- 1Department of Biochemistry, Schulich School of Medicine & Dentistry, University of Western Ontario, London, ON, Canada
- 2Schulich School of Medicine & Dentistry, University of Western Ontario, London, ON, Canada
- 3Department of Medicine, Division of Nephrology, London Health Sciences Centre, London, ON, Canada
Nephrolithiasis is a condition in which crystals precipitate out of the urine forming kidney stones in the renal calyces and pelvis. Approximately 80% of stones are composed of calcium oxalate and calcium phosphate. In recent years, there has been a significant increase in the prevalence of nephrolithiasis across populations, specifically in that of the pediatric population. The etiology of stone disease is multifactorial, and includes environmental, dietary, hormonal, and genetic factors. Evidence for monogenic causation (also known as Mendelian or single-gene disorders) in nephrolithiasis includes the finding that 30% of children with stone disease report a positive family history, with monogenic nephrolithiasis accounting for approximately 30% of cases. Monogenic nephrolithiasis can occur in isolation or may be the result of an underlying genetic disorder including autosomal dominant hypocalcemia (ADH), primary hyperoxalurias, and hereditary hypophosphatemic rickets with hypercalciuria (HHRH), to name a few. Currently, there are 41 known genes that represent monogenic causes of human nephrolithiasis. Since early detection of these mutations can in some cases prevent the progression to end stage kidney disease in pediatric patients, establishing the genetic basis for nephrolithiasis is increasingly important. Here we provide an overview of kidney stone disease in children with a focus on monogenic causation in the pediatric population.
Introduction
Nephrolithiasis is a common condition in which crystals of mineral concentrations precipitate out of the urine, forming kidney stones in the renal calyces and pelvis (1). There are four main distributions of compositions of kidney stones; 80% of stones are composed of calcium oxalate and calcium phosphate, 8-10% are uric acid stones, 7-8% are struvite stones, and about 2-5% are cysteine stones (2). The most common stone formation, calcium oxalate, begins with nucleation, crystal growth and then crystal aggregation to ultimately form a stone (3). There are many factors which influence the development of nephrolithiasis. Some are promoters of calcium supersaturation including high urinary excretion of calcium, oxalate, urate, and low urine volume; others are inhibitors of stone formation including citrate, magnesium and potassium (4). Patients diagnosed with nephrolithiasis typically present with abdominal and flank pain, fever and chills, nausea and vomiting, hematuria, dysuria, and urinary frequency (5). Common diagnostic techniques include computed tomography (CT), ultrasonography, and urinalysis (5). Treatment options for both adults and children include pain management, urinary decompression if secondary obstruction is present, medical expulsion therapy, ureteroscopy, and extracorporeal shockwave lithotripsy (6). Patients with nephrolithiasis also have an increased risk of both chronic kidney disease (CKD) and end stage kidney disease (ESKD) (7). Somewhat surprising is that even asymptomatic kidney stone patients are at a higher risk of developing ESKD (7). This elevated risk of CKD and ESKD has prompted the increased use of genetic evaluation to facilitate in the diagnosis, specific treatment strategies, and ultimately preventing recurrence of stone disease (7). The added benefit of establishing a genetic diagnosis in pediatric patients is that it can lead to early disease detection, provide a definitive molecular diagnosis, inform prognosis in both patients and potentially affected family members (8).
Prevalence of kidney stone disease
Kidney stone disease is common worldwide, with an ever-increasing prevalence. In the last decade, 1 in 10 people in the United States report a history of nephrolithiasis, compared to 1 in 20 individuals in 1994 (9). One of the most prominent increases in nephrolithiasis incidence over the past few decades has occurred in the pediatric population (10). Studies in the United States have reported a yearly increase in incidence in nephrolithiasis from 1984 to 1990 of 7.2 per 100,000 children under the age of 18, and 14.5 per 100,000 from 2003 to 2008 with an all-time high of 65.2 cases per 100,00 children in 2011 (10, 11). It is predicted that the prevalence of this disease will continue to increase in all populations, including the pediatric population.
