- 1Department of Radiology and Biomedical Imaging, University of California, San Francisco, San Francisco, CA, United States
- 2Division of Pediatric Orthopedic Surgery, Department of Orthopedic Surgery, Benioff Children's Hospital, University of California, San Francisco, San Francisco, CA, United States
- 3Division of Pediatric Orthopedic Surgery, Department of Orthopedic Surgery, Benioff Children's Hospital-Oakland, University of California, San Francisco, Oakland, CA, United States
- 4Department of Radiology and Biomedical Imaging, Benioff Children's Hospital, University of California, San Francisco, San Francisco, CA, United States
Objectives: We retrospectively assess the potential impact of a novel, investigational Augmented Reality (AR) software application, Radiology with Holographic Augmentation (RadHA), on pediatric orthopedic surgeon's confidence in surgical planning, hardware selection, hardware fit, and estimated potential intraoperative time savings in the setting of complex adolescent elbow fractures.
Methods: After study selection, 12 individual cases of complex elbow fractures in adolescent pediatric patients were identified for review. AR models were generated for each case derived from the patient's CT. Five fellowship-trained pediatric orthopedic surgeons reviewed each case for a total of 60 separate observations. Surgeons reviewed clinical data, radiologic imaging, and AR models and then answered Likert Scale questions on measures of confidence in presurgical planning and projected potential time savings. These data were reviewed and analyzed using various statistical tools.
Results: Surgeons reported high confidence in the quality of the AR models created. Additionally, surgeons reported increased confidence in their surgical plan, increased confidence in hardware selection, and increased confidence in hardware fit. Within the sub-analysis of complex (comminuted) fractures, surgeons reported greater expected increases in confidence of their surgical plan and hardware fit. Overall, surgeons estimated potential intraoperative time savings, averaging 17.3 min for all fracture types and 17.6 min for complex fractures.
Conclusions: Preoperative planning using AR-based models can increase surgeon confidence in preoperative planning, hardware selection, and confidence in hardware fit.
Highlights
– Evaluated the utility of AR holograms in pre-operative planning within the use of case of pediatric elbow fractures.
– Characterized the potential impact of AR on key measures, such as surgeon confidence and potential operative time savings.
Introduction
Repair of complex fractures in the pediatric orthopedic patient can be challenging, as often the exact type and approach of repair is dependent on the degree, pattern, and relationships of osseous fragmentation (Houshian et al., 2001; Abzug and Herman, 2012). Distal humeral fracture patterns, in particular, differ in adolescent patients, who often demonstrate a more complex “adult-type” fracture, compared to juveniles, who are more likely to have a straightforward supracondylar, lateral condyle, or medial epicondyle fracture pattern, all of which have standard treatment algorithms. These “adult-type” adolescent distal humerus fractures may require open reduction and internal fixation with plating, possible olecranon osteotomy; more typical juvenile patterns often require no more than closed reduction and pin fixation (Handelsman et al., 2006; Howard et al., 2012). For complex intra-articular cases, cross-sectional imaging plays an integral role in preoperative workup, including evaluating fracture patterns, determining a specific surgical approach, and selecting appropriate hardware (Koh et al., 2010; Park et al., 2017). The addition of three-dimensional (3D) imaging has been shown to be of potential benefit in other areas of orthopedic surgical planning (Wicky et al., 2000; Schepers et al., 2016; Fotouhi et al., 2018). However, conventional 3D reconstructions displayed on a two-dimensional flat screen may not always provide sufficient demonstration of complex, comminuted fractures and the relationships of various fracture fragments (Atesok and Schemitsch, 2010). These reformations are typically generated by the CT technologist and are limited to arbitrary planes of rotation, often not demonstrating the relevant plane the orthopedic surgeon requires for operative planning. Furthermore, these reconstructions are typically performed at the time of acquisition, and generating post-hoc reconstructions can be challenging and cumbersome.
Interventions that can help reduce operative time and increase surgeon confidence are of utmost importance in the pediatric patient. Within healthcare, a variety of different technologies have been developed to help physicians leverage patient-specific imaging (Wicky et al., 2000; Schepers et al., 2016). These include the utilization of 3D reconstructions that are usually provided at the time of image acquisition, 3D printing, and digital technologies such as Virtual Reality (VR), Augmented Reality (AR), and other types of Mixed Reality (MR) visualizations, where digital information is displayed on varying levels of digital backgrounds. Augmented Reality has been utilized in a variety of different applications, including military, industry, and increasingly, within healthcare (Fotouhi et al., 2018; Wake et al., 2020). Many of these applications leverage AR's ability to superimpose digital images on the physical world and use this superimposition to help users achieve specific tasks. AR applications have the potential to enhance visualization of complex medical imaging and add higher degrees of interactivity than conventional 3D reformations on two-dimensional flat screens (Vávra et al., 2017). Improved visualization of complex imaging can potentially be beneficial in reducing operating room times and complication rates and increasing physician confidence prior to performing an operation, specifically by improving user understanding and awareness of complex anatomy that can sometimes be lost in translation with current visualization standards (Oishi et al., 2013; Hassani et al., 2014; Schepers et al., 2015). Cost savings in terms of more-efficient use of hardware has also been demonstrated using digital templating software, where preoperative planning can help in anticipating appropriate hardware needs specific to a patient, and prior to the intervention, preventing the need to open and potentially waste numerous mal-sized hardware during a procedure (Hsu et al., 2012).
These improvements in visualization can become even more important in the pediatric patient, where reductions in OR time, length of anesthesia, and long-term sequelae of repair can have significant consequences (Mehlman et al., 2001; Ramachandran et al., 2008; Howard et al., 2012). It is critical to utilize available tools to try and limit time under anesthesia, particularly in pediatric patients, who may still be undergoing cognitive development. While time for precise characterization and surgical planning is available in non-emergent surgical cases, this time is finite and can be shortened if users are provided with the appropriate tools and imaging to best plan for a procedure (Leet et al., 2002). In many instances, given the precise nature of complex repairs, having an accurate 3D representation of the underlying anatomy is critical.
