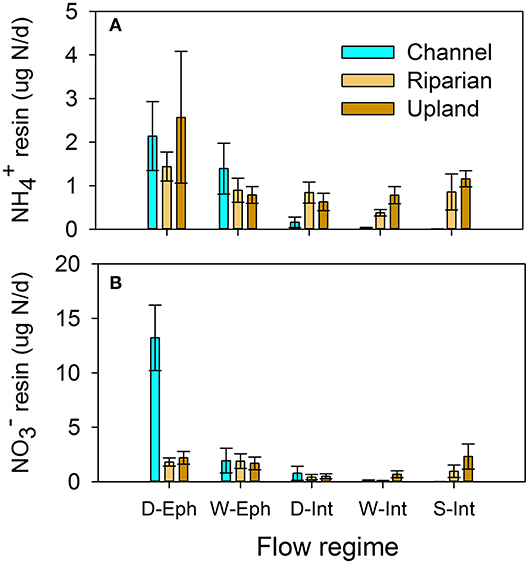
Figure 8. (A) Ammonium and (B) nitrate availability as measured by resin bags and normalized by total days deployed during the monsoon across flow regimes, Dry ephemeral (D-Eph), Wet-ephemeral (W-Eph), Dry-intermittent (D-Int), Wet-Intermittent (W-Int), and Seasonally-Intermittent (S-Int), and positions (channel, riparian, and upland).
Discussion
Higher decomposition rates observed in the seasonally- and wet-intermittent channels were consistent with expectations that mass loss would be higher under more permanent flow regime conditions compared to more temporary flow conditions. Rates of decomposition decreased by a factor of 3–6 with the transition from seasonally- and wet-intermittent to dry-intermittent and ephemeral flow regimes (Figure 4D). The few decomposition studies conducted in intermittent or drought-influenced streams have typically focused on one stream and shown similar decreases in rates of decomposition (Anderson and Nelson, 2006; Langhans and Tockner, 2006; Sangiorgio et al., 2007). Sangiorgio et al. (2007), for example, conducted their study in Mediterranean stream channels experiencing drought conditions and showed lower rates of leaf decay in dry compared to wet sites (0.003 compared to 0.006 d−1), rates which are comparable to those reported in this study (range: 0.001 to 0.0054 d−1). Results from our study contrasts with two studies focused in Arizona, USA on short-term decomposition rates of different litter in the aquatic phase of intermittent streams. Specifically, Schade and Fisher (1997) showed faster rates of decomposition (0.017–0.005 d−1) compared to this study, with the lowest rates of decomposition observed in sycamore leaves (0.005 d−1) relative to the ash, cottonwood, and willow leaves. Corman et al. (2016) showed similarly high rates of decomposition of maple (Acer grandidentatum) leaves, 0.016–0.003 d−1) in the wet-to seasonally intermittent sites in the Fort Huachuca. These differences among studies may indicate that short-term decomposition studies in temporary streams, particularly intermittent, and ephemeral streams, may overestimate organic matter dynamics and decay rates if only conducted during the aquatic phase. Difference in k across studies may also be explained owing to differences in litter type/quality, though a limited subset of sycamore leaves decomposed across these same sites in this study showed no substantial differences in k from oak leaves (Figure 5, Lohse et al., 2020a).
Faster rates of leaf decay have been typically observed in channels compared to other floodplain positions in perennial streams, similar to our findings. However, these effects often only emerged after 12–18 months (Gurtz and Tate, 1988; Anderson and Nelson, 2006; Langhans and Tockner, 2006; Langhans et al., 2008). In our study, differences in decomposition emerged among positions as early as 4 weeks after deployment and may possibly be explained based on differences in controls on decomposition in intermittent to ephemeral streams. Other global and regional analyses of organic matter and nutrient dynamics in intermittent streams have typically conducted lab incubations and pointed to the importance of rewetting and duration of wetting of litter and sediment in releasing of carbon dioxide (CO2) and nutrients (Gallo et al., 2014; Datry et al., 2018; Shumilova et al., 2019; Correa-Araneda et al., 2020). These studies have pointed to the need to measure in situ decomposition rates and measure the resumption of flow and quantifying different flow regime (Datry et al., 2018). Though limited in scope to dryland regions (MAP, 111–750 mm), we directly measured streamflow duration, water presence, and decomposition rates across a strong climate gradient and held litter quality constant to evaluate these hydroclimatic controls. We found that cumulative days of stream channel water presence and channel bed sediment texture explained 79 % of the variation in k across all sites (Figure 5). Proportional loss of mass and N (Figure 4D) in the channels indicated that physical fragmentation from cumulative duration of streamflow could likely explain much of this variation, but that water presence contributed to explaining slightly more variation in decomposition rates, especially on the dry end of the spectrum. Collectively, our findings indicate that stream water presence may be a more important indicator of ephemeral and intermittent stream function than streamflow alone. This finding warrants further investigation and consideration in other studies.