Pathophysiology of kidney stones
Kidney stones are solid masses with sizes that vary from a grain of sand to a pearl and can be either yellow or brown in color (12). Kidney stones arise from a positive imbalance in urinary promoters and inhibitors of crystallization, causing supersaturation leading to crystallization (13). The stages of formation are as follows: crystal nucleation begins with solute molecules becoming clustered in solvent (12). Supersaturation then occurs, followed by crystal growth by encrustation once a nucleus is present, and finally agglomeration of the stone (Figure 1) (12). There are four different forms of kidney stones: 1) calcium oxalate and phosphate (80%), 2) uric acid (8-10%), 3) struvite stones (7-8%), and 4) cysteine stones (2-5%) (2). Calcium nephrolithiasis is the most common stone type, with calcium oxalate significantly more prevalent than calcium phosphate stone formation (15). Calcium oxalate stones grow and form at Randall’s plaques which are lesions that seem to provide the platform for calcium oxalate crystal formation (Figure 1) (15). Hypercalciuria, excess calcium in urine, is one of the most important pathophysiological factors for the development of calcium nephrolithiasis (15). When calcium is in abundance, it increases the ionic activity and saturation of the crystalizing salts; oxalate and phosphate, while binding to stone inhibitors (15). This same effect can also be caused by high dietary salt (16). Hypercalciuria is mainly caused by systemic acidosis, since chronic acidosis leads to renal calcium leak, and excess protein load (15). Hypocitraturia often goes hand in hand with hypercalciuria, as citrate complexes with calcium and prevents stone growth, therefore a lack of citrate permits calcium oxalate and phosphate stones (17). It has also been reported that hyperoxaluria causes calcium oxalate stones by increasing the concentration of calcium oxalate in urine (17). Uric acid nephrolithiasis, which is less common, is caused by hyperuricosuria, acidic pH, or a combination of the two (15). Struvite stones, or “infection stones”, are not caused by metabolic deficiency, but rather urease-positive microorganisms which produce ammonium and bicarbonate which causes struvite crystallization (15). The final type, cysteine nephrolithiasis, is mainly caused by genetic defects, although each stone types may have underlain genetic causation (15).
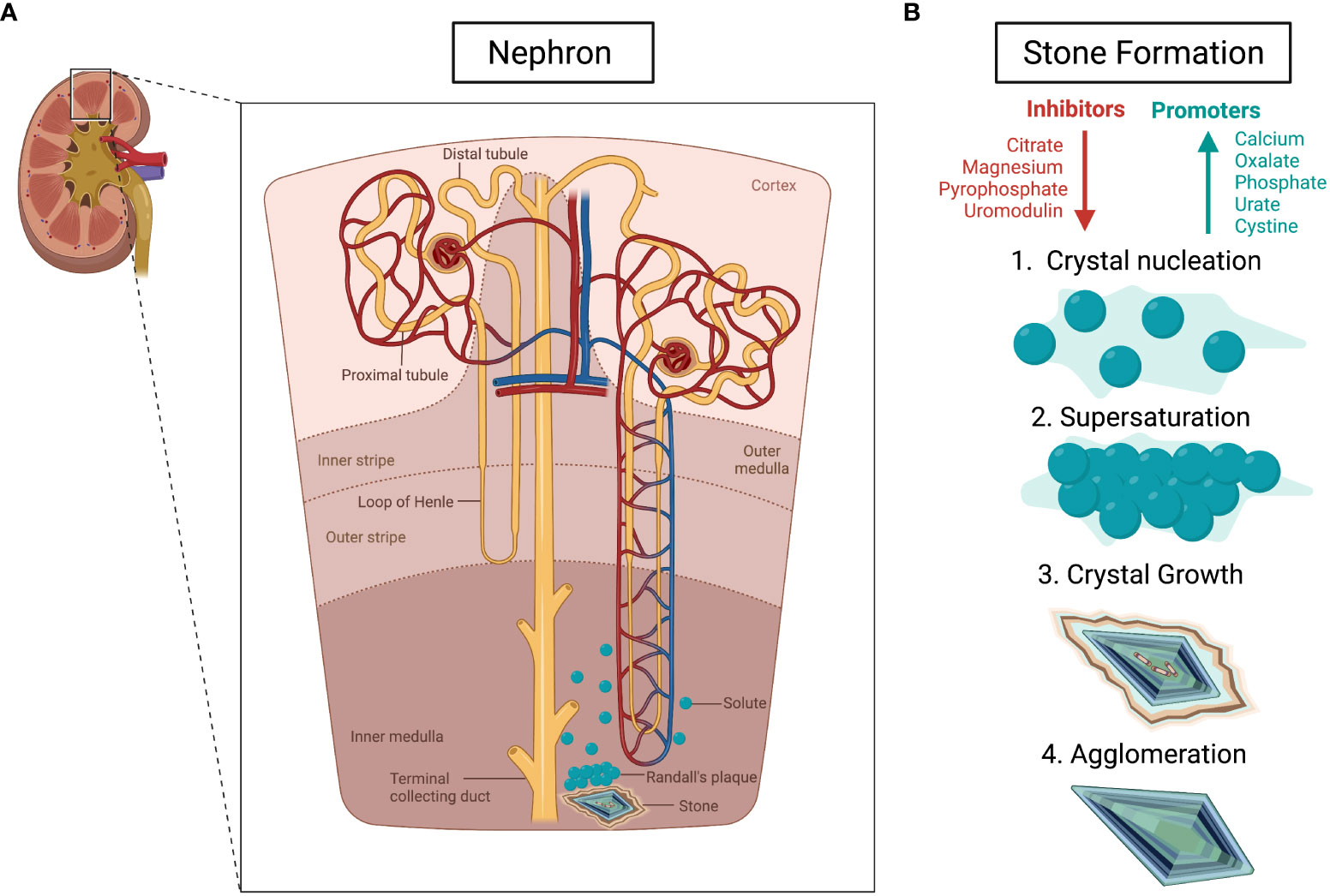
Figure 1 Pathophysiology of kidney stones. (A) The development of kidney stones through Randal’s plaque in the nephron. (B) The steps of kidney stone formation, beginning with an imbalance of inhibitors (shown in red) and promoters (shown in blue). The arrows represent an increase (blue) and decrease (red). Created using BioRender.com (14).