Previous work with quality control phantoms have demonstrated a high degree of accuracy and precision of AR models in comparison with gold standard measurements (Oral, Scientific, and Educational Abstracts 2018, 2019). Early preliminary work has demonstrated preplanning with 3D models has the potential to decrease operating room times (Jacobs et al., 2008; Zhang et al., 2011; Lethaus et al., 2012) and improve accuracy in hardware placement (Teber et al., 2009; Elmi-Terander et al., 2016; Zhang et al., 2017).
In this study, we retrospectively assess the potential impact of a novel AR software application, Radiology with Holographic Augmentation (RadHA), on pediatric orthopedic surgeons' confidence in surgical planning, hardware selection, hardware fit, and estimated potential intraoperative time savings in the setting of complex adolescent elbow fractures.
Materials/Methods
Approval for the study was obtained through our institutional review board. A total of 12 cases of intra-articular elbow fractures of varying levels of complexity in pediatric patients 10–18 years of age were identified through retrospective review using specified search criteria (Figure 1). Search criteria included patients <18 years of age, undergoing CT, with reports including “comminuted,” “intra-articular,” and “elbow.” Digital Imaging and Communications in Medicine (DICOM) images (Figure 2) were converted to 3D.stl mesh files using OSIRIX MD (Pixmeo SARL, Bernex Switzerland) and optimized for AR using Blender (Stichting Blender Foundation, Amsterdam, Netherlands) (Figure 3). The 3D images were adapted (Figures 4A,B) and loaded onto the Microsoft HoloLens (Microsoft Corporation, Redmond, WA, USA) platform using a novel, investigational software application written in C# programming language-based code on the Unity Platform (Unity Technologies, San Francisco, CA, USA), which was developed at our institution. Abstracted pertinent clinical information (Table 1) and conventional CT DICOM data of the 12 models were reviewed by five separate fellowship-trained attending pediatric orthopedic surgeons (averaging 4.6 years post-fellowship completion, ranging between 2 and 8 years). Subsequently, the same surgeons evaluated an AR model derived from the same CT DICOM data. Surgeons then completed a survey with multiple Likert scale questions regarding use of AR models for planning (Table 2), and included their own subjective estimation of time savings based on use of these AR models (Figure 5 and Table 3). A one sample, two-tailed t test was performed to compare the mean Likert responses to a null hypothesis of no significant change upon review of AR models (Likert = 3) among all and comminuted fracture types (Table 4), with all responses treated as independent measures. A Kruskal–Wallis test was performed to compare differences between Likert responses among surgeons (Table 5), given that our collected ordinal data did not have a normal distribution, both among all and comminuted fracture patterns.
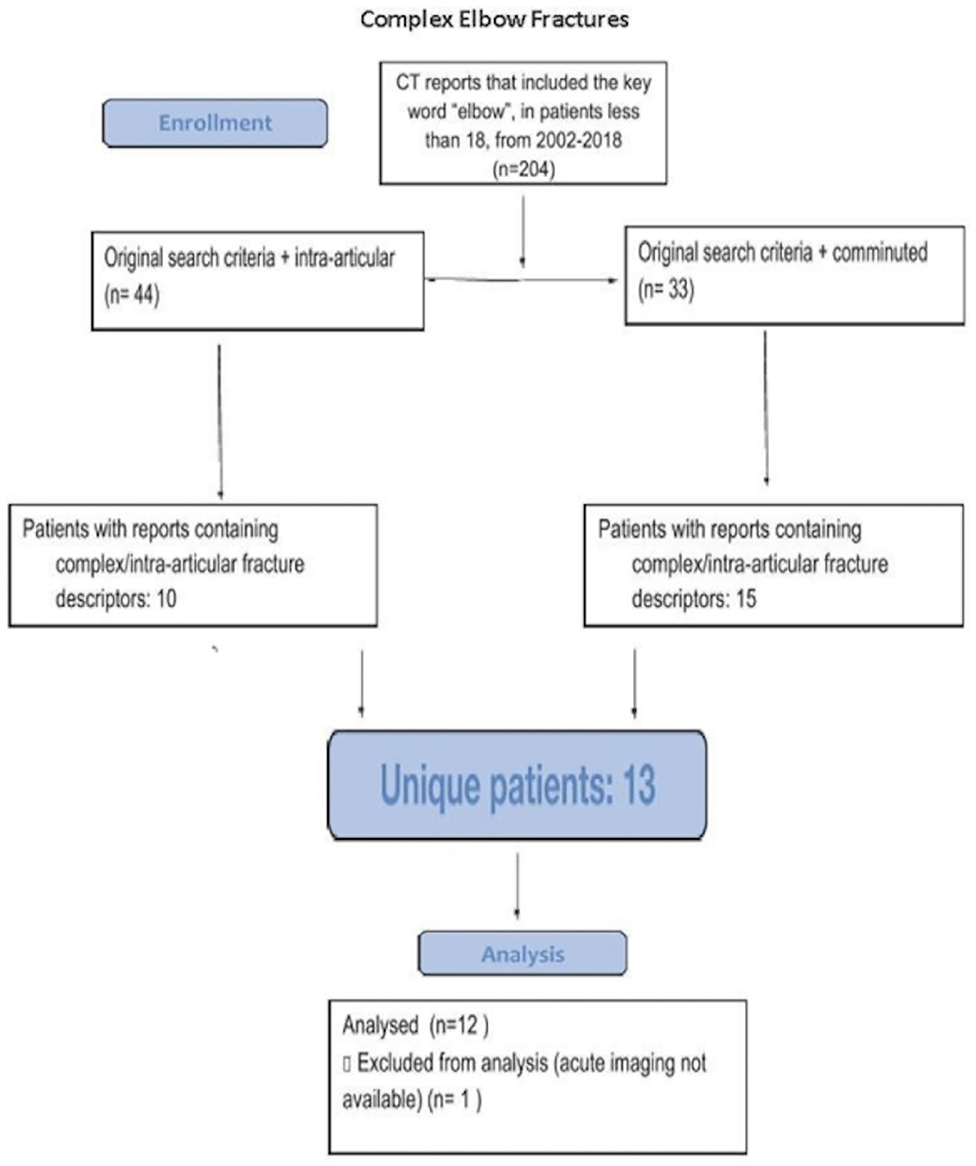
Figure 1. Consort diagram detailing specific search criteria for obtaining our representative cases.
