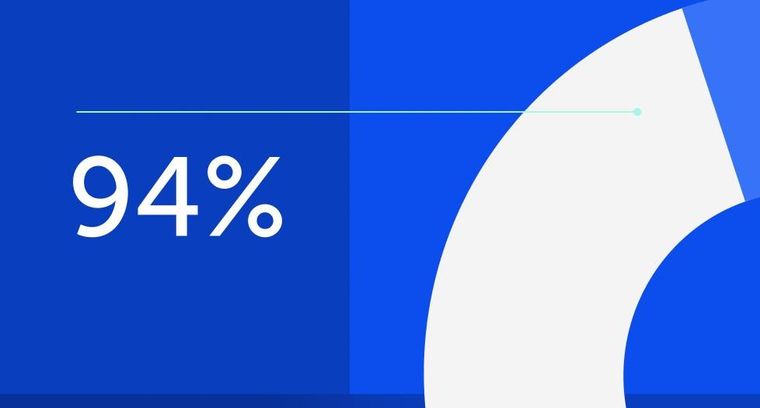
94% of researchers rate our articles as excellent or good
Learn more about the work of our research integrity team to safeguard the quality of each article we publish.
Find out more
ORIGINAL RESEARCH article
Front. Virtual Real., 31 May 2022
Sec. Haptics
Volume 3 - 2022 | https://doi.org/10.3389/frvir.2022.894575
This article is part of the Research TopicNew Materials and Technologies for Haptics that Enhance VR and ARView all 4 articles
Despite the ubiquitous presence of tactile actuators (tactors) in mobile devices, there is a continuing need for more advanced tactors that can cover the entire frequency range of human tactile perception. Broadband tactors can increase information transmission and enrich sensory experience. The engineering challenges are multifold in that the ideal tactors should exhibit an effective bandwidth of at least 300 Hz, small form factor, robustness, power efficiency and low cost. For wearable applications, there are the additional challenges of ease of mounting and maintaining adequate skin contact during body movements. We propose an approach to interleave narrowband tactile stimuli to achieve broadband effects, taking advantage of the limited spatial resolution of the skin on the torso and limbs. Three psychophysical experiments were conducted to assess the validity of this approach. Participants performed pairwise discriminations of two broadband stimuli delivered using one or two tactors. The broadband stimuli consisted of one mid-frequency and one high-frequency component delivered through one tactor by mixing the two components, or through two tactors (one component per tactor). The first two experiments revealed extraneous cues such as localization and mutual masking of mid- and high-frequency components that were subsequently eliminated in the third experiment. Results from 12 participants confirmed that performance on pairwise comparisons was below the discrimination threshold, confirming that broadband haptic effects can be achieved through narrowband tactors placed within the skin’s two-point limen.
There has been a growing interest in wearable and array-based vibrotactile displays in academic research and commercial products in the recent years. With this interest comes a need for tactors (tactile stimulators) that can be easily attached to the skin at various body sites, and have large intensity and frequency ranges that can be independently controlled. Previous research with these type of displays include tactor arrays on hands (Hsieh et al., 2016; Park et al., 2016; Park et al., 2019), wrist (Chen et al., 2008; Lee and Starner, 2010; Matscheko et al., 2010; Liao et al., 2016), arm (Cholewiak and Collins, 2003; Brown et al., 2006; Jones et al., 2009; Culbertson et al., 2018; Reed et al., 2019), waist (Cholewiak et al., 2004; Van Erp, 2005a; Cholewiak and McGrath, 2006; Elliott et al., 2013) and torso (Ertan et al., 1998; Rupert, 2000; Jones et al., 2009; Israr and Poupyrev, 2011). Similarly, commercial products include the Optacon for persons who are visually impaired (Telesensory Corp., Mountain View, CA), Tactaid VII for the hearing impaired (Audiological Engineering Corp., Somerville, MA) and more recent creations for virtual reality and sensory substitution (e.g., TESLASUIT by VR Electronics Ltd. in United Kingdom; SUBPAC X1 by Subpac Inc. in Palo Alto, CA; Buzz by Neosensory in San Francisco, CA; Hi5 VR Gloves by Noitom in Beijing, China; and Dot Watch by Dot Inc. in South Korea). With few exceptions, the tactors used in these examples are resonant devices that operate most efficiently within a narrow, high-frequency band (
In the range of sinusoidal frequencies from
Tactor arrays can also achieve high levels of information transmission by delivering multi-dimensional tactile stimuli via broadband actuators [see a recent review by Tan et al. (2020a)]. For instance, the OMAR device by Eberhardt et al. (1994) was used to control up to 10 channels of one-dimensional motion, or five channels of two-dimensional motion in the range from DC to 800 Hz. Studies of vibration onset asynchrony (VOA) demonstrated that participants could identify whether vibrations proceeded movement for asynchronies in the range of tens of milliseconds. This result demonstrated that VOA could be used to disambiguate lipreading of stop consonants in haptic supplements for lipreading. Another example is the Tactuator by Tan and Rabinowitz (1996). The device was composed of three independent motor assemblies used to deliver vibrotactile stimuli to the finger pads of the thumb, index and middle fingers in the range of DC to 300 Hz. Tan et al. (1999) used the device to conduct absolute identification experiments with three sets of distinct stimuli. They demonstrated a maximum information transfer rate of 12 bits/sec. More recently, Reed et al. (2019) designed a TActile Phonemic Sleeve (TAPS) for speech communication on the skin. The device consisted of a 4-by-6 broadband tactor array used to encode the 39 English phonemes as 39 distinct multi-dimensional vibrotactile stimuli delivered to the forerarm. They reported a phoneme recognition rate of 86% after one to 4 h of learning. The set of phonemic tactile codes used mid- and high-frequency signals and amplitude modulation to achieve perceptually-distinct sensations that could be easily learned and memorized. Using the same codes in the TAPS, Tan et al. (2020b) trained 51 participants on the reception of up to 500 English words. The best participants could achieve a learning rate of roughly one word per minute. Despite the success of the TAPS, the broadband tactors employed in the TAPS display are relatively large and difficult to attach to the forearm, making them suitable for lab studies but not for wearable applications.
The aforementioned research and applications make use of commercially-available tactors with different operating principles. The majority of mobile devices use either ERM (eccentric rotating mass) or LRA (linear resonant actuator) tactors, while others use solenoids or piezoelectric actuators (Jones and Sarter, 2008; Choi and Kuchenbecker, 2012). LRAs are often preferred when independent control of amplitude and frequency is required. These are high-Q actuators with a peak output over a narrow frequency range that typically centers above 100 Hz (Hayward and MacLean, 2007; Choi and Kuchenbecker, 2012), although some are designed with a lower resonant frequency. For example, the C2 tactor has a frequency range of 200–300 Hz, while the range of the C2-HDLF tactor is 50–160 Hz (Engineering Acoustics, Orlando, FL, United States). In contrast, tactors with lower Q factors have much wider bandwidths (e.g., 50–500 Hz for the Haptuator by TactileLabs in Montreal, Canada; 35–1,000 Hz for Lofelt’s L5). These are commonly less power efficient, larger in form factor, heavier and more costly. A comparison of some of these commercial actuators is provided in Table 1.
Ideally, a tactor should be small, power efficient, low cost, easily mountable, and capable of conveying rich haptic effects over the entire frequency range of 0–1,000 Hz. However, building such a tactor is a challenging task. We hypothesize that it may not be necessary to build such a broadband actuator to achieve broadband perceptual haptic effects. In the present study, we present an alternative way to achieve rich haptic experiences using commercially-available tactors by taking advantage of the limited spectral and spatial resolution of the skin.