Risk factors for kidney stones
Factors hypothesized to contribute to this rise in nephrolithiasis prevalence include dietary habits, medications, and global warming (18). Many other risk factors are also at an all-time high, and include obesity, diabetes, hypertension, and metabolic syndrome (3). Obesity and diabetes alone increase the risk of developing nephrolithiasis by 55% and 59%, respectively (9). Researchers have discovered that nutritional exposure is one of the most important factors in the increase in prevalence of nephrolithiasis (3). Recent trends have shown that the reduction of intake of fluids, calcium, and fruit intake, as well as an increase in dietary sodium, meat and high oxalate-containing foods are all major contributors in the development of nephrolithiasis (3). Managing these nutritional factors is crucial for nephrolithiasis management and prevention, along with prevention of systemic and cardiovascular comorbidities (3). Various medications including antibiotics, diuretics, laxatives, carbonic anhydrase inhibitors, ephedra alkaloids, potassium channel blockers, reverse transcriptase inhibitors, sulfonylureas, and others, also pose a risk in nephrolithiasis development and recurrence (5). Because nephrolithiasis has a 50% 5-year recurrence establishing a definitive diagnosis and targeting potential modifiable risk factors is vital to avoid recurrence of disease (19).
Evidence for genetic causation in kidney stone disease
Although kidney stone formation can be caused by environmental, dietary, and hormonal factors as outlined above, another significant contributor is genetics. There is strong evidence that nephrolithiasis aggregates in families; indeed, there is a 3-fold increase in incidence when there is a positive family history (20). Data suggest that 35-60% of patients with stone disease have a family history (20). Twin studies have shown that there is a 45% heritability factor for developing nephrolithiasis (21). The family clustering index for nephrolithiasis is 2.5-4 (20, 22). This is comparable to other diseases with a strong evidence of genetic background including diabetes and hypertension, both which have a heritability factor of 2 (23). Dietary habits including a high sodium diet and decreased fluid intake are other risk factors for developing nephrolithiasis (3). Studies have shown that diet, which was thought to be mainly attributed to environmental causation, also has a heritability of 65% for meal size and 44% for meal frequency, thereby linking diet to genetics (24).
Studies show that pathogenic, single gene mutations account for a high proportion of kidney stone disease in childhood (18). For example, Daga demonstrated that among a cohort of patients who presented with kidney stones before the age of 25, exome sequencing detected a monogenic causative mutation in 15 of 51 patients (29.4%) (18). Another study conducted by Huang, detected monogenic causative mutations using exome sequencing in 24 of 32 cases (75%) (25). The majority (80%) of patients with monogenic stone disease carry recessive mutations and most have disease onset before the age of 10 years (18). Currently, there are 41 known genes that represent monogenic causes of human nephrolithiasis (Table 1). Yucheng found that between 17-29% of cases of childhood onset kidney stones are due to mutation in one of these known kidney stone genes (65). However, the heritability factor for nephrolithiasis is over 45% suggesting that a higher that reported proportion of kidney stones are due to single gene disorders in yet to be discovered genes (21).