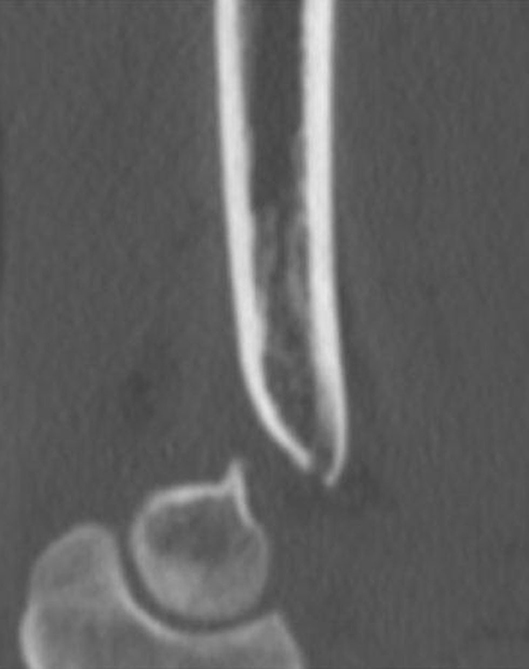
Figure 2. Representative sagittal image of a complex intra-articular elbow fracture in a 16-year-old male teenager as seen on a standard Digital Imaging and Communications in Medicine (DICOM) viewer.
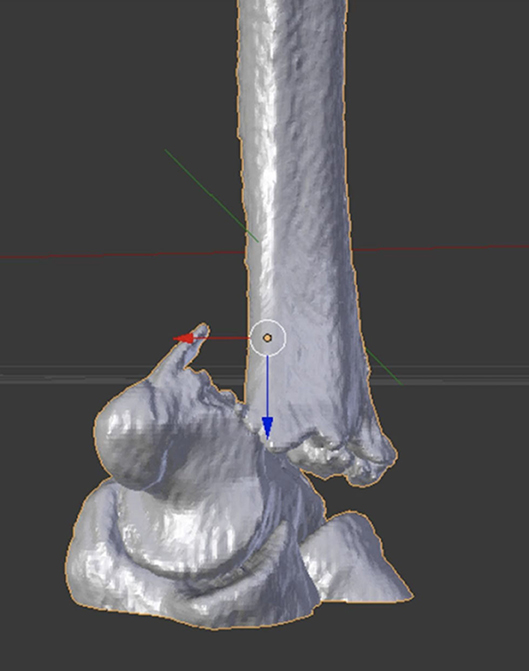
Figure 3. 3D image representation of the elbow fracture, optimized for visualization using Augmented Reality (AR).
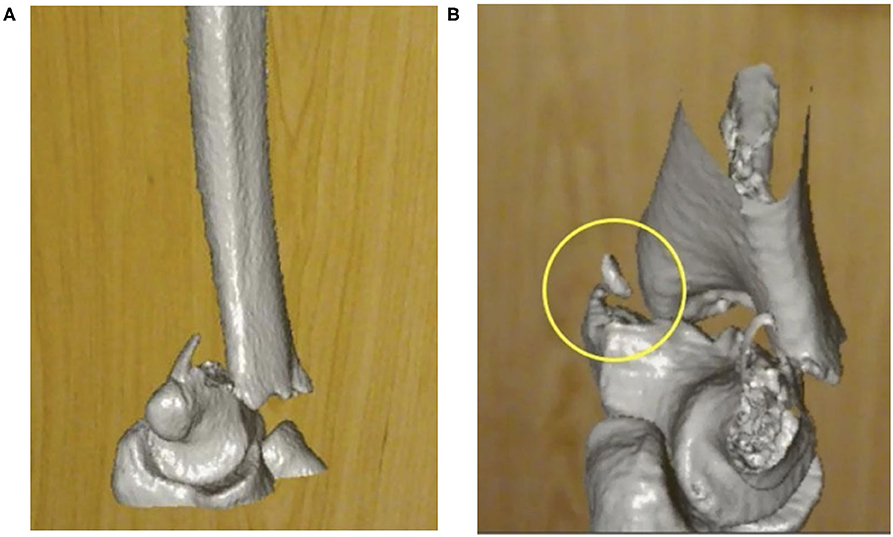
Figure 4. Screen capture placeholders for video clip of the same complex elbow fracture, (A) as seen through an Augmented Reality (AR) head mounted display and (B) highlighting a small fracture fragment (yellow circle), which was reported to be better appreciated on the AR model by reviewers.