Our findings that differences in rates of mass loss were only associated with the channel positions, not the upland and riparian positions, were surprising given the wide range of actual precipitation received across flow regimes, 111–516 mm annual precipitation (Figures 4A–C). Many upland studies show climate and litter quality as the main factors controlling decomposition rates (e.g., Hobbie, 1992; Couteaux et al., 1995; Aerts, 1997). In this study, we held litter quality constant by transplanting the wettest site litter material across sites. Seminal decomposition studies comparing k in different litter types in upland areas show substantial differences in k associated with similar magnitude change in precipitation (~400 mm) (Melillo et al., 1982). Our results indicate that the subsidy of water in the channels as streamflow and/or soil-water is the main factor driving the increase in rates of decomposition in the seasonally- and wet-intermittent channel sites compared to the dry-intermittent and ephemeral flow regimes rather than climate differences alone. If climate factors were the main factor driving these differences, significantly higher rates of decomposition would have been observed in the riparian and upland positions at the seasonally- and wet-intermittent sites. The lack of difference in k in the riparian and upland position despite differences in precipitation could be explained by the comparable soil moisture dynamics observed in the upland and riparian position across flow regimes (Figure 6). High sand and silt content across riparian and upland sites (>91 %) and relatively similar water holding capacity (Table 1) could also help to explain similar soil moisture dynamics despite differences in precipitation across flow regimes. Finally, similar effective precipitation, the amount of water actually added and stored in the soil, due to significant increases in vegetation volume and richness in the riparian and upland environments with decreasing aridity as shown by Stromberg et al. (2017) may also help to explain lack of difference in soil moisture dynamics. Collectively these findings indicate that rates of decomposition in the terrestrial portions of these landscapes may be less sensitive than channels to declines in precipitation and associated increases in temperature.
Litter quality such as % N and C:N did not emerge as a major factor explaining rates of decomposition. Indeed, the lack of difference in total mass loss among flow regimes in the upland and riparian positions may be explained by net gains of N being immobilized from the surrounding environment (Figure 4) indicating possible nutrient limitation to decomposition in these upland environments (Hobbie and Vitousek, 2000). In contrast, channels showed N loss varying in proportion to mass loss indicating that differences in water presence and the physical breakdown or physical abrasion of litter by water were the main factors contributing to differences in rates of decomposition in the channels. As the duration of streamflow decreased from intermittent to ephemeral flow, the importance of the terrestrial phase of the channel increased and N loss decreased such that N loss in ephemeral channels mirrored patterns of N loss in upland and riparian positions (Figure 4). Corman et al. (2016) suggested that phosphorus (P) availability may limit decomposition in the same wet-intermittent to seasonally intermittent reaches that we studied where calcium carbonate deposition occurs. However, we observed higher decomposition rates in these sites with low P availability compared to the drier reaches with higher P availability.
Other abiotic factors such as soil coverage/sediment burial (Fritz et al., 2006; Barnes et al., 2012, 2015) and UV degradation (Austin and Vivanco, 2006; Throop and Archer, 2007), as well as biotic factors such as invertebrates (Anderson and Nelson, 2006) and microbial communities associated with the litter (Kaiser et al., 2014) may need further consideration to be able to explain the remaining variation in decomposition rates observed, particularly in the uplands. As mentioned above, lignin was not evaluated because litter quality was held constant in this study, though sycamore leaves decomposed at the same rate as oak leaves in channels suggesting that physical controls were more important than litter quality in these dryland regions. Furthermore, studies have indicated in the past that lignin is not likely associated with inhibiting effects in desert environments (Schaefer et al., 1985). Mesh size (1 mm) in our study likely limited invertebrates attack and therefore breakdown of litter. The role of invertebrates and microbial films/consortium in driving decomposition was beyond the scope of this study and merits further consideration as these processes have been shown to be important in driving decomposition in both the aquatic (Langhans et al., 2008; McCluney and Sabo, 2009; Allen et al., 2014) and terrestrial (Barnes et al., 2012) portions of landscapes. Finally, sediment burial has also been found to slow decomposition rates in intermittent coastal plain streams (Fritz et al., 2006) as well as in dryland upland environments (Barnes et al., 2012, 2015) and may help to explain further variation in decomposition rates given that some of channel litterbags were buried in sediment during the monsoon season.