The frequency resolution of the human skin is very limited (Goff, 1967; Franzen and Nordmark, 1975; Verrillo and Gescheider, 1992; Israr et al., 2006; Mahns et al., 2006). Therefore, it is unnecessary for a tactor to exhibit a continuous frequency response over the entire 0–1,000 Hz range to achieve perceptually broadband haptic effects. In fact, previous research has shown that only three distinct sensations can be elicited from single-frequency sinusoidal stimulation over the range of 0–1,000 Hz: pressure variation/slow motion at low frequency (up to ≈6 Hz), fluttery (with low amplitude)/rough (with high amplitude) at mid frequency (≈10–70 Hz) and smooth vibration at high frequency (above 100 Hz) (Talbot et al., 1968; Merzenich and Harrington, 1969; Mountcastle et al., 1969; Tan et al., 1999). Vibrations from these ranges can be combined and remain salient perceptually (Marks, 1979; Makous et al., 1995; Tan et al., 1999). For example, a dual-frequency vibration with 30 and 300-Hz components feels like a smooth vibration (due to 300 Hz) with superimposed roughness (due to 30 Hz). Research on the perception of combined frequencies from the three regions has resulted in other interesting discoveries. For example, Park and Choi (2011) studied the perceptual space of amplitude-modulated sinusoidal signals using multi-dimensional scaling (MDS). The stimuli shared a carrier frequency of 150 Hz and varied their modulating frequency from 0 (no modulation) to 80 Hz. The optimal perceptual space had two dimensions and the modulating frequencies formed a circle in the space. It was found that modulations below 10 Hz result in more discernible vibrations, i.e., it is easier to distinguish individual frequency components in the amplitude-modulated signal. Furthermore, Yoo et al. (2014) studied the degree of consonance of “vibrotactile chords,” i.e., vibrotactile signals composed of superimposed frequencies that resemble musical chords. The chords were dual-frequency vibrations. In each signal, a base frequency of 40, 55, 80, or 110 Hz was paired with one of 19 semitones derived from the base frequency. The researchers demonstrated that signals sensed as high-pitch vibrations are consonant, whereas low-frequency, fluttering, pulsatory, rough and low-pitch vibrations were judged as dissonant. These studies demonstrate that a few discrete frequency values can be combined to elicit rich haptic sensations, as opposed to a continuous range of frequencies.
As far as spatial resolution is concerned, the two-point limen (threshold at which two contact points on the skin are felt as one) varies greatly across body sites. Except for the hand, the two-point limen is at least 30 mm on the body surface (Weber, 1834/1978; Weinstein, 1968). It follows that sufficiently small tactors can be placed closely on the skin and perceived as a single tactor.
With these considerations, we hypothesized that placing narrowband tactors within the two-point limen of the skin can effectively deliver broadband haptic effects indistinguishable from those delivered by one broadband tactor. Up to three narrowband tactors can be placed at each stimulation site with each tactor operating over the low-, mid- or high-frequency range, respectively. We tested our hypothesis using a paired-comparison psychophysical procedure. Participants compared a broadband stimulus delivered with one tactor and multiple narrowband stimuli simultaneously delivered with multiple tactors. We predicted that the two types of stimuli could not be distinguished provided that extraneous cues were either eliminated or matched for the two stimulus types. We employed mid- and high-frequency vibrations in the present study.
Findings from three experiments are reported here. In the first experiment, we sought to equalize the perceived intensity of vibrotactile stimuli, but found that the participants were still able to discriminate the two types of stimuli under some conditions. In the second experiment, we refined the intensity matching procedure to take into account the mutual masking of mid- and high-frequency components and changed the stimulation site to remove localization cues. It was also found that the vibration amplitude at the lowest mid-frequency may have been limited with the tactor chosen for this study. Therefore, a higher mid-frequency was used in the third experiment. The general methods of this work and the contents of Exp. 3 were included in a previous publication by Martinez et al. (2021) This paper includes the findings and discussions from the first two experiments that informed the design of the third experiment. In the remainder of this paper, we first present the general methods that are common to the three experiments. This is followed by the design and procedures specific to each of the three experiments along with the respective results and discussion. Finally, we present guidelines for interleaving narrowband tactors to achieve broadband effects based on the findings from the present investigation.
A total of twelve participants (P01 to P12; 6F; 23–30 years old, 26.2 ± 1.9 years) took part in the present study. All had a normal sense of touch by self report. Two of the participants (P01, the first author, and P02) took part in Exp. 1 and Exp. 2. All participants were tested in Exp. 3. Each participant signed an informed consent form approved by the Purdue University IRB when they reviewed and approved the human-use protocols. They received a compensation of 10 USD per hour for their time.
In our simple discrimination test (see Section 2.5 for details on the procedure), knowledge about the experimental design does not provide the participant with any advantage nor would it induce any additional response bias. Therefore, P01 (the first author) had to rely entirely on the perceived difference between the stimulus alternatives to perform the task, just like the other participants.
Two tactors were used (Figure 1) in all the experiments. They were broadband audio speakers (Tectonic Elements, model TEAX13C02-8/RH) with an impedance of 8 Ω across the frequency range of 50–1,000 Hz, except for a peak impedance of 35 Ω at ≈ 600 Hz. Each tactor measures 26.3 mm in diameter (32.2 mm with soldering tab) and 9.0 mm in thickness. A circular adhesive ring on top of the diaphragm provides attachment. It is known that detection thresholds decrease with contactor area until ≈2.9 cm2 (Verrillo, 1963). Therefore, a white 3D-printed plastic disk was attached to the adhesive ring to increase the contactor area to ≈3.8 cm2 (see the white top in Figure 1; the brown rim belongs to the diaphram of the tactor underneath the plastic ring). Measurements taken with an accelerometer (Kistler 8794A500) attached to the disk verified that the tactors were able to deliver vibrations without distortion in the frequency range of 10–500 Hz. Tactors were placed side-by-side on a Velcro band without touching (see Figure 1). Given their close proximity, we verified that the activation of one tactor did not induce enough electromagnetic noise in the adjacent tactor to produce a perceivable vibration. To this end, we took acceleration measurements on a resting tactor while the other tactor vibrated at 10, 30, 60, 150 and 300 Hz. Each frequency was delivered at intensities of 20 and 30 dB (relative to the maximum output of the system). We estimated the corresponding displacement of the resting tactor from the peak acceleration measured. Table 2 shows the calculated displacements along with the detection thresholds in hairy skin reported in (Bolanowski et al., 1994). As shown, the measurements are well below the detection thresholds at each frequency.
FIGURE 1. The two tactors used in the experiment. A white 3D-printed plastic disk is attached to the underlying adhesive ring connected to the tactor diaphragm (visible as an orange border around the plastic disk).
TABLE 2. Measured displacement of the resting tactor while the other tactor is active. The detection thresholds in hairy skin from (Bolanowski et al., 1994) are shown for comparison.
The same tactors were used in the TAPS system for speech communication on the skin (Reed et al., 2019; Tan et al., 2020b). While the tactors work well after calibration in a laboratory setting, they are not suitable for wearable applications due to their large size, variability among individual tactors, and the difficulty of maintaining consistent contact with the skin during arm movement. For these reasons, the present study is still needed to explore the use of two or three narrowband tactors in place of one broadband tactor, when commercially-available tactors with small form factors are used in a wearable tactile display.
The tactors were connected to class D stereo amplifiers (Maxim MAX98306) that received input from a MOTU 24Ao audio interface (MOTU, Inc., Cambridge, MA, United States). The MOTU audio interface performed synchronous D/A conversion of a 2-channel MATLAB waveform played with the Playrec utility (Humphrey, 2008).
Two stimulation sites were used in the present study, as shown in Figure 2. In Exp. 1, the two tactors were placed on the dorsal side at the middle of the forearm (see Figure 2A). The upper arm was used in Exps. 2 and 3 where the tactors were placed on top of the bicep muscles (see Figure 2B).
FIGURE 2. Tactor configuration in the three experiments. The location of the two tactors underneath the Velcro band are indicated by red text. (A) Tactors worn at the middle of the dorsal forearm. The elbow and wrist rested on top of two foam supports. (B) Exps. 2 & 3: Tactors worn at the upper arm, atop the left biceps.
To ensure that the stimuli were delivered to the skin at the same perceived intensity for all participants, they were calibrated in two steps. First, the detection thresholds of all participants were estimated at two high frequencies (150, 300 Hz). Second, the output of the two tactors was equalized. These steps accounted for the variation in detection thresholds among the participants and possible differences between the two tactors.