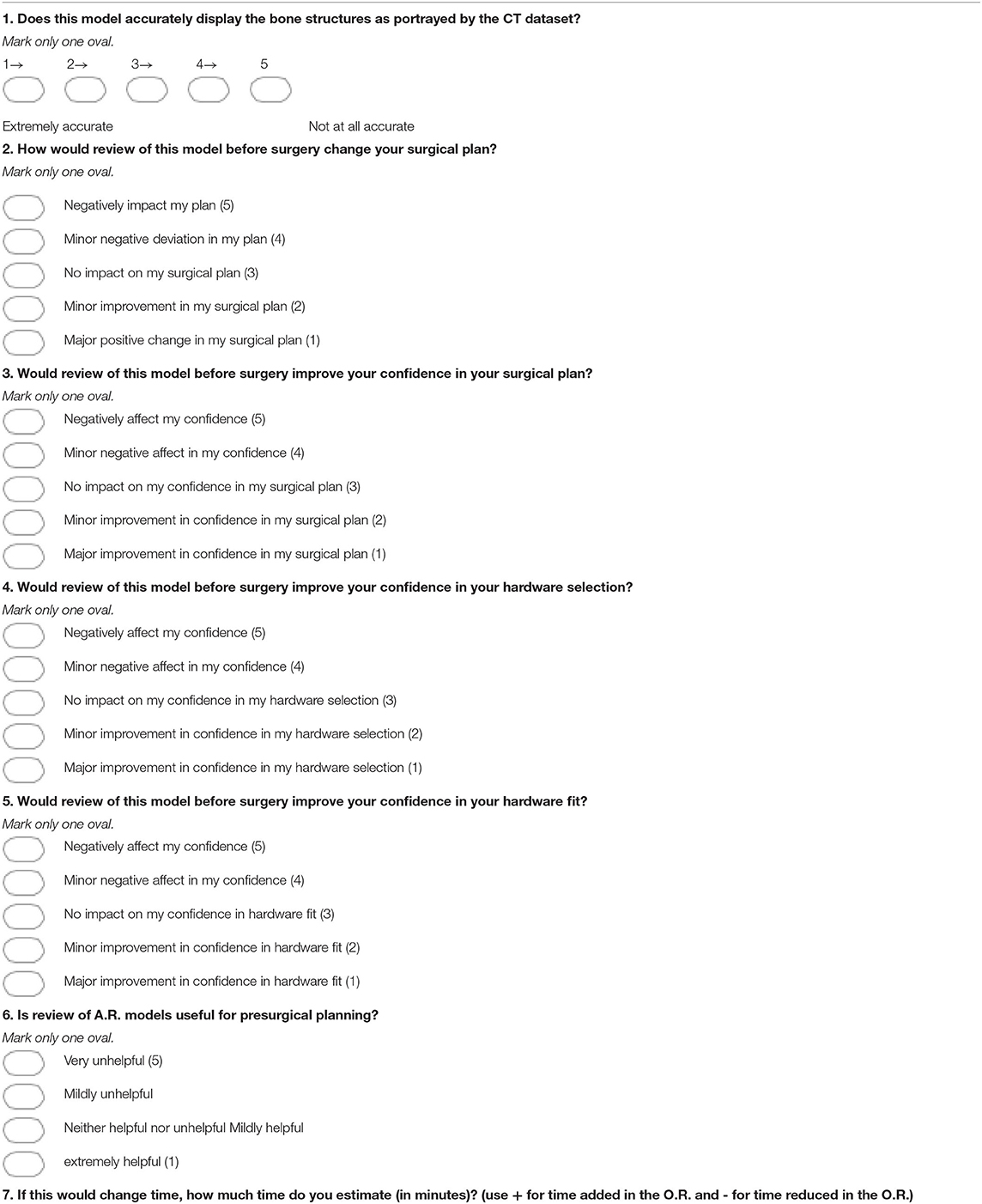
Table 2. Survey questions posed to orthopedic surgeon raters following Augmented Reality (AR) model review.
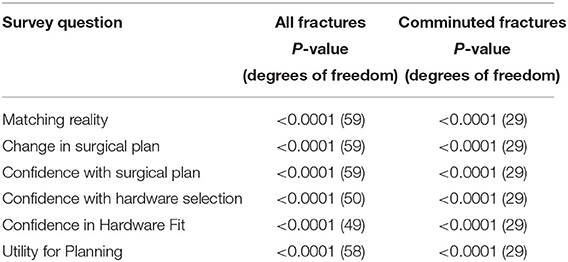
Table 4. One sample two-tailed t test evaluating improvement of Augmented Reality (AR) model in Likert survey questions for all fractures, comparing average responses to a neutral change.
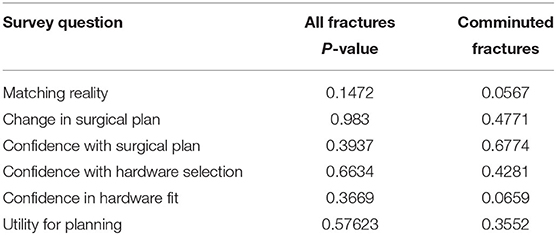
Table 5. Kruskal–Wallis evaluating for significant difference between reviewers for all and comminuted fracture types.
Results
Of the 25 total reports found using the specified search criteria, 13 unique patients were present. A single study was excluded on the basis of absence of acute imaging. A total of 12 patients were therefore included in the study [average age, 13.8 (range, 11–17), 8:4 M/F, mean weight = 54 kg]. Further clinical details on each case is provided in Table 1.
Summary of responses to survey questions (Figures 6A,B) and summary of responses separated by reviewer (Figures 7A,B) are provided. Surgeons rated models “highly accurate” (median = 1, mode = 1, interquartile range = 0). Raters noted no overall change in surgical plan (median = 3, mode = 3, interquartile range = 1). Raters noted improved confidence in surgical plan (median = 2, mode = 1, interquartile range = 1). Confidence scores for hardware selection were on average mildly increased (median = 2, mode = 2, interquartile range = 2), as were confidence scores in hardware fit (median = 2, mode = 2, interquartile range = 1), and utility for planning for specific fracture type (median = 2, mode = 2, interquartile range = 1). Four of the five orthopedic surgeons felt comfortable providing time estimations (the surgeon who most recently completed training abstained, Reviewer 3). Overall average estimated intraoperative time savings for all fracture types = 17.3 min (Table 3). When asked to clarify/expand on how AR models would impact case or affect time estimates, surgeons responded with “better understanding of alignment/fracture read,” “better understanding of surgical approach,” “can see extent better,” “retractor positioning,” and “better understanding of relationships of fragments” (Table 6). A Kruskal–Wallis test was performed to evaluate if there was any significant difference among between different raters and their survey responses. Overall, we did not find a statistical difference between respondents, both in the case of all fractures and in the case of comminuted fracture types (Table 5). This is also graphically depicted (Figures 7A,B), where mean Likert responses and 95% confidence intervals are displayed between reviewers, visually confirming the Kruskal–Wallis test results. Additionally, a t-test (Table 4) allowed us to reject our null hypothesis that the review of AR models would result in no difference among surgeons preparing for pediatric elbow fracture repair.