Soil nutrient dynamics were more variable in space and time across this climate gradient, although patterns emerged especially in the dry ephemeral streams. Specifically, experimental wetting and in-situ wetting following monsoon rains resulted in elevated soil nitrate concentrations as extractable nitrate and in resin bags in ephemeral channels. Previous work in ephemeral washes has shown large release of nutrient and gas fluxes following wetting and controlled by sediment texture and associated properties (Gallo et al., 2014). High soil nitrate concentrations observed in our dry ephemeral washes after in-situ and experimental wetting were similar to those observed in the sand washes (6.8 ug N/ g dry soil) in Gallo et al. (2014). These findings suggest that soil nitrate concentrations and its accumulation may be a good indicator of streams transitioning from intermittent to ephemeral ones. They also point to rapid and large release of trace gases and nutrients following wetting that may not have been previously appreciated from dryland rivers (Marcé et al., 2019) and warrants further study in both natural and urban systems (Gallo et al., 2014).
Collectively, findings from this study indicate that decomposition and nutrient release in stream channels are tightly coupled to streamflow and water presence, and controls on these processes will reach a tipping point (Scheffer et al., 2009) as streams move from perennial to intermittent and ephemeral conditions with climate and/or land use change. Our data indicate that transitions to more temporary flow regimes (<40% streamflow) in this region and other dryland regions will result in a regime shift and rapid declines in organic matter processing (Figure 4) as well as gradual declines in soil stores of carbon and nutrients (Table 1). As such, changes in streamflow permanence associated with climate change may tip the relative importance of controls on decomposition and nutrient dynamics toward terrestrial compared to aquatic ones.
Data Availability Statement
The datasets presented in this study can be found in online repositories. The names of the repository/repositories and accession number(s) can be found below: Boise State University ScholarWorks doi: 10.18122/boisestate/reynoldscreek/17, doi: 10.18122/boisestate/reynoldscreek/18, doi: 10.18122/reynoldscreek/19.
Author Contributions
KL and TM conceived and designed the study. KL performed the research. KL and EG analyzed the data. EG and TM contributed new methods to the paper and KL wrote the paper. All authors reviewed the manuscript.
Funding
This study was funded by the Department of Defense Strategic Environmental Research and Development Program (SERDP) project number RC-1726. Partial support to Gallo for analysis and writing was supported by NSF EPSCOR IIA-1301792 and to Lohse by NSF EAR 1331872.
Conflict of Interest
The authors declare that the research was conducted in the absence of any commercial or financial relationships that could be construed as a potential conflict of interest.
Acknowledgments
We acknowledge the contributions of Julie Stromberg at Arizona State University who conceived the overall project, assisted with design of the study, and compiled the final report to SERDP (Stromberg et al., 2015). We thank Elizabeth Hatton, Stacy Schwabedissen, Noelle Guernsey at ISU with soil and litter collection and analyses and Hilary Nicholas and Jeff Silvertooth at UA for assistance with soil and hydrology analyses, and Barry M. Goldwater Air Force Base, Fort Huachuca Army Post, and Nature Conservancy Ramsey Canyon Preserve for access to sites.
Supplementary Material
The Supplementary Material for this article can be found online at: https://www.frontiersin.org/articles/10.3389/frwa.2020.571044/full#supplementary-material
Supplementary Figure 1. Cumulative precipitation dry-ephemeral [DE] sites (BG, SW, HP, GP), wet-ephemeral (WE) sites (RP, RL), dry-intermittent (DI) sites, (HU, GL), wetintermittent sites (WE) (HL, GU), and seasonally-intermittent (SI) sites (RU) locations since time of litterbag deployment.