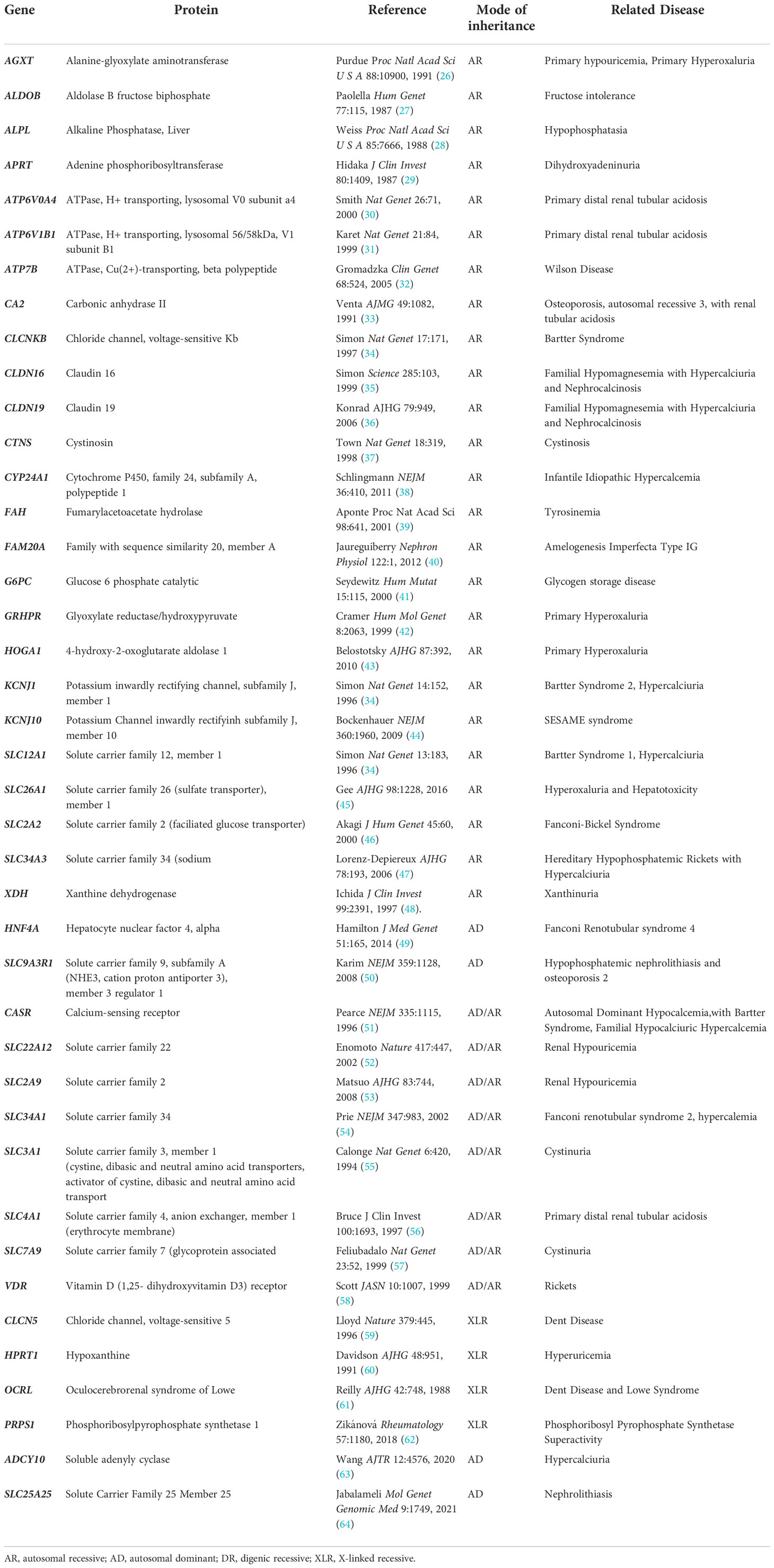
Table 1 Genes causing monogenic human nephrolithiasis. Related monogenic nephrolithiasis disorders also provided.
Clinical diagnosis of kidney stone disease
With the rising incidence of pediatric nephrolithiasis, it is crucial for healthcare providers to use optimal diagnosis strategies (66). Symptoms of nephrolithiasis may vary, or appear mild in pediatric populations, therefore a high index of clinical suspicion is warranted particularly in individuals with a positive family history of stone disease (67). Evaluation requires a complete medical history and physical examination, along with laboratory investigations and diagnostic imaging as outlined below (68). Although symptoms are variable, many patients will present with either renal colic (episodic pain in the renal angle) or hematuria associated with abdominal pain (66). After acute management of symptoms, diagnostic imaging is required to confirm the presence of one or more stones (66). This can include computed tomography (CT) scan, ultrasound or kidney and urinary tract, or X-ray film of the abdomen, all of which may help confirm the presence or absence of stone disease (66). Ultrasound is specifically recommended in pediatric populations, as opposed to the computed tomography scan used in adult populations, although it should be noted that ultrasound may have limitations in its capacity to detect stones (67–69). If stone presence is verified by imaging, a detailed family history and complete metabolic evaluation are warranted (66). A urology consult may also be necessary if the patient has renal colic, infection is present, the stone is larger than 5mm making spontaneous passage of the stone unlikely, or there are sign of obstruction within the urinary tract (66).
In pediatric patients, it is recommended that a complete in-depth metabolic analysis is completed (70). This consist of serum and 24-hour urine tests, as well as spot urine analysis and a full dietary history (70). A 24-hour urine collection collects data on volume, creatinine, calcium, sodium, potassium, oxalate, citrate, uric acid, magnesium, and cystine (70). Analysis of blood samples should also be completed, and include serum sodium, potassium, bicarbonate, creatinine, calcium, phosphorus, magnesium, uric acid, citrate, parathyroid hormone (PTH) and vitamin D evaluation (66). These tests provide evidence of either the presence of promoter substances (i.e., excess calcium in the urine) or absence of inhibitor substance (i.e., low urinary citrate levels). These evaluations can provide evidence for underlying metabolic disorders such as hypercalciuria, hyperoxaluria, hyperuricuria, and hypocitraturia (68), all which can promote stone formation. The gold standard for confirming stone type is analysis of the stone composition following passage or extraction and recovered of a stone (66). If all tests are inconclusive, one may require genetic evaluation to determine disease causation.