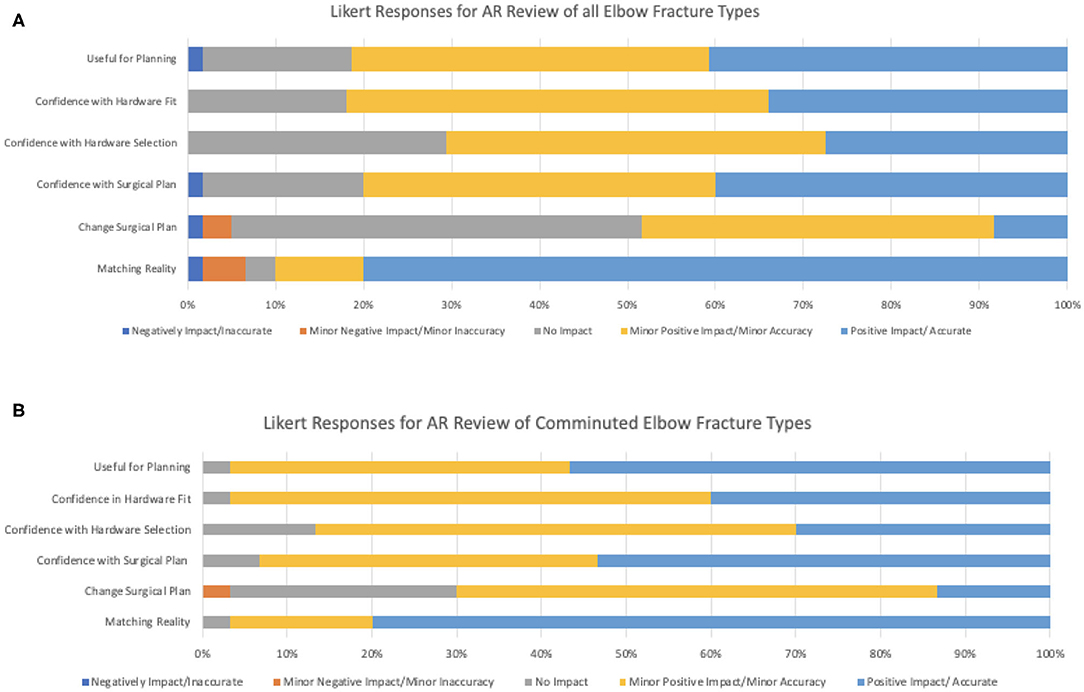
Figure 6. Summary statistics for Likert survey responses (A) for all elbow fracture types and for (B) all comminuted elbow fracture types.
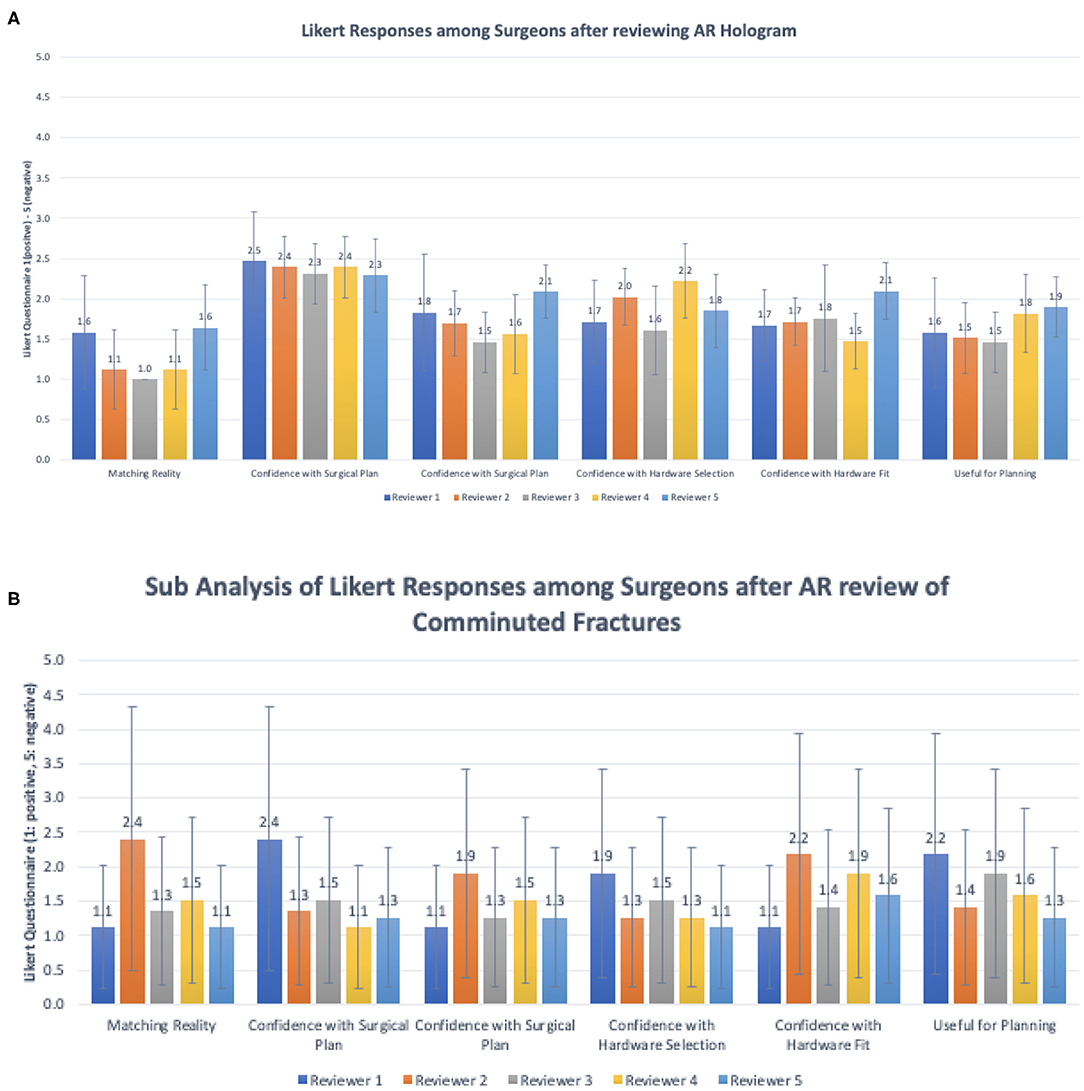
Figure 7. Summary statistics for Likert survey responses organized by individual reviewers (A) within all fracture types and (B) within comminuted fracture types.