References
Aerts, R. (1997). Climate, leaf litter chemistry and leaf litter decomposition in terrestrial ecosystems: a triangular relationship. Oikos 79, 439–449. doi: 10.2307/3546886
Allen, D. C., McCluney, K. E., Elser, S. R., and Sabo, J. L. (2014). Water as a trophic currency in dryland food webs. Front. Ecol. Environ. 12, 156–160. doi: 10.1890/130160
Alley, W. M., Healy, R. W., LaBaugh, J. W., and Reilly, T. E. (2002). Flow and storage in groundwater systems. Science 296, 1985–1990. doi: 10.1126/science.1067123
Anderson, D. C., and Nelson, S. M. (2006). Flood pattern and weather determine populus leaf litter breakdown and nitrogen dynamics on a cold desert floodplain. J. Arid Environ. 64, 626–650. doi: 10.1016/j.jaridenv.2005.06.022
Arce, M. I., Mendoza-Lera, C., Almagro, M., Catalán, N., Romaní, A. M., Martí, E., et al. (2019). A conceptual framework for understanding the biogeochemistry of dry riverbeds through the lens of soil science. Earth Sci. Rev. 188, 441–453. doi: 10.1016/j.earscirev.2018.12.001
Austin, A. T., and Vivanco, L. (2006). Plant litter decomposition in a semi-arid ecosystem controlled by photodegradation. Nature 442, 555–558. doi: 10.1038/nature05038
Barnes, P. W., Throop, H. L., Archer, S. R., Breshears, D. D., McCulley, R. L., and Tobler, M. A. (2015). Sunlight and soil–litter mixing: drivers of litter decomposition in drylands. Prog. Bot. 76, 273–302. doi: 10.1007/978-3-319-08807-5_11
Barnes, P. W., Throop, H. L., Hewins, D. B., Abbene, M. L., and Archer, S. R. (2012). Soil coverage reduces photodegradation and promotes the development of soil-microbial films on dryland leaf litter. Ecosystems 15, 311–321. doi: 10.1007/s10021-011-9511-1
Belnap, J., Welter, J. R., Grimm, N. B., Barger, N., and Ludwig, J. A. (2005). Linkages between microbial and hydrologic processes in arid and semiarid watersheds. Ecology 86, 298–307. doi: 10.1890/03-0567
Binkley, D., and Matson, P. (1983). Ion exchange resin bag method for assessing forest soil nitrogen availability. Soil Sci. Soc. Am. J. 47, 1050–1052.
Blasch, K. W., Ferre, T. P. A., Christensen, A. H., and Hoffmann, J. P. (2002). New field method to determine streamflow timing using electrical resistance sensors. Vadose Zone J. 1, 289–299. doi: 10.2136/vzj2002.2890
Corman, J. R., Moody, E. K., and Elser, J. J. (2016). Calcium carbonate deposition drives nutrient cycling in a calcareous headwater stream. Ecol. Monogr. 86, 448–461. doi: 10.1002/ecm.1229
Correa-Araneda, F., Tonin, A. M., Pérez, J., Álvarez, K., López-Rojo, N., Díaz, A., et al. (2020). Extreme climate events can slow down litter breakdown in streams. Aquat. Sci. 82:25. doi: 10.1007/s00027-020-0701-9
Couteaux, M. M., Bottner, P., and Berg, B. (1995). Litter decomposition, climate and litter quality. Trends Ecol. Evol. 10, 63–66. doi: 10.1016/S0169-5347(00)88978-8
Dane, J. H., Topp, G. C., and Campbell, G. S. (2002). Methods of Soil Analysis: Physical Methods. (Madison, WI: SSSA), 721–738.