Detection thresholds were measured for each participant at the beginning of the experiment using tactor T2 which was closer to the torso (see Figure 2). Thresholds were measured at 150 and 300 Hz using a three-interval, two-alternative, forced-choice, one-up two-down adaptive procedure with trial-by-trial response feedback. The one-up two-down rule estimates the 70.7-percentile point on the psychometric function (Levitt, 1971). The vibration amplitude was adjusted with a step size of 5 dB at the first four reversals, and 2 dB at an additional 12 reversals. On each trial, a 400-ms signal was presented in only one of the three intervals, randomly selected with equal a priori probabilities. Each interval was visually indicated and the gap between intervals was 500 ms. The participant indicated which interval contained the signal and received feedback for that trial. The threshold was estimated as the mean of the last twelve reversals at the smaller step size.
The participants then completed a method-of-adjustment procedure to equalize the perceived intensity of the two tactors. A 400-ms long signal at 300 Hz and −10 dB (relative to the maximum output allowed by the MATLAB software) was delivered to T2, the reference tactor. The participant adjusted the amplitude of a 400-ms, 300-Hz signal on the test tactor, T1, until the two were perceived to be equally strong. The tactors were activated in the sequence reference-test-reference and the participant increased or decreased the intensity of the test tactor in steps of 1 dB. The final adjustment was recorded. On average, the adjusted values differed by 0.83 dB relative to that at the reference tactor.
The results of threshold measurement and tactor equalization were used to calculate signal amplitudes that corresponded to specific sensation levels (SLs) at high frequencies. They refer to the vibration amplitude relative to the detection threshold at the corresponding frequency in the unit of dB SL. Verrillo et al. (1969) showed that sensation levels grow almost linearly with signal amplitudes when both are expressed in dB (see Figures 8, 9 of (Verrillo et al., 1969) for the vibrotactile equal-sensation magnitude curves).
To account for spectral masking of mid- and high-frequency signal components, additional calibrations were performed for the mid-frequency signals. In the first two experiments, 10 and 30 Hz were used in the mid-frequency range. These changed to 30 and 60 Hz in the third experiment. The calibration procedures evolved with the experiments. They will be described later in each experiment.
All experiments employed a one interval, two alternatives, forced choice (1I-2AFC) paired-comparison paradigm, following the design of R. Cholewiak and Collins (2000; Exp. 2) (Cholewiak and Collins, 2000) and S. Cholewiak et al. (2010; Exp. 3) (Cholewiak et al., 2010). The two stimulus alternatives included a dual-frequency vibration delivered with one tactor (“broadband”) and two single-frequency vibrations delivered with the two adjacent tactors (“narrowband”). For the broadband stimulus alternative, one of the two tactors was randomly selected to be driven with the sum of two sinusoidal waveforms, one at a mid frequency (10, 30 or 60 Hz) and the other at a high frequency (150 or 300 Hz). The other tactor was not activated. For the narrowband stimulus alternative, one randomly-selected tactor was driven with a single-frequency vibration at a mid frequency, and the other tactor at a high frequency. The randomization of tactor selection was performed on each trial. The signals were 400 ms in duration and smoothed by a 5-ms Hanning window so they started and ended at zero amplitude. The specific frequency values used in an experiment varied, and will be described later in the respective experiments.
The participant wore a thin fabric sleeve on the left arm for hygiene purposes. The experimenter wrapped the tactor band around the stimulation site (forearm or upper arm) and fastened it with Velcro (see Figure 3). The participant sat facing the computer screen with the left arm resting comfortably on a table and the elbow supported. Audio pink noise was played through a headset throughout the experiment to mask any audible sounds from the apparatus.
FIGURE 3. Experimental setup in Exp. 3. The tactor band was worn on top of the left biceps. The computer screen shows the interface for pairwise discrimination.
After the signal-intensity calibration steps, data collection for pairwise discrimination began. At the beginning of each experimental condition, the two stimulus alternatives were presented once to the participant with their respective response labels shown as “A” or “B.” This was followed by a block of 60 trials with the first 10 trials considered as training and discarded from data analysis. On each trial, one of the two stimulus alternatives was presented with an equal a priori probability of 0.5. The participant felt the stimulus and responded by clicking one of two buttons on the computer screen. A check mark appeared above the selection for a correct response. For an incorrect response, a cross appeared above the incorrectly selected button and a check mark was shown above the button with the correct response label. The correct-answer feedback served to ensure that the participant used the correct response labels and reduced the response bias in the two-interval discrimination task. It remained on the computer screen for 1 s and the next trial started immediately thereafter. Participants were allowed to take a break at the end of each block of trials. They continued with the next 60-trial block by clicking on a “Next” button when ready.
The results of the 1I-2AFC discrimination experiment were analyzed using the decision model from Signal Detection Theory (Green and Swets, 1966; Macmillan and Creelman, 2004; Jones and Tan, 2013). Compared to many other psychophysical paradigms such as method of constant stimuli, method of adjustment and adaptive procedures, Signal Detection Theory provides a discrimination performance measure d′ that is independent of response bias c. It is therefore preferred over the commonly-used percent-correct scores. A d′ value of 1.0 indicates threshold performance. Therefore, if d′ ≥ 1.0 (or d′ < 1.0), we conclude that the participants can (or cannot) distinguish the stimulus pair. Details on the theory and computation of d′ and response bias c are available in Supplementary Appendix.
The pairwise discrimination could be accomplished using any number of perceptual cues. The most obvious cue was that of perceived intensity of different frequency components. It was paramount that the perceived intensity of the two stimulus alternatives be equalized, not only for the high-frequency components but also for the mid-frequency components due to possible spectral masking effects. In Exp. 1, the detection thresholds at 10 and 30 Hz in the presence of 150 or 300 Hz maskers were estimated before determining the signal amplitudes at the mid frequencies. The results suggested the presence of additional perceptual cues, addressed in Exp. 2.
There were eight conditions in Exp. 1, based on the combinations of 1) two mid frequencies (10, 30 Hz), 2) two high frequencies (150, 300 Hz), and 3) two sensation levels (20, 30 dB SL). The order of experimental conditions was randomized for each participant.
In order to eliminate any tactor localization cues, it was important to place the two tactors within the two-point limen of the body site. The tactors used in the present study needed a minimum center-to-center distance of ≈30 mm to avoid direct contact. According to Weber (1834/1978) and Weinstein (1968), this was below the 40 mm two-point limen for touch on the forearm. Using vibrations at 100 and 250 Hz, Cholewiak and Collins (2003) applied an array of vibrotactile actuators spaced by 25 mm on the volar forearm to study tactor localization. Localization was poorest at the middle of the array (≈40%). Subsequent analysis showed errors to be evenly distributed between the two tactors adjacent to the middle tactor, indicating that two tactors placed 25 mm apart could not be localized (personal communication with R. Cholewiak, 2021). Based on these findings, we chose the middle of the dorsal forearm as the stimulation site.
Gescheider et al. (1970, 1982) demonstrated that the detection threshold at the thenar eminence of a lower-frequency signal increases as a function of the intensity of a narrow-band, high-frequency masking vibration. To account for the possibility of high frequencies masking mid frequencies, we measured the detection thresholds at 10 and 30 Hz in the presence of a 150 Hz or 300 Hz masker. A total of four mid-frequency detection thresholds under masking were obtained for P01 and P02 using the same three-interval, two-alternative, forced-choice, one-up two-down adaptive procedure used to measure detection thresholds at high frequencies. However, we presented stimuli in each interval following the design in (Gescheider et al., 1982). On each trial, an 800-ms high-frequency masker at 25 dB SL was present in all three intervals. In one of the randomly-selected intervals, an additional 400-ms mid-frequency target signal was added and centered in time within the 800-ms masker.
While the signal amplitudes for the high-frequency components were computed based on the detection thresholds obtained without masking, the amplitudes for the mid-frequency components were based on the detection thresholds in the presence of high-frequency maskers.
The results of d′ from the discrimination procedure for each condition are shown in Figure 4. The sensitivity indices appeared to show two trends. First, the discriminability between the broadband and the narrowband stimulus alternatives was better (higher d′) at 30 dB SL than at 20 dB SL. Second, discrimination appeared to be easier (higher d′) when the mid-frequency component was at 10 Hz, except for P02’s result at 20 dB SL for the (10,300) frequency combination.