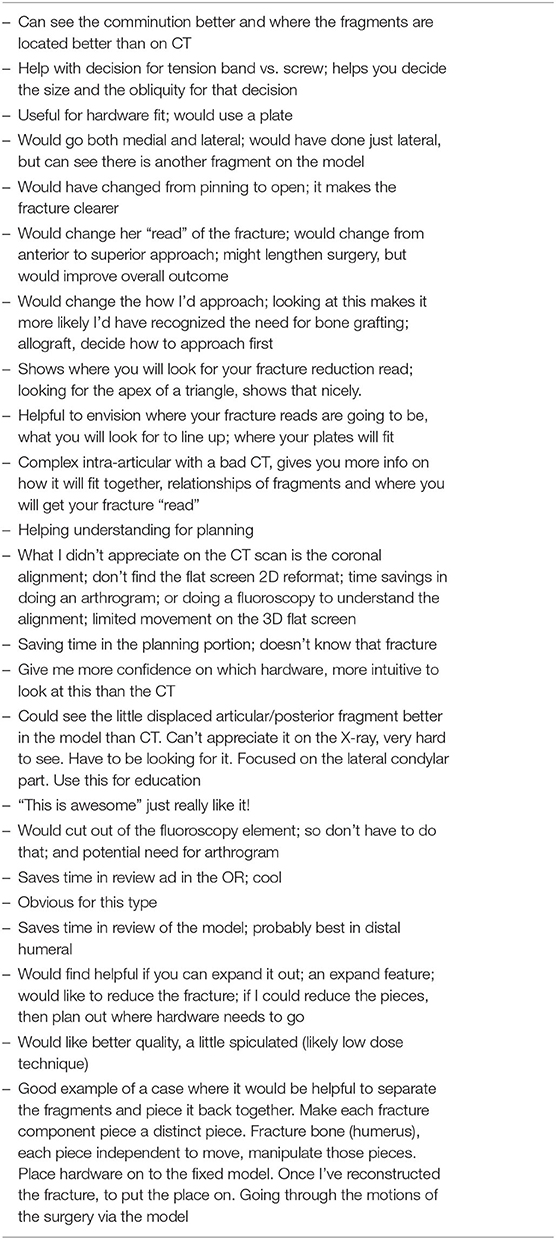
Table 6. Responses to “any other comments or clarification on how these AR models change your management or potentially help reduce operative time?”
When subanalyzed in the cases of complex fractures (fractures more than simple, non-displaced fractures, n = 6), surgeons rated: subjective assessment of model accuracy “highly accurate” = (scale 1–5, median = 1, mode = 1, interquartile range = 0); confidence in surgical plan “major increase” = (median = 1, mode = 1, interquartile range = 1); confidence in hardware selection “mild increase” = (median = 2, mode = 2, interquartile range = 1); confidence in hardware fit “mild increase” = (median = 2, mode = 2, interquartile range = 1). Utility for planning for specific fracture type was rated “high” = (median = 1, mode = 1, interquartile range = 1). In the cases of comminuted fracture types, average estimated intraoperative time savings was 17.6 min.
Discussion
In this study, we evaluated orthopedic surgeons' assessment of 3D AR models for presurgical planning in complex elbow fractures. Surgeons reported high confidence in the quality of the AR models created. Additionally, surgeons reported increased confidence in their surgical plan, increased confidence in hardware selection, and increased confidence in hardware fit. Within the subanalysis of comminuted fractures, there was a greater overall increase in surgeon confidence in surgical plan. Overall, there was a projected intraoperative time savings (averaging 17.3 min for all fracture types and 17.6 min for comminuted fractures). The most experienced of the surgeons interestingly estimated that the use of this technology could have up to a 30-min time reduction in the cases of complex fractures.
The improved 3D visual spatial awareness of the fracture fragments and fracture patterns, as cited by the surveyed surgeons, is similar with previously reported studies of 3D imaging use in orthopedics (Sasso and Garrido, 2007; Citak et al., 2008; Yu et al., 2015). This includes a study of 3D modeling for pelvic acetabular fractures, which allowed for improved hardware placement and reduced soft tissue trauma (Yu et al., 2015). Notably, reviewers in this study found that AR models provided additional value over standard 3D reformations through improved ability to interact with and freely manipulate the AR model (as opposed to the fixed horizontal and vertical spin directions of rotation provided on routine clinical imaging). Furthermore, the ability to interact with real world objects with the AR models was reported as an advantage, providing the potential opportunity for hardware sizing.
Time reduction of ~18 min per case in cases of complex fractures in our study, while an estimation, is in keeping with other studies of time reduction using 3D visualization (Sasso and Garrido, 2007; Citak et al., 2008). Physicians reported a variety of mechanisms for time reduction (Table 6). These included enhanced visualization of relevant anatomy, reducing total preoperative review time; prevention of unnecessary procedures during a case, such as an arthrogram or fluoroscopy to understand anatomy; precise hardware selection, reducing time spent deciding between hardware types; aid in appropriate surgical technique, either in approach or specific technique, potentially reducing intraprocedural time; and aids operator “read” of fracture pattern, possibly reducing time spent reviewing CT prior to surgery. Reported operating times for repair of complex distal humeral fractures in a study in the adult population averaged 2.5 h (Frankle et al., 2003). Furthermore, reported costs per minute of operating room time range from $36.50/min to $133.12, averaging $66/min (Shippert, 2005; Macario, 2010; Childers and Maggard-Gibbons, 2018). While an estimation, using the provided estimates of our study, this could represent a potential 12% overall decrease in time per case and a reduction in cost of $1,122 per case.
The time to segment, process, and optimize orthopedic models for this AR application is <5 min, as the processing is done electronically. This increases the potential for such applications to be used more broadly than alternative options such as 3D printing that require more resources and infrastructure. Further, the opportunity for interaction between physical orthopedic hardware and scale 3D holographic models provides a degree of interactivity that is beyond conventional 3D-rendered reformats displayed on a 2D monitor.
Our study was not without limitations. In addition to the retrospective nature of the study, our study was limited by the subjective assessment of projected time savings and by the differences in training and degree of experience by the surgeons reviewing models. Further, while the models available for review had limitations in degree of interactivity in that the fracture fragments on the tested version could not be manipulated or repositioned, this was a feature that reviewing surgeons stated would have been useful in planning. Within these limitations, we do believe that our study not only provides critical information on the improvement in surgeon confidence in plan and hardware choice but also provides an early preliminary window into the potential economic impact that this technology can provide, which may influence further adoption.
Preoperative planning using AR-based models statistically increases surgeon confidence in preoperative planning, hardware selection, and confidence in hardware fit. This study lays a foundation for further, larger-scale prospective trials assessing AR technology for planning in a wider variety of orthopedic fracture types. Additionally, further testing should be focused on the safety and barriers of using intraoperative AR systems for surgical guidance and reference.