Datry, T., Corti, R., Claret, C., and Phillippe, M. (2011). Intermittence controls leaf litter breakdown in a French temporary alluvial river: the “drying memory”. Aquat. Sci. 73, 471–483. doi: 10.1007/s00027-011-0193-8
Datry, T., Foulquier, A., Corti, R., von Schiller, D., Tockner, K., Mendoza-Lera, C., et al. (2018). A global analysis of terrestrial plant litter dynamics in non-perennial waterways. Nat. Geosci. 11, 497–503. doi: 10.1038/s41561-018-0134-4
Datry, T., Larned, S. T., and Tockner, K. (2014). Intermittent rivers: a challenge for freshwater ecology. Bioscience 64, 229–235. doi: 10.1093/biosci/bit027
Döll, P., and Schmied, H. M. (2012). How is the impact of climate change on river flow regimes related to the impact on mean annual runoff? A global scale analysis. Environ. Res. Lett. 7, 14–37. doi: 10.1088/1748-9326/7/1/014037
Fritz, K. M., Feminella, J. W., Colson, C., Lockaby, B. G., Governo, R., and Rummer, R. B. (2006). Biomass and decay rates of roots and detritus in sediments of intermittent Coastal Plain streams. Hydrobiologia 556, 265–277. doi: 10.1007/s10750-005-1154-9
Gallo, E. L., Lohse, K. A., Brooks, P. D., Ferlin, C., and Meixner, T. (2014). Physical and biological controls on trace gas fluxes in semi-arid urban ephemeral waterways. Biogeochemistry 121, 189–207. doi: 10.1007/s10533-013-9927-0
[Dataset] Gallo, E. L., Meixner, T., Lohse, K. A., and Nicholas, H. (2020). Dataset for Estimating Surface Water Presence and Streamflow of Ephemeral and Intermittent Streams in Southwest US. doi: 10.18122/reynoldscreek/19/boisestate
Gee, G. W., and Bauder, J. W. (1986). “Particle-size analysis” in Methods of Soil Analysis. Part 1 - Physical and Mineralogical Properties, ed A. Klute (Madison, WI: Soil Science Society of America), 383–411.
Goodrich, D. C., Lane, L. J., Shillito, R. M., Miller, S. N., Syed, K. H., and Woolhiser, D. A. (1997). Linearity of basin response as a function of scale in a semiarid watershed. Water Resour. Res. 33, 2951–2965. doi: 10.1029/97WR01422
Goodrich, D. C., Williams, D. G., Unkrich, C. L., Hogan, J. F., Scott, R. L., Hultine, K. R., et al. (2004). “Comparison of methods to estimate ephemeral channel recharge, Walnut Gulch, San Pedro River Basin, Arizona,” in Groundwater Recharge in a Desert Environment: The Southwestern United States, eds J. F. Hogan, F. M. Phillips, and B. R. Scanlon (New York, NY: Water and Science Application 9, American Geophysical Union), 77–99.
Grossman, R. B., and Reinsch, T. G. (2002). “Bulk density and linear extensibility,” in Methods of Soil Analysis. Part 4. Physical Methods. eds J.H. Dane, and C. Topp (Madison, WI: Soil Science Society of America), 201–225.
Gurtz, M. E., and Tate, C. M. (1988). Hydrologic influences on leaf decomposition in a channel and adjacent bank of a gallery forest stream. Am. Midland Nat. 120, 11–21. doi: 10.2307/2425882
Hart, S. C., Stark, J. M., Davidson, E. A., and Firestone, M. K. (1994). “Nitrogen mineralization, immobilization, and nitrification,” in Methods of Soil Analyses: Biochemical and Microbiological Properties, ed R. E. A. Weaver (Madison, WI: Soil Science Society of America), 985–1018.
Heilweil, V. M., Solomon, D. K., Perkins, K. S., and Ellett, K. M. (2004). Gas-partitioning tracer test to quantify trapped gas during recharge. Ground Water 42, 589–600. doi: 10.1111/j.1745-6584.2004.tb02627.x
Hobbie, S. E. (1992). Effects of plant species on nutrient cycling. Trends Ecol. Evol. 7, 336–339. doi: 10.1016/0169-5347(92)90126-V
Hobbie, S. E., and Vitousek, P. M. (2000). Nutrient limitation of decomposition in Hawaiian forests. Ecology 81, 1867–1877. doi: 10.1890/0012-9658(2000)081(1867:NLODIH)2.0.CO;2
Jaeger, K. L., and Olden, J. D. (2012). Electrical resistance sensor arrays as a means to quantify longitudinal connectivity of rivers. River Res. Appl. 28, 1843–1852. doi: 10.1002/rra.1554
Jaeger, K. L., Olden, J. D., and Pelland, N. A. (2014). Climate change poised to threaten hydrologic connectivity and endemic fishes in dryland streams. Proc. Natl. Acad. Sci. U.S.A. 111, 13894–13899. doi: 10.1073/pnas.1320890111
Kaiser, C., Franklin, O., Dieckmann, U., and Richter, A. (2014). Microbial community dynamics alleviate stoichiometric constraints during litter decay. Ecol. Lett. 17, 680–690. doi: 10.1111/ele.12269
Lane, L. J. (1983). “Chapter 19: transmission losses,” in SCS National Engineering Handbook. (Washington, DC: US Gov. Printing Office), 19.11–19.21.