Genetic evaluation in kidney stone disease
Currently, the Canadian Urological Association (CUA) Guidelines do not recommend genetic testing as a first tier diagnostic tool for pediatric patients with isolated kidney, however it is recommended as a future direction in patients with recurrent nephrolithiasis (70). Genetic testing was previously too costly and unavailable to many patients, but this has changed with new advancements in high throughput next sequencing techniques, allowing patients to receive genetic evaluations at ever reducing costs (13). In Canada, genetic testing is available with government funding through targeted gene panel sequencing, which may include a comprehensive gene panel. In many instances the comprehensive panel is denied funding, therefore whole exome sequencing (WES) is completed at the patient’s expense, but more often through enrollment in a research project. There is mounting evidence that any child presenting with stone disease, particularly in the absence of obvious environmental precipitants, should undergo a full genetic workup as this is the only way to confirm a heritable nephrolithiasis disorders many of which pose a distinct risk for renal failure (71). It has been reported that of patients recruited from renal stones clinics, 14.9% had monogenic causation, and 40% had novel gene mutations associated with nephrolithiasis (72).
Next generation sequencing analysis in association with copy number variant analysis is currently the methods of evaluation of choice to identify a monogenic or single gene causes of kidney stone disease. Broadly, next generation sequencing in clinical practice methods include whole exome sequencing, whole genome sequencing (WGS) and targeted gene panel testing (73). WES is a method of determining the DNA sequence of the protein coding regions (exons) of the genes across the genome (74). WGS, on the other hand, sequences the genome in its entirety, including the protein coding regions (exons), non-coding regions (introns), as well as other non-genic portions of the genome (75). Both techniques facilitate the detection of variants in both known monogenic causes of kidney stone and both have the added advantage of allowing for novel gene detection (73). The use of WGS is increasing as it allows for evaluation for deep intronic variants and copy number variant analysis, both of which can be limited in standard exome analysis. Targeted gene panels increasingly is performed on an exome backbone but targets only specific gene know to be causative of the disease phenotype in question, therefore a high index of clinical suspicion for the underlying cause of disease is warranted pre-testing (76). The benefit of panel testing is that the data output is more simplified as analysis omit potentially less-relevant genes or regions of the genome thereby reducing the likelihood of detection of incidental findings (76). In recent years exome based analysis has largely replaced targeted gene panel tests, as it can detect the ever growing number of “new” kidney stone causing genes that are discovered across the genome (73). Figure 2 displays a potential diagnostic strategy for a pediatric nephrolithiasis population.