Data Availability Statement
The datasets generated for this study are available on request to the corresponding author.
Ethics Statement
This work was conducted with approval of our University's Institutional Review Board.
Author Contributions
BL: manuscript preparation, statistical analysis, and figure legends. KL: study design, survey design input, performed measurements, and manuscript preparation. RB, JJ, NP, and CS: manuscript preparation and performed measurements. JC: study design, manuscript preparation, figure annotation, video editing, statistical analysis, and project oversight. All authors contributed to the article and approved the submitted version.
Funding
This work was funded by the Clinical and Translational Science Institute (CTSI) and UCSF Catalyst Program of Innovation Ventures Fund #5018, Project #2001449.
Conflict of Interest
BL and JC are founders of Sira Medical, pre-revenue Augmented Reality startup. BL is a co-founder of an AR company Sira Medical.
The remaining authors declare that the research was conducted in the absence of any commercial or financial relationships that could be construed as a potential conflict of interest.
Acknowledgments
Preliminary findings of this work were presented at the 2018 Annual Meeting of the American Roentgen Ray Society, Washington, DC. Microsoft HoloLens v1 was used with permission from Microsoft (this equipment was purchased and owned by the BL). We thank Dr. Ivor Asztalos for statistical guidance.
Supplementary Material
The Supplementary Material for this article can be found online at: https://www.frontiersin.org/articles/10.3389/frvir.2020.528810/full#supplementary-material
References
Abzug, J. M., and Herman, M. J. (2012). Management of supracondylar humerus fractures in children: current concepts. J. Am. Acad. Orthop. Surg. 20, 69–77. doi: 10.5435/00124635-201202000-00002
Atesok, K., and Schemitsch, E. H. (2010). Computer-assisted trauma surgery. J. Am. Acad. Orthop. Surg. 18, 247–258. doi: 10.5435/00124635-201005000-00001
Childers, C. P., and Maggard-Gibbons, M. (2018). Understanding costs of care in the operating room. JAMA Surg. 153:e176233. doi: 10.1001/jamasurg.2017.6233
Citak, M., Gardner, M. J., Kendoff, D., Tarte, S., Krettek, C., Nolte, L.-P., et al. (2008). Virtual 3D planning of acetabular fracture reduction. J. Orthop. Res. 26, 547–552. doi: 10.1002/jor.20517
Elmi-Terander, A., Skulason, H., Söderman, M., Racadio, J., Homan, R., Babic, D., et al. (2016). Surgical navigation technology based on augmented reality and integrated 3d intraoperative imaging: a spine cadaveric feasibility and accuracy study. Spine 41, E1303–E1311. doi: 10.1097/BRS.0000000000001830
Fotouhi, J., Alexander, C. P., Unberath, M., Taylor, G., Lee, S. C., Fuerst, B., et al. (2018). Plan in 2-D, execute in 3-D: an augmented reality solution for cup placement in total hip arthroplasty. J. Med. Imaging 5:021205. doi: 10.1117/1.JMI.5.2.021205
Frankle, M. A., Herscovici, D., DiPasquale, T. G., Vasey, M. B., and Sanders, R. W. (2003). A comparison of open reduction and internal fixation and primary total elbow arthroplasty in the treatment of intraarticular distal humerus fractures in women older than age 65. J. Orthop. Trauma 17, 473–480. doi: 10.1097/00005131-200308000-00001
Handelsman, J. E., Weinberg, J., and Hersch, J. C. (2006). Corrective supracondylar humeral osteotomies using the small AO external fixator. J. Pediatr. Orthop. B 15, 194–197. doi: 10.1097/01.bpb.0000194440.75378.97
Hassani, H., Cherix, S., Ek, E. T., and Rüdiger, H. A. (2014). Comparisons of preoperative three-dimensional planning and surgical reconstruction in primary cementless total hip arthroplasty. J. Arthroplasty 29, 1273–1277. doi: 10.1016/j.arth.2013.12.033
Houshian, S., Mehdi, B., and Larsen, M. S. (2001). The epidemiology of elbow fracture in children: analysis of 355fractures, with special reference to supracondylar humerus fractures. J. Orthop. Sci. 6, 312–315. doi: 10.1007/s007760100024
Howard, A., Mulpuri, K., Abel, M. F., Braun, S., Bueche, M., Epps, H., et al. (2012). The treatment of pediatric supracondylar humerus fractures. J. Am. Acad. Orthop. Surg. 20, 320–327. doi: 10.5435/JAAOS-20-05-320
Hsu, A. R., Gross, C. E., Bhatia, S., and Levine, B. R. (2012). Template-directed instrumentation in total knee arthroplasty: cost savings analysis. Orthopedics 35, e1596–e1600. doi: 10.3928/01477447-20121023-15
Jacobs, S., Grunert, R., Mohr, F. W., and Falk, V. (2008). 3D-Imaging of cardiac structures using 3D heart models for planning in heart surgery: a preliminary study. Interact. Cardiovasc. Thorac. Surg. 7, 6–9. doi: 10.1510/icvts.2007.156588
Koh, K. H., Seo, S. W., Kim, K. M., and Shim, J. S. (2010). Clinical and radiographic results of lateral condylar fracture of distal humerus in children. J. Pediatr. Orthop. 30, 425–429. doi: 10.1097/BPO.0b013e3181df1578
Leet, A. I., Frisancho, J., and Ebramzadeh, E. (2002). Delayed treatment of type 3 supracondylar humerus fractures in children. J. Pediatric Orthop. 22, 203–207. doi: 10.1097/01241398-200203000-00014
Lethaus, B., Poort, L., Böckmann, R., Smeets, R., Tolba, R., and Kessler, P. (2012). Additive manufacturing for microvascular reconstruction of the mandible in 20 patients. J. Craniomaxillofac. Surg. 40, 43–46. doi: 10.1016/j.jcms.2011.01.007
Macario, A. (2010). What does one minute of operating room time cost? J. Clin. Anesth. 22, 233–236. doi: 10.1016/j.jclinane.2010.02.003
Mehlman, C. T., Strub, W. M., Roy, D. R., Wall, E. J., and Crawford, A. H. (2001). The effect of surgical timing on the perioperative complications of treatment of supracondylar humeral fractures in children. J. Bone Joint Surg. Am. 83, 323–327. doi: 10.2106/00004623-200103000-00002
Oishi, M., Fukuda, M., Yajima, N., Yoshida, K., Takahashi, M., Hiraishi, T., et al. (2013). Interactive presurgical simulation applying advanced 3D imaging and modeling techniques for skull base and deep tumors. J. Neurosurg. 119, 94–105. doi: 10.3171/2013.3.JNS121109
Oral, Scientific, and Educational Abstracts 2018 (2019). Available online at: https://cf.arrs.org/abstracts/oralpresentations/index.cfm?fid=1210&app=false (accessed July 8, 2018).