FIGURE 4. Results of sensitivity index d′ from pairwise discrimination in Experiment 1 for the two participants: P01 in the top panel, and P02 in the bottom panel. The horizontal line indicates d′ = 1.0.
After the experiment, the participants were asked about the cues they used to perform the pairwise discrimination. They reported that 1) sometimes, one stimulus elicited a “fluttery vibration” sensation while the other felt more like a “smooth vibration”; and 2) in other times, there appeared to be a slight shift in stimulated location on the skin. These subtle cues were reported as the strategy used to solve the discrimination task on these occasions. After revisiting the experimental conditions, it was found that the “fluttery” sensation corresponded to the conditions involving the 10-Hz mid-frequency component. This was within the frequency range for fluttery vibration reported in the literature (Talbot et al., 1968; Merzenich and Harrington, 1969; Mountcastle et al., 1969; Tan et al., 1999).
To investigate the first cue, we reasoned that for the (10, 150) and (10, 300) frequency combinations at 20 and 30 dB SL, if the perceived intensity of the 10-Hz mid-frequency component was well matched to that of the high-frequency component, then the sensation should have contained both fluttery and smooth vibrations simultaneously. Whenever the fluttery or the smooth sensation dominated perception, it indicated that the mid-frequency or the high-frequency component was felt to be more intense and possibly masked its counterpart. Both cases turned out to be explainable upon further examination of the logged data.
To understand why fluttery sensation may have dominated the perception of a combined mid- and high-frequency vibration, we note that our Exp. 1 considered only the masking effects of high-frequency components on mid-frequency components, but not vice versa. Indeed most vibrotactile masking studies use high-frequency components as the maskers (Gescheider et al., 1970, 1982; Verrillo et al., 1983). However, Verrillo et al. (1983) showed evidence of a 13-Hz stimulus masking a 300-Hz stimulus at the fingertip when the amplitude of the 13-Hz stimulus exceeded the detection threshold at 300 Hz. At the relatively high intensity levels of 20 and 30 dB SL used in our Exp. 1, masking occurred in both directions of mid-to high-frequency and vice versa. This is illustrated in Figure 5 where vibrotactile thresholds in hairy skin and various intensity levels above threshold for 10, 30, 150 and 300 Hz are plotted on the same graph. It can be seen that the amplitudes of mid-frequency components were well above the detection thresholds of the high-frequency components, demonstrating mid-frequency masking of high frequencies. The amplitudes of high-frequency components were also above the detection thresholds of the mid-frequency components when set to 20 or 30 dB SL, albeit to a lesser degree. Our calibration of the mid-frequency components focused solely on possible masking of high-frequency components on mid-frequency, thereby resulting in mid-frequency amplitudes that were perhaps too high. This could explain why the flutter sensation dominated perception in conditions that included 10 Hz as the mid-frequency.
FIGURE 5. Vibrotactile thresholds on the volar forearm. The solid gray line is re-plotted from Figure 1 of (Bolanowski et al., 1994). The thresholds at 10, 30, 150, and 300 Hz are highlighted by four open circles. Filled circles, squares and triangles denote the displacement values at 13, 20 and 30 dB above threshold, respectively, for the four frequencies.
That the smooth vibration sensation dominated perception in some conditions was due to amplitude saturation of mid-frequency components that was discovered after Exp. 1. The software used in our experiment automatically capped vibration amplitudes to levels that would not exceed the power limit of the audio amps. Due to the relatively high detection thresholds at 10 Hz as compared to those at 30, 150 and 300 Hz [the masked threshold at 10 Hz was 7 dB higher than that at 30 Hz, which is significant compared to the amplitude discrimination threshold of 1.5–2.0 dB (Craig, 1972)], the lack of rigid surround [which is known to elevate detection threshold (Gescheider et al., 1978; Van Doren, 1990)], a 3–10 dB increase observed in the thresholds under masking conditions, and the relatively high signal intensities at 20–30 dB SL, we suspected that the 10-Hz vibration amplitudes may have exceeded the maximum limit under some experimental conditions. This was later confirmed, more often at 30 dB SL than at 20 dB SL. When the amplitude of the 10-Hz vibration was clipped to the maximum allowable value, two things would occur. First, the intensity of the 10-Hz mid-frequency component was reduced, thereby making the fluttery sensation less noticeable. Second, some harmonic distortions occurred which may have contributed to additional masking of the 10-Hz vibration. It was therefore conceivable that the smooth sensation of the 300-Hz high-frequency component dominated perception under these conditions. Since clipping occurred more often at 30 dB SL than at 20 dB SL, it was also expected that the pairwise discrimination was easier (higher d′) at 30 dB SL.
To remove the extraneous cues due to mutual masking of mid- and high-frequency vibrations, and amplitude clipping, respectively, the subsequent experiments adopted an intensity matching procedure and the intensity levels for all signals were reduced to 13 dB SL. Based on (Bolanowski et al., 1994), amplitudes corresponding to 13 dB SL at 150 and 300 Hz are below the detection thresholds of non-Pacinian receptors (nPC) at 10 and 30 Hz on hairy skin (see solid circles at 150 and 300 Hz in Figure 5), ensuring no masking of mid-frequency components by high-frequency vibrations [see further explanation in (Gescheider et al., 1982)]. However, since the detection thresholds at 10 and 30 Hz are higher than those at 150 and 300 Hz (Bolanowski et al., 1994), it would not be possible to choose a signal amplitude at the mid frequencies that would not activate the Pacinian channel.
To investigate the second cue of a possible shift in perceived simulation site, it should be noted that most studies of two-point discrimination threshold were conducted with touch/pressure rather than vibrotactile stimuli [although see (Cholewiak and Collins, 2003)]. Among the former method, even though Weber (1834/1978) and Weinstein (1968) reported a 40-mm two-point limen on the forearm, a more recent study by Mancini et al. (2014) reported a two-point touch threshold of 22 mm which was below the center-to-center distance of the two tactors used in Exp. 1. Similarly, Lévêque et al. (2000) reported a two-point gap discrimination of 21.23 mm on the volar forearm. Craig and Johnson (2000) explained why the two-point limen is not a good measure of tactile spatial resolution, and pointed to several potential confounds that exist in its estimates. Using vibrotactile stimuli, van Erp (2005b) conducted an experiment on vibrotactile spatial acuity by activating pairs of tactors in a linear array at different locations of the torso with 28-ms sine waves at 250 Hz. The results showed a uniform acuity of 20–30 mm except for arrays oriented horizontally and placed on the body midline. Perez et al. (2000) used an array of 16 piezoelectric vibrators with a thickness of 0.5 mm spaced by 1 mm and delivered waveforms composed of bursts of rectangular pulses to the index finger. They activated pairs of vibrators simultaneously and used the method of limits to determine the two-point threshold. They found that the threshold increases from 2.1 to 5.1 mm as the pulse repetition period decreases from 1/25 to 1/500 s. This was similar to the finding by Weinstein (1968) who reported values of ≈4 mm at the index finger. It thus appears that spatial resolution improves from forearm to the fingertips. At more proximal locations such as the upper arm, the two-point limen was reported to be 44 and 67 mm by Weinstein (1968) and Weber (1834/1978), respectively. Since the minimum center-to-center distance of the two tactors used in the present study needed to be ≈30 mm to avoid direct contact, it was necessary to move the stimulation site to a more proximal location with poorer spatial acuity–the upper arm. It was also important to avoid any perceptual anchors that can be easily localized [see (Cholewiak and Collins, 2003)] and to avoid bone conduction that might elicit auditory sensations (Kaufmann et al., 2012). Therefore, the fleshy surface atop the biceps on the left upper arm was chosen as the stimulation site for subsequent experiments.
The experimental methods for Exp. 2 were similar to those for Exp. 1, except for a few modifications noted below.
There were four conditions in Exp. 2, based on the combinations of two mid frequencies (10, 30 Hz) and two high frequencies (150, 300 Hz). Unlike Exp. 1, only one signal intensity of 13 dB SL was used.