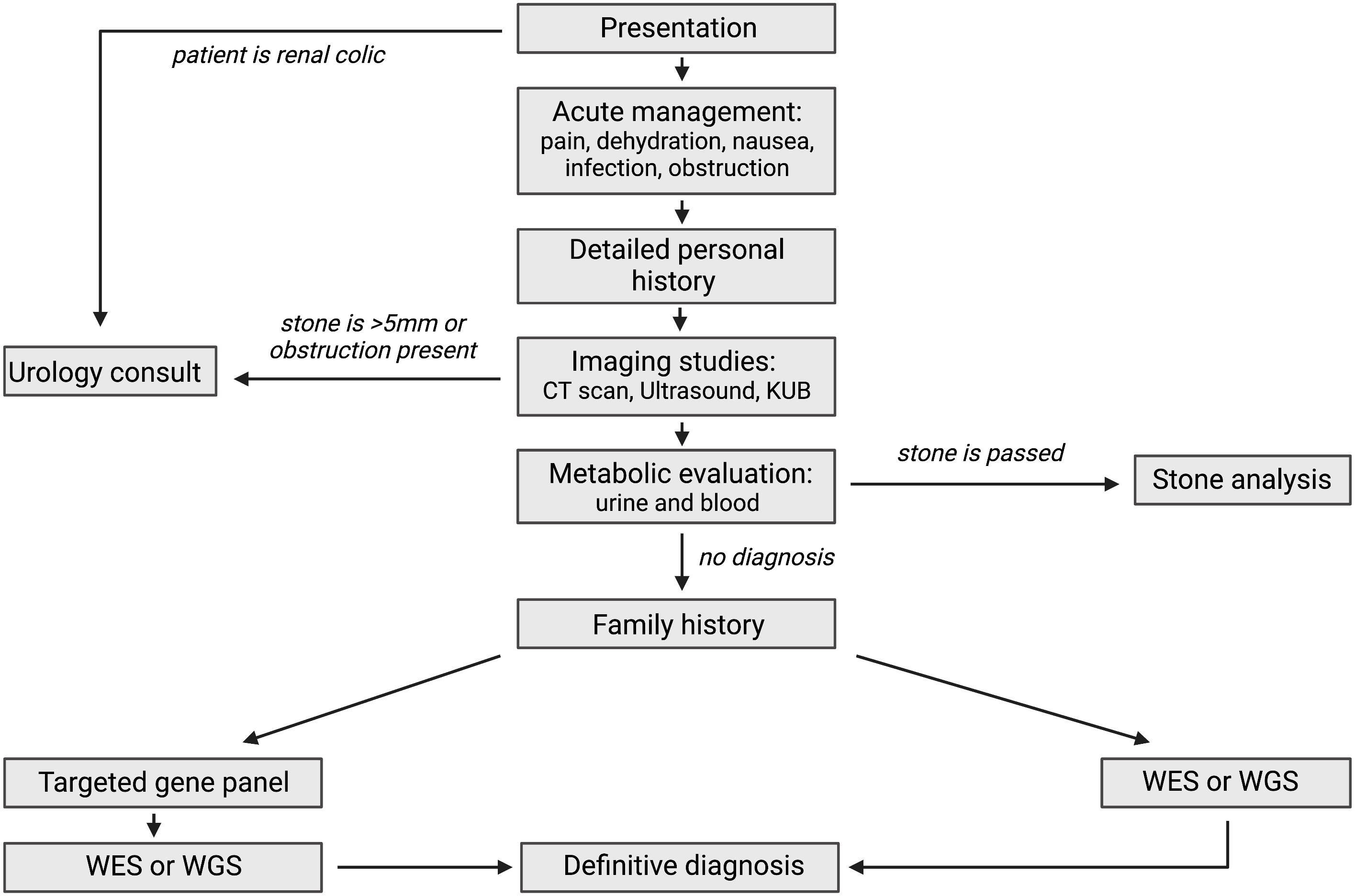
Figure 2 Complete overview of diagnostic methods for pediatric nephrolithiasis. KUB (kidney urinary bladder scan), WES (whole exome sequencing), WGS (whole genome sequencing). Created using BioRender.com (14).
Treatment
Pediatric nephrolithiasis comes with a variety of symptoms, or can be asymptomatic, leading to different treatment approaches depending on initial symptoms (5). Some stones are passed spontaneously, though studies have not supported the hypothesis that spontaneous passage occurs at a higher rate in pediatric patients compared to adults (68). Acute management of pediatric nephrolithiasis upon diagnosis includes pain control, observation, medical expulsive therapy (MET), and surgical intervention (77, 78). MET is frequently employed among pediatric patients, and includes alpha-blockers or calcium channel-blockers due to the theorization that they relax the distal ureter, facilitating stone passage (77). A few studies have supported this hypothesis, further promoting the efficacy of MET over analgesics alone (79, 80). Surgical intervention has been reported to be required in 22-60% of pediatric patients (81). Options for such procedures include extracorporeal shockwave lithotripsy (SWL), percutaneous nephrolithotomy (PCNL), retrograde intrarenal surgery (RIRS) with ureteroscopy (77). Pediatric nephrolithiasis cases specifically place patients in a high-risk category due to their high recurrence rates, significantly increasing the need for evaluation of risk factors (77). Metabolic abnormalities, anatomic abnormalities, as well as genetic conditions are among such factors (77). Ultimately, prevention of stone recurrence becomes the focus after acute management has been completed and depends on underlying causes of the formation of the stones. If metabolic conditions are known to underlie such cases, then dietary changes and pharmacological intervention are performed (77). Nevertheless, adequate fluid intake remains pivotal in the attempt to prevent stone recurrence (68).
Genetic causes of kidney stone disease
Monogenic kidney stone disease demonstrates a Mendelian pattern with autosomal dominant, autosomal recessive and X-linked of inheritance reported. In many of the pediatric populations reported to date, pathogenic mutations were more likely detected in those with younger age of onset of disease (<10 years) with an autosomal recessive inheritance observed in the majority of cases (65).