Park, D. Y., Cho, J. H., Lee, D.-H., Choi, W.-S., Bang, J. Y., and Yin, X. Y. (2017). A 3-Dimensional analysis of the fracture planes in pediatric lateral humeral condyle fractures for image-based pin positioning during fixation. J. Orthop. Trauma 31, e340–e346. doi: 10.1097/BOT.0000000000000914
Ramachandran, M., Skaggs, D. L., Crawford, H. A., Eastwood, D. M., Lalonde, F. D., Vitale, M. G., et al. (2008). Delaying treatment of supracondylar fractures in children: has the pendulum swung too far? J. Bone Joint Surg. Br. 90, 1228–1233. doi: 10.1302/0301-620X.90B9.20728
Sasso, R. C., and Garrido, B. J. (2007). Computer-assisted spinal navigation versus serial radiography and operative time for posterior spinal fusion at L5-S1. J. Spinal Disord. Tech. 20, 118–122. doi: 10.1097/01.bsd.0000211263.13250.b1
Schepers, R. H., Kraeima, J., Vissink, A., Lahoda, L. U., Roodenburg, J. L. N., Reintsema, H., et al. (2016). Accuracy of secondary maxillofacial reconstruction with prefabricated fibula grafts using 3D planning and guided reconstruction. J. Craniomaxillofac. Surg. 44, 392–399. doi: 10.1016/j.jcms.2015.12.008
Schepers, R. H., Raghoebar, G. M., Vissink, A., Stenekes, M. W., Kraeima, J., Roodenburg, J. L., et al. (2015). Accuracy of fibula reconstruction using patient-specific CAD/CAM reconstruction plates and dental implants: a new modality for functional reconstruction of mandibular defects. J. Craniomaxillofac. Surg. 43, 649–657. doi: 10.1016/j.jcms.2015.03.015
Shippert, R. D. (2005). A study of time-dependent operating room fees and how to save $100 000 by using time-saving products. Am. J. Cosmetic Surg. 22, 25–34. doi: 10.1177/074880680502200104
Teber, D., Guven, S., Simpfendörfer, T., Baumhauer, M., Güven, E. O., Yencilek, F., et al. (2009). Augmented reality: a new tool to improve surgical accuracy during laparoscopic partial nephrectomy? Preliminary in vitro and in vivo results. Eur. Urol. 56, 332–338. doi: 10.1016/j.eururo.2009.05.017
Vávra, P., Roman, J., Zonča, P., Ihnát, P., Němec, M., Kumar, J., et al. (2017). Recent development of augmented reality in surgery: a review. J. Healthc. Eng. 2017:4574172. doi: 10.1155/2017/4574172
Wake, N., Nussbaum, J. E., Elias, M. I., Nikas, C. V., and Bjurlin, M. A. (2020). 3D Printing, augmented reality, and virtual reality for the assessment and management of kidney and prostate cancer: a systematic review. Urology 143, 20–32. doi: 10.1016/j.urology.2020.08.022
Wicky, S., Blaser, P. F., Blanc, C. H., Leyvraz, P. F., Schnyder, P., and Meuli, R. A. (2000). Comparison between standard radiography and spiral CT with 3D reconstruction in the evaluation, classification and management of tibial plateau fractures. Eur. Radiol. 10, 1227–1232. doi: 10.1007/s003300000326
Yu, A. W., Duncan, J. M., Daurka, J. S., Lewis, A., and Cobb, J. (2015). A feasibility study into the use of three-dimensional printer modelling in acetabular fracture surgery. Adv. Orthop. 2015:617046. doi: 10.1155/2015/617046
Zhang, X., Chen, G., and Liao, H. (2017). High-quality see-through surgical guidance system using enhanced 3-D autostereoscopic augmented reality. IEEE Trans. Biomed. Eng. 64, 1815–1825. doi: 10.1109/TBME.2016.2624632
Zhang, Y. Z., Chen, B., Lu, S., Yang, Y., Zhao, J. M., Liu, R., et al. (2011). Preliminary application of computer-assisted patient-specific acetabular navigational template for total hip arthroplasty in adult single development dysplasia of the hip. Int. J. Med. Robot. 7, 469–474. doi: 10.1002/rcs.423
Keywords: Hololens, radiology, DICOM, Augmented Reality (AR), computed tomography
Citation: Laguna B, Livingston K, Brar R, Jagodzinski J, Pandya N, Sabatini C and Courtier J (2020) Assessing the Value of a Novel Augmented Reality Application for Presurgical Planning in Adolescent Elbow Fractures. Front. Virtual Real. 1:528810. doi: 10.3389/frvir.2020.528810
Received: 21 January 2020; Accepted: 15 September 2020;
Published: 26 November 2020.
Edited by:
Holger Thomas Regenbrecht, University of Otago, New ZealandReviewed by:
Christopher M. Laine, University of Southern California, United StatesAli Fardinpour, Wise Realities Institute for Healthcare Emerging Technologies Research, Australia
Copyright © 2020 Laguna, Livingston, Brar, Jagodzinski, Pandya, Sabatini and Courtier. This is an open-access article distributed under the terms of the Creative Commons Attribution License (CC BY). The use, distribution or reproduction in other forums is permitted, provided the original author(s) and the copyright owner(s) are credited and that the original publication in this journal is cited, in accordance with accepted academic practice. No use, distribution or reproduction is permitted which does not comply with these terms.
*Correspondence: Jesse Courtier, amVzc2UuY291cnRpZXImI3gwMDA0MDt1Y3NmLmVkdQ==