The tactor band was fastened around the participant’s left upper arm so that the tactors rested on the biceps muscle.
Detection thresholds for the four frequencies were measured in isolation using the same three-interval, two-alternative, forced-choice, one-up two-down adaptive procedure employed in Exp. 1. This was followed by an intensity matching procedure to calibrate the amplitudes of mid frequencies for each of the four experimental conditions. For each frequency combination, the broadband dual-frequency stimulus (the reference) used 13 dB SL (dB above detection threshold in isolation) for both the mid- and high-frequency amplitudes. For the two single-frequency stimulus alternatives (the narrowband comparison), the high-frequency amplitude was also set to 13 dB SL. The amplitude of the mid-frequency component could be changed by the participant using the method of adjustment (Jones and Tan, 2013). The participant felt a sequence of three signals in the order reference-comparison-reference, and adjusted the amplitude of the mid-frequency component until the comparison stimulus felt similar to the reference. The calibrated amplitudes were then used in the subsequent pairwise discrimination procedure.
The results of Exp. 2 are shown in two plots. First, the adjusted amplitudes of the mid-frequency components are shown in Figure 6 after they have been converted to dB SL. The dashed line corresponds to 13 dB SL that was used with both components of the broadband stimuli and the high-frequency components of the narrowband stimuli. It can be seen that the adjusted intensity level for P01 stayed within ±1 dB around 13 dB SL, a difference that is below the 1.5–2.0 dB amplitude discrimination threshold for vibrotactile stimuli (Craig, 1972). The same was true for P02 for the two conditions where the mid-frequency was at 10 Hz. The adjusted amplitudes for the 30-Hz mid-frequency component was significantly below 13 dB SL for P02.
FIGURE 6. Adjusted amplitudes of mid frequencies in dB SL from intensity matching in Exp. 2. The horizontal dashed line denotes 13 dB SL.
Second, the d′ values from pairwise comparison for each condition are shown in Figure 7 for the two participants in separate panels. Participant P01 could not distinguish the broadband and narrowband stimuli, as demonstrated by |d′| < 1.0 in all conditions. While participant P02 showed poor discrimination for the two conditions (30,150) and (30,300), the d′ values for (10,150) and (10,300) were well above 1.0. The participant reported using the “fluttery vs. smooth” cue for pairwise comparison in the latter two conditions. Note that the d′ values for P02 when the mid-frequency was at 10 Hz were lower than those in Exp. 1 (see Figure 4).
FIGURE 7. Sensitivity index d′ values from pairwise comparison in Exp. 2. The horizontal line indicates the performance criterion for discrimination at d′ = 1.0.
An examination of logged data did not reveal a large difference in the detection thresholds between the two participants and the thresholds were more than 13 dB below the maximum allowable output (−16.7 ± 1.9 dB for P01; −15.8 ± 2.3 dB for P02; relative to maximum amplitudes). It was noticed however that the perceived intensity of the fluttery sensation due to the 10-Hz mid-frequency component changed noticeably if the tactor band was wrapped very tight around the upper arm. We reasoned that the diaphragm of the speaker could be damped by the pressure exerted by the Velcro band, especially when the displacement was high as was the case with the 10-Hz vibration. Moreover, the discriminability of signals containing 10 Hz could be related to the “borderline” characteristics of this frequency. As described by Tan et al. (1999), 10 Hz is located at the boundary between the perception of slow movement and rough/fluttery vibration. From a practical point of view, few resonance-type tactors on the market can produce discernible vibrations at 10 Hz. Therefore, we replaced the 10-Hz mid-frequency component with a 60-Hz mid-frequency component in Exp. 3 where all 12 participants were tested, including P01 and P02.
Now that we have eliminated the extraneous cues that could contribute to the discriminability of one broadband, dual-frequency signal and two simultaneous narrowband single-frequency signals, the objective of Exp. 3 was to conduct the pairwise comparison with a large number of participants (N = 12). They included the two participants from Exp. 1 and Exp. 2 and 10 additional naive participants.
In this experiment, the two tactors were placed atop the bicep muscle on the left upper arm. There were four experimental conditions denoted by the mid- and high-frequency pairs in Hz (30,150), (30,300), (60,150) and (60,300). The procedure was the same as that in Exp. 2. Each participant was tested for detection thresholds at the four frequencies, performed intensity matching, and completed pairwise comparison of narrowband and broadband stimuli. Per additional IRB guidelines for conducting human experiments during the COVID-19 pandemic, a webcam, a microphone and TeamViewer software were installed to allow the experimenter to control programs and to monitor and communicate with participants from an adjacent room (see Figure 3).
The intensity matching results for all 12 participants are presented as box plots in Figure 8. The figure shows the distributions of the adjusted amplitudes of the single-frequency vibrations for the mid frequencies and the four experimental conditions. The adjustments are compared to the 13 dB SL amplitude level used with both components of the broadband stimuli and the high-frequency component of narrowband stimuli. As shown, there is a wide range of adjusted amplitudes with some outliers at conditions (30,300) and (60,300). However, a one-way ANOVA did not indicate a significant difference between adjustments among the four experimental conditions (F (2, 44) = 1.02, p = 0.39). In addition, individual t-tests were used to compare the intensity matching results with the 13 dB SL reference per condition. The results did not show a significant difference between the adjusted amplitudes and 13 dB SL for any condition [t (11) = −1.79, p = 0.10 for condition (30, 150); t (11) = −1.58, p = 0.14 for (30, 300); t (11) = −0.88, p = 0.39 for (60, 150); t (11) = −0.05, p = 0.96 for (60, 300)]. The variability of the data shown in Figure 8 suggests that intensity matching was necessary for individual participants.
FIGURE 8. Results of adjusted mid-frequency amplitudes from intensity matching. The horizontal dashed line corresponds to 13 dB SL.
The d′ values from pairwise discrimination are shown in Figure 9. In general, the 4 d′ values for the four experimental conditions were all between 0 and 1, indicating that the participants could not reliably distinguish between a dual-frequency vibration delivered by one tactor and two corresponding single-frequency vibrations delivered by two adjacent tactors. The d′ values were compared to the threshold value of 1.0 via individual t-tests per condition. The results revealed that d′ values were significantly below 1.0 across all four conditions [t (11) = −4.62 for condition (30, 150); t (11) = −4.80 for (30, 300); t (11) = −3.46 for (60, 150); t (11) = −5.88 for (60, 300); all with p < 0.01]. Furthermore, a one-way ANOVA indicated no significant differences among the 4 d′ values (F (3, 44) = 0.73, p = 0.54). The response biases, c, were relatively small, ranging from −0.16 to −0.04 for the four conditions. These results demonstrate clearly that with the removal of extraneous cues, it was feasible to achieve a broadband vibration (i.e., containing mid- and high-frequency components) with two narrowband vibrations (one mid-frequency and one high-frequency) delivered by two tactors placed in close proximity.
FIGURE 9. Results of sensitivity index d′ from pairwise discrimination in Exp. 3. The dashed horizontal line indicates d′ = 1.0. Error bars denote ±1 std.err.
Our findings that the d′ values under all experimental conditions in Experiment 3 were well below 1.0 provides psychophysical validation that broadband haptic effects can be achieved by interleaving narrowband vibrotactile stimuli, when narrowband tactors are placed within the two-point limen on the skin and signal amplitudes are properly calibrated. This result can be used to guide the design of wearable tactile displays that interleave narrowband tactors. For example, a body site can be divided into distinct stimulation areas defined by the two-point limen, as illustrated by the circular grid on the back and the diamond grid on the forearm in Figure 10. The exact shape of the grid element is not important, as long as 1) tactors within each area are felt as one location and 2) tactors in different areas are felt as two locations. The left panel in Figure 10 provides an example of a haptic back display with three types of tactors in each distinct stimulation area. The right panel shows one variant where low-frequency tactors are placed along the middle of the forearm to deliver, for example, pleasant strokings using the signal patterns described in (Culbertson et al., 2018). The rest of the grid contains two parallel columns of mid- and high-frequency tactors that can be used to encode English phonemes using haptic codes similar to those in (Reed et al., 2019). Such wearable displays can differ in many ways including body site stimulated, layout of distinct stimulation areas, number and type of tactors within each stimulation area, waveforms and signal activation patterns. The distinct sensations delivered by different types of tactors can be used to encode near and far in an immersive VR game, imminent danger versus surrounding traffic to a visually-impaired pedestrian, or speed-up vs. slow-down cues in an AR workout app.