Currently, there are 41 known genes with monogenic causation for nephrolithiasis (Table 1). Most of these genes encode renal solute transporters including Solute Carrier Family 12 Member 1 (SLC12A1), and Solute Carrier Family 34 Member 3 (SLC34A3), along with many others (82). These proteins are divided into families but are largely responsible for the transport of different solutes including glucose, heavy and light subunits of heterodimeric amino acids, bicarbonate, sodium, chloride cations, organic ions, anions, and phosphate in the kidney (82). Another group of causative genes include a genes that code for the chloride channel, voltage-sensitive 5 (CLCN5), which encodes for tight-junction proteins including Claudin 16 (CLDN16) and Claudin 19 (CLDN19). Metabolizing enzymes like such as Alanine-glyoxylate Aminotransferase (AGXT) and Cytochrome P450, family 24, subfamily A, polypeptide 1 (CYP24A1) (13) have also been implicated in disease. Of the 41 nephrolithiasis causing genes, 4-Hydroxy-2-Oxoglutarate Aldolase 1 (HOGA1), AGXT, and Solute Carrier Family 3 Member 1 (SLC3A1) are most commonly reported in pediatric patients with nephrolithiasis, followed by mutations to Solute Carrier Family 7 member 9 (SLC7A9) and Glyoxylate reductase/hydroxypyruvate (GRHPR) (65). Additionally, Solute Carrier Family 34 Member 1 (SLC34A1), ATPase, H+ Transporting, Lysosomal 56/58kDa, V1 Subunit B1 (ATP6V1B1), CLCN5, CLDN16, AGXT and CYP24A1 are seen to cause early onset nephrolithiasis, all with an age of onset under 18 years (72). Many of these monogenic disease genes also contribute to individual monogenic forms of nephrolithiasis. Syndromic diseases including Bartter, Lowe, Dent, FHHNC and distal RTA have a recessive mode of inheritance, however heterozygous, dominant pathogenic mutations have been identified in individuals with milder clinical phenotypes (13). Here we will discuss individual monogenic forms of nephrolithiasis and nephrocalcinosis (Table 2).
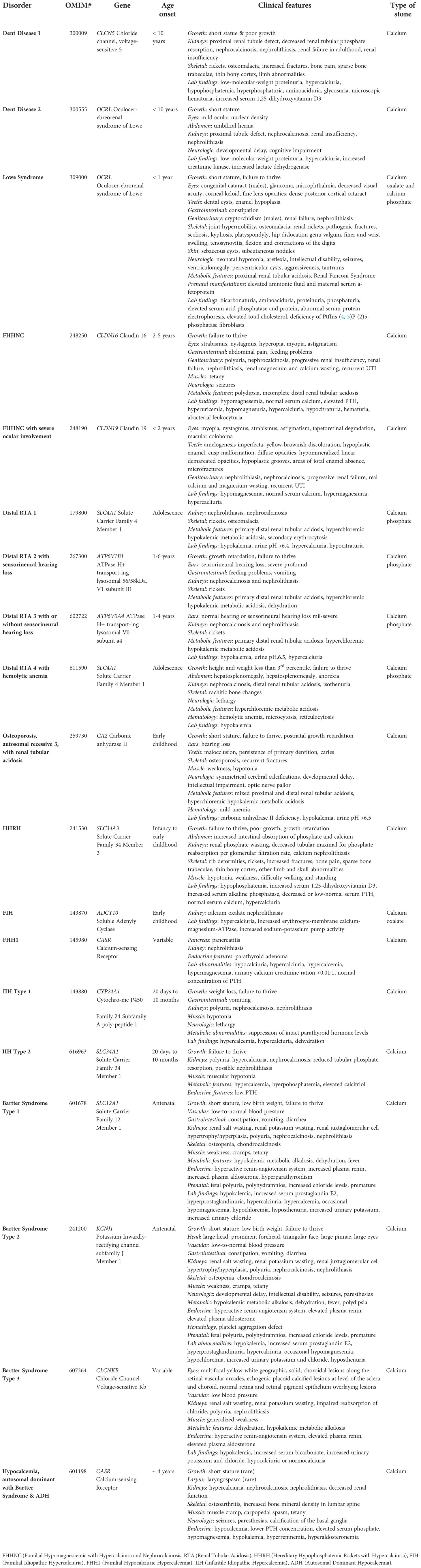
Table 2 Monogenic nephrolithiasis diseases. The table described the disorder, its OMIM number, gene, age of onset, clinical features, and stone type.
Though many monogenic forms of nephrolithiasis follow Mendelian genetics, incomplete penetrance and variable expressivity have been well described, which may complicate genetic evaluation and pedigree analysis. Briefly, penetrance is the likelihood a clinical condition will occur when a genotype is present, and is described as incomplete when not all individuals with a given genotype (i.e. a certain mutation in a disease causing gene) develop the condition (83). Variable expressivity is the range of symptoms individuals with the same genotype can experience (83). These phenomena are almost exclusively observed in autosomal dominant disease, an example of which is observed in primary hyperoxaluria (PH) (83) and mutations in the gene Alanine-glyoxylate Aminotransferase (AGXT), which is responsible for Primary hyperoxaluria type 1 (PH1) (83). Another gene, 4-Hydroxy-2-Oxoglutarate Aldolase 1 (HOGA1), which causes Primary hyperoxaluria type 3 (PH3), has alleles that are either fully penetrant or incompletely penetrant with the more penetrant mutations having stronger phenotypic effects (83). Studies have shown that variants of Solute Carrier Family 25 Member 25 (SLC25A25), which again cause dominant nephrolithiasis, can display incomplete penetrance (64).