Langhans, S. D., Tiegs, S. D., Gessner, M. O., and Tockner, K. (2008). Leaf-decomposition heterogeneity across a riverine floodplain mosaic. Aquat. Sci. 70, 337–346. doi: 10.1007/s00027-008-8062-9
Langhans, S. D., and Tockner, K. (2006). The role of timing, duration, and frequency of inundation in controlling leaf litter decomposition in a river-floodplain ecosystem (Tagliamento, Northeastern Italy). Oecologia 147, 501–509. doi: 10.1007/s00442-005-0282-2
Larned, S. T., Datry, T., Arscott, D. B., and Tockner, K. (2010). Emerging concepts in temporary-river ecology. Freshw. Biol. 55, 717–738. doi: 10.1111/j.1365-2427.2009.02322.x
Levick, L., Fonseca, J., Goodrich, D., Hernandez, M., Semmens, D., Stromberg, J., et al. (2008). The Ecological and Hydrological Significance of Ephemeral and Intermittent Streams in the Arid and Semi-arid American Southwest. Tucson, AZ: US Environmental Protection Agency and USDA/ARS Southwest Watershed Research Center.
Lohse, K. A., Gallo, E. L., and Meixner, T. (2020a). Dataset on Rates of in-situ Litter Decomposition Across a Range of Ephemeral to Seasonally-Intermittent Stream Reaches and Landscape Positions in Arizona, USA. Boise, ID: Boise State University Scholarworks.
Lohse, K. A., Gallo, E. L., and Meixner, T. (2020b). Dataset on Soil Physio-Chemical Properties and Seasonal Moisture and Nutrient Dynamics in Temporary Stream Channels and Contributing Uplands in Arizona, USA. Boise, ID: Boise State University ScholarWorks.
Marcé, R., Obrador, B., Gómez-Gener, L., Catalán, N., Koschorreck, M., Arce, M. I., et al. (2019). Emissions from dry inland waters are a blind spot in the global carbon cycle. Earth Sci. Rev. 188, 240–248. doi: 10.1016/j.earscirev.2018.11.012
McCluney, K. E., and Sabo, J. L. (2009). Water availability directly determines per capita consumption at two trophic levels. Ecology 90, 1463–1469. doi: 10.1890/08-1626.1
Melillo, J. M., Aber, J. D., and Muratore, J. F. (1982). Nitrogen and lignin control of hardwood leaf litter decomposition dynamics. Ecology 63, 621–626. doi: 10.2307/1936780
Postel, S. L., Daily, G. C., and Ehrlich, P. R. (1996). Human appropriate of renewable fresh water. Science 271, 785–787. doi: 10.1126/science.271.5250.785
PRISM Climate Group (2013). PRISM Climate Data. Oregon State University. Available online at: http://prism.oregonstate.edu
Reynolds, J. F., Maestre, F. T., Kemp, P. R., Stafford- Smith, D. M., and Lambin, E. (2007). “Natural and human dimensions of land degradation in drylands: causes and consequences,” in Errestrial Ecosystems in a Changing World, eds D.P. J. Canadell, L. F. Pitelka (Berlin: Springer), 247–258.