FIGURE 10. Illustration of interleaving narrowband tactors in a wearable haptic display worn on the back or volar forearm. The large circles on the back and the diamonds on the forearm indicate spatially distinct areas of stimulation. The legend shows the three types of narrowband tactors. The table at the bottom lists the two-point limens at select body sites (from (Weber, 1834/1978; Weinstein, 1968; Mancini et al., 2014)).
A question that naturally arises is what to do when a skin area has dense nerve innervation and hence high spatial resolution, such as the fingertip. It is unlikely to find tactors that can be squeezed into a scale on the order of millimeters (Weinstein, 1968), except for pin arrays such as the Optacon (Linvill and Bliss, 1966) and the 400-probe display (Pawluk et al., 1998). However, at very small contactor areas (below 0.08 cm2), vibrotactile detection thresholds increase significantly and flatten across the entire vibrotactile frequency range [see Figure 7 of (Verrillo, 1963)]. As a result, perception of vibrotactile stimuli at suprathreshold levels will involve several mechanoreceptor populations. As shown by the results of experiments 2 and 3, our efforts to calibrate signal amplitudes are necessary due to the need to balance the perceived intensities associated with multiple mechanoreceptor channels. This would not be possible with very small contact areas where the detection thresholds are the same for all frequencies. Evidence of this issue is available in studies that investigate localization of low- and high-frequency vibrotactile stimuli. Rogers (1970) showed better localization of a 250-Hz stimulus compared to a 10-Hz stimulus using 1-mm-diameter probes at the finger tip, even though the Pacinian receptors (most sensitive at 250 Hz) have larger receptive fields than the non-Pacinian receptors responsible for perception at 10 Hz. It was likely that the 1-mm-diameter probes excited more mechanoreceptors than just the Pacinian corpuscles. In another study, Sherrick et al. (1990) used two 6-mm-diameter contactors in a series of four experiments to study localization. They found worse localization with 250-Hz sinusoidal stimuli than with 25-Hz stimuli in the proximal area of the metacarpus (closest to the wrist), but equal localization accuracy at the two frequencies in the distal area of the metacarpus (closest to the little finger). These findings demonstrate the difficulties that arise when trying to dissociate mechanoreceptor populations in the hand using small contactors, complicating the interpretation of experimental results. Therefore, it remains to be seen whether the approach proposed in the present study can be readily applied to the skin on the hand given the difficulty of eliciting distinct sensations such as flutter and smooth vibration. Furthermore, in most use scenarios, it would be desirable to place wearable haptic displays on the torso and limbs because the user’s hand would likely be engaged in text input and menu selection tasks.
Given that the dimensions of tactors plays a key role in the selection of the stimulation site (according to the two-point limen constraint), we might wonder if vertically stacking them instead of placing them in adjacent locations is a viable way to address areas with high spatial resolution. This would seem to remove the restriction of placing tactors within the two-point limen. However, direct contact between mechanically-coupled stacked actuators would likely result in a distorted waveform. This would be owing to the difficult-to-control dynamic effects of the additional loading on each tactor. Hence, the waveforms delivered to the skin would neither be clean, independently-delivered narrowband stimuli, nor a simple broadband stimulus composed of the sum of the independent narrowband signals. This was confirmed with acceleration measurements on the two LRA tactors under several conditions. The tactors were vertically stacked and actuated with sinusoidal signals at 60, 150, and 300 Hz. We measured the acceleration at the top of the stack for all possible combinations of frequencies and configurations of the two tactors. We observed that the response was always dominated by high frequencies, especially when delivered to the tactor closest to the accelerometer. Thus, the idea of stacking actuators to achieve broadband effects appears to be impractical.
Our work also constitutes an effort to optimize the design decisions for vibrotactile actuator manufacturing. The possibility of presenting complex haptic effects with a few narrowband signals eliminates the need for a complex and costly broadband actuator. In practice, the replacement of a single actuator with multiple interleaved narrowband actuators could represent a potential overhead in circuit complexity and mounting difficulties. Nevertheless, these complications can be resolved with better product designs. Commercially-available narrowband actuators such as LRA’s are sufficiently small, cost effective and power efficient. Therefore, the advantages of interleaving narrowband actuators outweigh the trade-offs.
We hope that our approach serves as an example of how psychophysical validation and perception experiments can guide the design of new actuators. Another example of this approach is the work by Friesen et al. (2018), who asked participants to adjust the amplitude and frequency of a signal on a variable friction display to match the perception of a two-frequency reference stimulus. They demonstrated that participants matched a tactile pitch whose frequency is a function of the frequencies and amplitudes in the reference stimulus. This shows that a target texture can be achieved without a complex representation of the texture on a surface friction display.
In conclusion, we proposed, tested and validated a new way of achieving rich, broadband haptic effects by interleaving narrowband vibrotactile stimuli on the skin. We provide guidelines and examples of applying this approach in creating new wearable consumer products. Whereas the experiments in the present study used a relatively large broadband tactor, most commercially-available tactors are smaller in size (e.g., a footprint of 1 cm × 1 cm or less in mobile phones). It is therefore possible to place one mid-frequency and one high-frequency resonant tactors within the two-point limen on the skin using tactors that are on the market today. Our work also contributes to future development of tactors by providing perception-based specifications on frequency range and tactor dimensions.
The raw data supporting the conclusion of this article will be made available by the authors, without undue reservation.
The studies involving human participants were reviewed and approved by Purdue Institutional Review Board. The patients/participants provided their written informed consent to participate in this study.
All authors contributed to the design of all experiments, as well as writing, revising and approving the manuscript for submission. JM was responsible for conducting the three experiments, collecting and analysing the data.
This research was supported by a Google Faculty Research Award 2019 and Grant No. 1954842-IIS from the National Science Foundation.
The authors declare that the research was conducted in the absence of any commercial or financial relationships that could be construed as a potential conflict of interest.
All claims expressed in this article are solely those of the authors and do not necessarily represent those of their affiliated organizations or those of the publisher, the editors, and the reviewers. Any product that may be evaluated in this article, or claim that may be made by its manufacturer, is not guaranteed or endorsed by the publisher.
The content of this manuscript has been presented (in part) at the IEEE World Haptics Conference 2021, (Martinez et al., 2021).
The Supplementary Material for this article can be found online at: https://www.frontiersin.org/articles/10.3389/frvir.2022.894575/full#supplementary-material
Bolanowski, S. J., Gescheider, G. A., and Verrillo, R. T. (1994). Hairy Skin: Psychophysical Channels and Their Physiological Substrates. Somatosens. Mot. Res. 11, 279–290. doi:10.3109/08990229409051395
Brown, L. M., Brewster, S. A., and Purchase, H. C. (2006). “Multidimensional Tactons for Non-visual Information Presentation in Mobile Devices,” in Proceedings of the ACM International Conference on Human-Computer Interaction with Mobile Devices and Services (MobileHCI) (New York, NY: ACM), 231–238. doi:10.1145/1152215.1152265
Chen, H.-Y., Santos, J., Graves, M., Kim, K., and Tan, H. Z. (2008). Tactor Localization at the Wrist. Proc. EuroHaptics 2008 LNCS 5024, 209–218.
Choi, S., and Kuchenbecker, K. J. (2012). Vibrotactile Display: Perception, Technology, and Applications. Proc. IEEE 101, 2093–2104.
Cholewiak, R. W., Brill, J. C., and Schwab, A. (2004). Vibrotactile Localization on the Abdomen: Effects of Place and Space. Percept. Psychophys. 66, 970–987. doi:10.3758/bf03194989
Cholewiak, R. W., and Collins, A. A. (2000). The Generation of Vibrotactile Patterns on a Linear Array: Influences of Body Site, Time, and Presentation Mode. Percept. Psychophys. 62, 1220–1235. doi:10.3758/bf03212124
Cholewiak, R. W., and Collins, A. A. (2003). Vibrotactile Localization on the Arm: Effects of Place, Space, and Age. Percept. Psychophys. 65, 1058–1077. doi:10.3758/bf03194834
Cholewiak, R. W., and McGrath, C. (2006). Vibrotactile Targeting in Multimodal Systems: Accuracy and Interaction. Proc. IEEE Haptics Symposium 2006, 413–420.
Cholewiak, S. A., Kwangtaek Kim, K., Tan, H. Z., and Adelstein, B. D. (2010). A Frequency-Domain Analysis of Haptic Gratings. IEEE Trans. Haptics 3, 3–14. doi:10.1109/toh.2009.36
Craig, J. C. (1972). Difference Threshold for Intensity of Tactile Stimuli. Percept. Psychophys. 11, 150–152. doi:10.3758/bf03210362
Craig, J. C., and Johnson, K. O. (2000). The Two-Point Threshold. Curr. Dir. Psychol. Sci. 9, 29–32. doi:10.1111/1467-8721.00054
Culbertson, H., Nunez, C. M., Israr, A., Lau, F., Abnousi, F., and Okamura, A. M. (2018). A Social Haptic Device to Create Continuous Lateral Motion Using Sequential Normal Indentation. Proc. IEEE Haptics Symposium 2018, 32–39. doi:10.1109/haptics.2018.8357149
Eberhardt, S. P., Bernstein, L., Barac-Cikoja, D., Coulter, D., and Jordan, J. (1994). Inducing Dynamic Haptic Perception by the Hand: System Description and Some Results. Proc. ASME Dyn. Syst. Control 55, 345–351.
Elliott, L. R., Mortimer, B. J. P., Cholewiak, R. W., Mort, G. R., Zets, G. A., and Pittman, R. (2013). “Development of Dual Tactor Capability for a Soldier Multisensory Navigation and Communication System,” in International Conference on Human Interface and the Management of Information (IEEE), 46–55. doi:10.1007/978-3-642-39215-3_6
Ertan, S., Lee, C., Willets, A., Tan, H. Z., and Pentland, A. (1998). “A Wearable Haptic Navigation Guidance System,” in Digest of the Second International Symposium on Wearable Computers (Pittsburgh, PA, USA: IEEE), 164–165.
Franzén, O., and Nordmark, J. (1975). Vibrotactile Frequency Discrimination. Percept. Psychophys. 17, 480–484. doi:10.3758/bf03203298
Friesen, R. F., Klatzky, R. L., Peshkin, M. A., and Colgate, J. E. (2018). “Single Pitch Perception of Multi-Frequency Textures,” in 2018 IEEE Haptics Symposium (HAPTICS) (IEEE), 290–295. doi:10.1109/haptics.2018.8357190
Gescheider, G. A., Capraro, A. J., Frisina, R. D., Hamer, R. D., and Verrillo, R. T. (1978). The Effects of a Surround on Vibrotactile Thresholds. Sens. Process. 2, 99–115.
Gescheider, G. A., Herman, D. D., and Phillips, J. N. (1970). Criterion Shifts in the Measurement of Tactile Masking. Percept. Psychophys. 8, 433–436. doi:10.3758/bf03207041
Gescheider, G. A., Verrillo, R. T., and Van Doren, C. L. (1982). Prediction of Vibrotactile Masking Functions. J. Acoust. Soc. Am. 72, 1421–1426. doi:10.1121/1.388449
Goff, G. D. (1967). Differential Discrimination of Frequency of Cutaneous Mechanical Vibration. J. Exp. Psychol. 74, 294–299. doi:10.1037/h0024561
Hayward, V., and MacLean, K. (2007). Do it Yourself Haptics: Part I. IEEE Robot. Autom. Mag. 14, 88–104. doi:10.1109/m-ra.2007.907921
Hsieh, M. J., Liang, R. H., and Chen, B. Y. (2016). “NailTactors: Eyes-free Spatial Output Using a Nail-Mounted Tactor Array,” in Proceedings of the ACM International Conference on Human-Computer Interaction with Mobile Devices and Services (MobileHCI) (IEEE), 29–34.
Israr, A., and Poupyrev, I. (2011). “Tactile Brush: Drawing on Skin with a Tactile Grid Display,” in Proceedings of the SIGCHI Conference on Human Factors in Computing Systems (IEEE), 2019–2028.
Israr, A., Tan, H. Z., and Reed, C. M. (2006). Frequency and Amplitude Discrimination along the Kinesthetic-Cutaneous Continuum in the Presence of Masking Stimuli. J. Acoust. Soc. Am. 120, 2789–2800. doi:10.1121/1.2354022
Jones, L. A., Kunkel, J., and Piateski, E. (2009). Vibrotactile Pattern Recognition on the Arm and Back. Perception 38, 52–68. doi:10.1068/p5914
Jones, L. A., and Sarter, N. B. (2008). Tactile Displays: Guidance for Their Design and Application. Hum. Factors 50, 90–111. doi:10.1518/001872008x250638
Jones, L. A., and Tan, H. Z. (2013). Application of Psychophysical Techniques to Haptic Research. IEEE Trans. Haptics 6, 268–284. doi:10.1109/toh.2012.74
Kaufmann, M., Adelman, C., and Sohmer, H. (2012). Mapping Sites on Bone and Soft Tissue of the Head, Neck and Thorax at Which a Bone Vibrator Elicits Auditory Sensation. Audiol. Neurotol. Extra 2, 9–15. doi:10.1159/000336159
Lee, S. C., and Starner, T. (2010). “BuzzWear: Alert Perception in Wearable Tactile Displays on the Wrist,” in Proceedings of CHI 2010: Computng on the Body (IEEE), 433–442.
Lévêque, J.-L., Dresler, J., Ribot-Ciscar, E., Roll, J.-P., and Poelman, C. (2000). Changes in Tactile Spatial Discrimination and Cutaneous Coding Properties by Skin Hydration in the Elderly. J. Investigative Dermatology 115, 454–458. doi:10.1046/j.1523-1747.2000.00055.x
Levitt, H. (1971). Transformed Up‐Down Methods in Psychoacoustics. J. Acoust. Soc. Am. 49, 467–477. doi:10.1121/1.1912375
Liao, Y.-C., Chen, Y.-L., Lo, J.-Y., Liang, R.-H., Chan, L., and Chen, B.-Y. (2016). “EdgeVib: Effective Alphanumeric Character Output Using a Wrist-Worn Tactile Display,” in Proceedings of the 29th Annual Symposium on User Interface Software and Technology (IEEE), 595–601.
Linvill, J. G., and Bliss, J. C. (1966). A Direct Translation Reading Aid for the Blind. Proc. IEEE 54, 40–51. doi:10.1109/proc.1966.4572
Macmillan, N. A., and Creelman, C. D. (2004). Detection Theory: A User’s Guide. Lawrence Erlbaum Associates.
Mahns, D. A., Perkins, N. M., Sahai, V., Robinson, L., and Rowe, M. J. (2006). Vibrotactile Frequency Discrimination in Human Hairy Skin. J. Neurophysiology 95, 1442–1450. doi:10.1152/jn.00483.2005
Makous, J., Friedman, R., and Vierck, C. (1995). A Critical Band Filter in Touch. J. Neurosci. 15, 2808–2818. doi:10.1523/jneurosci.15-04-02808.1995
Mancini, F., Bauleo, A., Cole, J., Lui, F., Porro, C. A., Haggard, P., et al. (2014). Whole-body Mapping of Spatial Acuity for Pain and Touch. Ann. Neurol. 75, 917–924. doi:10.1002/ana.24179
Marks, L. E. (1979). Summation of Vibrotactile Intensity: An Analog to Auditory Critical Bands? Sens. Process. 3, 188–203.