Sangiorgio, F., Fonnesu, A., and Mancinelli, G. (2007). Effect of drought frequency and other reach characteristics on invertebrate communities and litter breakdown in the intermittent Mediterranean river Pula (Sardinia, Italy). Int. Rev. Hydrobiol. 92, 156–172. doi: 10.1002/iroh.200510953
Scanlon, B. R., and Goldsmith, R. S. (1997). Field study of spatial variability in unsaturated flow beneath and adjacent to playas. Water Resour. Res. 33, 2239–2252. doi: 10.1029/97WR01332
Scanlon, B. R., Langford, R. P., and Goldsmith, R. S. (1999). Relationship between geomorphic settings and unsaturated flow in an arid setting. Water Resour. Res. 35, 983–999. doi: 10.1029/98WR02769
Schade, J., and Fisher, S. G. (1997). Leaf litter in a sonoran desert stream ecosystem. J. North Am. Benthol. Soc. 16, 612–626. doi: 10.2307/1468148
Schaefer, D., Steinberger, Y., and Whitford, W. G. (1985). The failure of nitrogen and lignin control of decomposition in a North American desert. Oecologia 65, 382–386. doi: 10.1007/BF00378913
Scheffer, M., Bascompte, J., Brock, W. A., Brovkin, V., Carpenter, S. R., Dakos, V., et al. (2009). Early-warning signals for critical transitions. Nature 461, 53–59. doi: 10.1038/nature08227
Seager, R., Ting, M. F., Held, I., Kushnir, Y., Lu, J., Vecchi, G., et al. (2007). Model projections of an imminent transition to a more arid climate in southwestern North America. Science 316, 1181–1184. doi: 10.1126/science.1139601
Shumilova, O., Zak, D., Datry, T., von Schiller, D., Corti, R., Foulquier, A., et al. (2019). Simulating rewetting events in intermittent rivers and ephemeral streams: a global analysis of leached nutrients and organic matter. Glob. Chang. Biol. 25, 1591–1611. doi: 10.1111/gcb.14537
Stanley, E. H., Fisher, S. G., and Grimm, N. B. (1997). Ecosystem expansion and contraction in streams. Bioscience 47, 427–435. doi: 10.2307/1313058
Stromberg, J., Gallo, E., Lohse, K., Meixner, T., Moody, E., Sabo, J., et al. (2015). “Structure and function of ephemeral streams in the arid and semiarid Southwest: implications for conservation and management,” in Department of Defense, Strategic Environmental Research and Development Program (Alexandria, VA).
Stromberg, J., Setaroa, D., Gallo, E., Lohse, K., and Meixner, T. (2017). Riparian vegetation of ephemeral streams. J. Arid Environ. 138, 27–37. doi: 10.1016/j.jaridenv.2016.12.004
Tank, J. L., Rosi-Marshall, E. J., Griffiths, N. A., Entrekin, A. A., and Stephen, M. L. (2010). A review of allochthonous organic matter dynamics and metabolism in streams. J. North Am. Benthol. Soc. 29, 118–146. doi: 10.1899/08-170.1
Thomas, G. W. (1996). “Soil pH and soil acidity,” in Methods of Soil Analysis-Part 3 Chemical Methods, eds D. L. Sparks, A. L. Page, P. A. Helmeke, R. H. Loeppert, P. N. Soltanpour, M. A. Tabatabai, C. T. Johnston, and M. E. Summer (Madison, WI: SSSA), 475–490.
Throop, H. L., and Archer, S. R. (2007). Interrelationships among shrub encroachment, land management and leaf litter decomposition in a semi-desert grassland. Ecol. Appl. 17, 1809–1823. doi: 10.1890/06-0889.1
U.S. Geological Survey (2008). National Hydrography Dataset (ver. 2 USGS National Hydrography Dataset). Available online at: https://www.usgs.gov/core-science-systems/ngp/national-hydrography/access-national-hydrography-products
Webster, J. R., and Benfield, E. F. (1986). Vascular plant breakdown in freshwater ecosystems. Annu. Rev. Ecol. Syst. 17, 567–594. doi: 10.1146/annurev.es.17.110186.003031
Keywords: temporary streams, decomposition, thresholds, climate change, ephemeral stream flow, intermittent stream flow
Citation: Lohse KA, Gallo EL and Meixner T (2020) Influence of Climate and Duration of Stream Water Presence on Rates of Litter Decomposition and Nutrient Dynamics in Temporary Streams and Surrounding Environments of Southwestern USA. Front. Water 2:571044. doi: 10.3389/frwa.2020.571044
Received: 09 June 2020; Accepted: 04 September 2020;
Published: 28 October 2020.
Edited by:
Dipankar Dwivedi, Lawrence Berkeley National Laboratory, United StatesReviewed by:
Joel Rowland, Los Alamos National Laboratory (DOE), United StatesPin Shuai, Pacific Northwest National Laboratory (DOE), United States
Copyright © 2020 Lohse, Gallo and Meixner. This is an open-access article distributed under the terms of the Creative Commons Attribution License (CC BY). The use, distribution or reproduction in other forums is permitted, provided the original author(s) and the copyright owner(s) are credited and that the original publication in this journal is cited, in accordance with accepted academic practice. No use, distribution or reproduction is permitted which does not comply with these terms.
*Correspondence: Kathleen A. Lohse, a2xvaHNlJiN4MDAwNDA7aXN1LmVkdQ==