Martinez, J. S., Tan, H. Z., and Cholewiak, R. W. (2021). “Psychophysical Validation of Interleaving Narrowband Tactile Stimuli to Achieve Broadband Effects,” in 2021 IEEE World Haptics Conference (WHC) (IEEE), 709–714. doi:10.1109/WHC49131.2021.9517268
Matscheko, M., Ferscha, A., Riener, A., and Lehner, M. (2010). Tactor Placement in Wrist Worn Wearables. Int. Symposium Wearable Comput. 2010, 1–8. doi:10.1109/iswc.2010.5665867
Merzenich, M. M., and Harrington, T. H. (1969). The Sense of Flutter-Vibration Evoked by Stimulation of the Hairy Skin of Primates: Comparison of Human Sensory Capacity with the Responses of Mechanoreceptive Afferents Innervating the Hairy Skin of Monkeys. Exp. Brain Res. 9, 236–260. doi:10.1007/BF00234457
Mountcastle, V. B., Talbot, W. H., Sakata, H., and Hyvärinen, J. (1969). Cortical Neuronal Mechanisms in Flutter-Vibration Studied in Unanesthetized Monkeys. Neuronal Periodicity and Frequency Discrimination. J. Neurophysiology 32, 452–484. doi:10.1152/jn.1969.32.3.452
Park, G., Cha, H., and Choi, S. (2019). Haptic Enchanters: Attachable and Detachable Vibrotactile Modules and Their Advantages. IEEE Trans. Haptics 12, 43–55. doi:10.1109/toh.2018.2859955
Park, G., and Choi, S. (2011). “Perceptual Space of Amplitude-Modulated Vibrotactile Stimuli,” in 2011 IEEE World Haptics Conference (IEEE), 59–64. doi:10.1109/whc.2011.5945462
Park, J., Kim, J., Oh, Y., and Tan, H. Z. (2016). Rendering Moving Tactile Stroke on the Palm Using a Sparse 2d Array. Proc. EuroHaptics 2016, 47–56. doi:10.1007/978-3-319-42321-0_5
Pawluk, D. T. V., Buskirk, C. P., Killebrew, J. H., Hsiao, S. S., and Johnson, K. O. (1998). Control and Pattern Specification for a High Density Tactile Array. Proc. ASME Dyn. Syst. Control Div. 64, 97–102. doi:10.1115/imece1998-0239
Perez, C. A., Holzmann, C. A., and Jaeschke, H. E. (2000). Two-point Vibrotactile Discrimination Related to Parameters of Pulse Burst Stimulus. Med. Biol. Eng. Comput. 38, 74–79. doi:10.1007/bf02344692
Reed, C. M., Tan, H. Z., Perez, Z. D., Wilson, E. C., Severgnini, F. M., Jung, J., et al. (2019). A Phonemic-Based Tactile Display for Speech Communication. IEEE Trans. Haptics 12, 2–17. doi:10.1109/TOH.2018.2861010
Rogers, C. (1970). Choice of Stimulator Frequency for Tactile Arrays. IEEE Trans. Man. Mach. Syst. 11, 5–11. doi:10.1109/tmms.1970.299954
Rupert, A. H. (2000). An Instrumentation Solution for Reducing Spatial Disorientation Mishaps. IEEE Eng. Med. Biol. Mag. 19, 71–80. doi:10.1109/51.827409
Sherrick, C. E., Cholewiak, R. W., and Collins, A. A. (1990). The Localization of Low‐ and High‐frequency Vibrotactile Stimuli. J. Acoust. Soc. Am. 88, 169–179. doi:10.1121/1.399937
Shim, S.-W., and Tan, H. Z. (2020). palmScape: Calm and Pleasant Vibrotactile Signals. Proc. HCI Int. 2020 LNCS 12200, 1–17. doi:10.1007/978-3-030-49713-2_37
Talbot, W. H., Darian-Smith, I., Kornhuber, H. H., and Mountcastle, V. B. (1968). The Sense of Flutter-Vibration: Comparison of the Human Capacity with Response Patterns of Mechanoreceptive Afferents from the Monkey Hand. J. Neurophysiology 31, 301–334. doi:10.1152/jn.1968.31.2.301
Tan, H. Z., Choi, S., Lau, F. W. Y., and Abnousi, F. (2020a). Methodology for Maximizing Information Transmission of Haptic Devices: A Survey. Proc. IEEE 108, 945–965. doi:10.1109/jproc.2020.2992561
Tan, H. Z., Durlach, N. I., Reed, C. M., and Rabinowitz, W. M. (1999). Information Transmission with a Multifinger Tactual Display. Percept. Psychophys. 61, 993–1008. doi:10.3758/bf03207608
Tan, H. Z., and Rabinowitz, W. M. (1996). “A New Multi-Finger Tactual Display,” in Proceedings of the ASME Dynamic Systems and Control Division (New York, NY: ASME). doi:10.1121/1.415560
Tan, H. Z., Reed, C. M., Jiao, Y., Perez, Z. D., Wilson, E. C., Jung, J., et al. (2020b). Acquisition of 500 English Words through a TActile Phonemic Sleeve (TAPS). IEEE Trans. Haptics 13, 745–760. doi:10.1109/TOH.2020.2973135
Van Doren, C. L. (1990). The Effects of a Surround on Vibrotactile Thresholds: Evidence for Spatial and Temporal Independence in the non‐Pacinian I (NP I) Channel. J. Acoust. Soc. Am. 87, 2655–2661. doi:10.1121/1.399550
van Erp, J. B. F. (2005b). “Vibrotactile Spatial Acuity on the Torso: Effects of Location and Timing Parameters,” in First Joint Eurohaptics Conference and Symposium on Haptic Interfaces for Virtual Environment and Teleoperator Systems (New York, NY: World Haptics Conference), 80–85.
Van Erp, J. B. (2005a). Presenting Directions with a Vibrotactile Torso Display. Ergonomics 48, 302–313. doi:10.1080/0014013042000327670
Verrillo, R. T. (1963). Effect of Contactor Area on the Vibrotactile Threshold. J. Acoust. Soc. Am. 35, 1962–1966. doi:10.1121/1.1918868
Verrillo, R. T., Fraioli, A. J., and Smith, R. L. (1969). Sensation Magnitude of Vibrotactile Stimuli. Percept. Psychophys. 6, 366–372. doi:10.3758/bf03212793
Verrillo, R. T., Gescheider, G. A., Calman, B. G., and Van Doren, C. L. (1983). Vibrotactile Masking: Effects of Oneand Two-Site Stimulation. Percept. Psychophys. 33, 379–387. doi:10.3758/bf03205886
Verrillo, R. T., and Gescheider, G. A. (1992). “Perception via the Sense of Touch,” in Tactile Aids for the Hearing Impaired (Londong, England: Whurr Publishers), 1–36. chap. 1.
Weinstein, A. (1968). The Bonanza King Myth: Western Mine Owners and the Remonetization of Silver. Bus. Hist. Rev. 42, 195–218. doi:10.2307/3112215
Keywords: actuator, broadband, haptic, narrowband, psychophysics, tactile, validation, vibrotactile
Citation: Martinez JS, Tan HZ and Cholewiak RW (2022) Psychophysical Studies of Interleaving Narrowband Tactile Stimuli to Achieve Broadband Perceptual Effects. Front. Virtual Real. 3:894575. doi: 10.3389/frvir.2022.894575
Received: 11 March 2022; Accepted: 25 April 2022;
Published: 31 May 2022.
Edited by:
Jin Ryong Kim, The University of Texas at Dallas, United StatesReviewed by:
Seokhee Jeon, Kyung Hee University, South KoreaCopyright © 2022 Martinez, Tan and Cholewiak. This is an open-access article distributed under the terms of the Creative Commons Attribution License (CC BY). The use, distribution or reproduction in other forums is permitted, provided the original author(s) and the copyright owner(s) are credited and that the original publication in this journal is cited, in accordance with accepted academic practice. No use, distribution or reproduction is permitted which does not comply with these terms.
*Correspondence: Juan S. Martinez, bWFydDEzMDRAcHVyZHVlLmVkdQ==
Disclaimer: All claims expressed in this article are solely those of the authors and do not necessarily represent those of their affiliated organizations, or those of the publisher, the editors and the reviewers. Any product that may be evaluated in this article or claim that may be made by its manufacturer is not guaranteed or endorsed by the publisher.
Research integrity at Frontiers
Learn more about the work of our research integrity team to safeguard the quality of each article we publish.