Allelism is another concept of genetic variation where the type of mutation in a particular gene can determine the severity of the disease phenotype. Missense mutations are those which result in a single base pair substitution causing one amino acid change; truncating mutations cause the loss of several amino acids due to early termination of the protein (84). In general missense “milder” mutations are thought to confer a milder phenotype compared to truncating “severe” mutations, which tend to result in a more severe disease spectrum (85). This phenomena has also been observed in monogenic stone disease (86). Examples include Oculocerebrorenal Syndrome of Lowe (OCRL) which causes Dent Disease or Lowe Syndrome, depending on the location of the mutation within the OCRL gene (Figure 3), and Calcium-sensing Receptor (CASR) which causes Hypocalcemia with Bartter Syndrome, Familial Hypocalciuric Hypercalcemia (FHH), or Autosomal Dominant Hypocalcemia (ADH1) (86). Allelism therefore leads to variation in the clinical phenotype even among individuals with pathogenic mutations in the same gene (87).
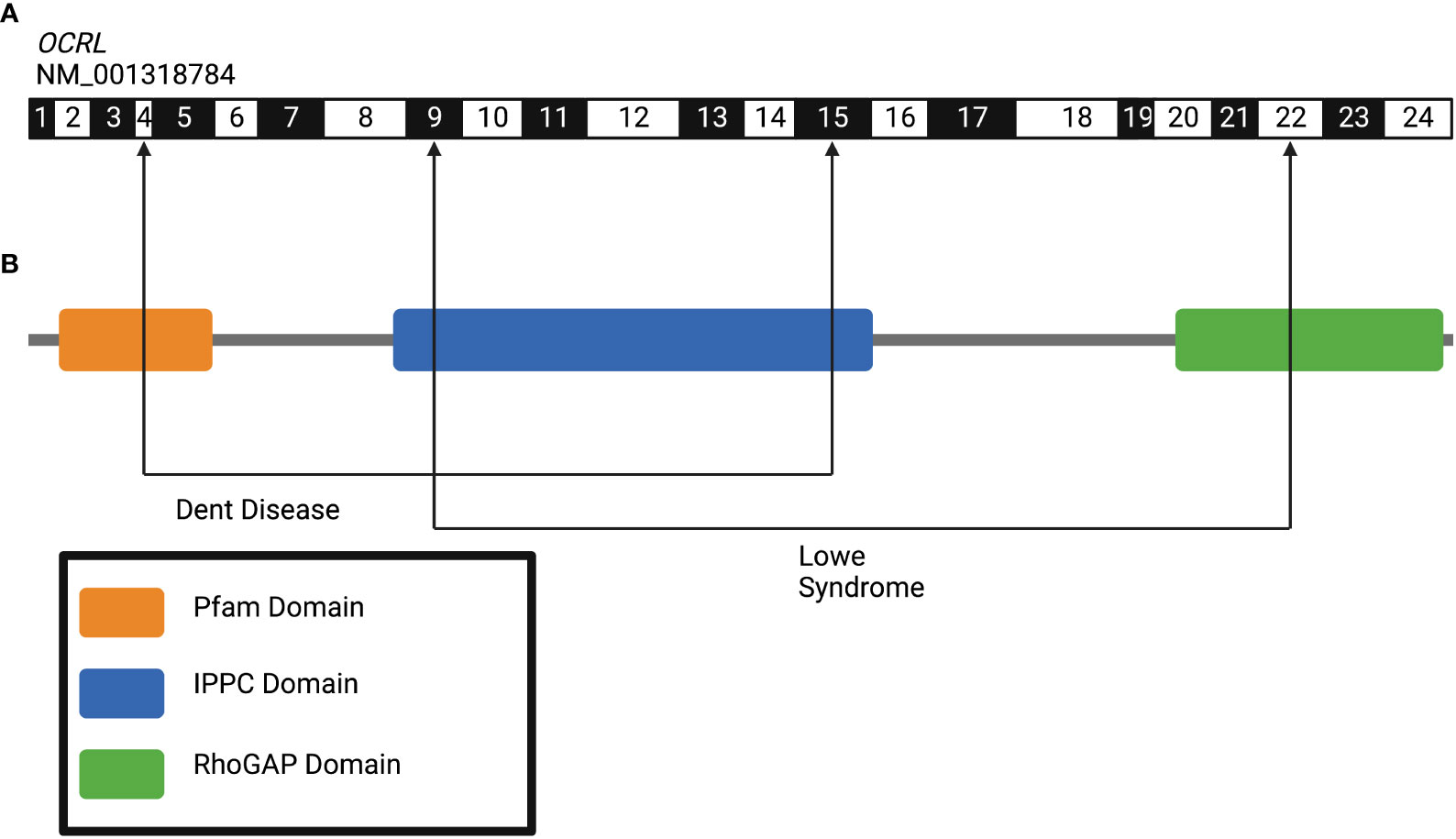
Figure 3 Allelism in the human gene Oculocerebrorenal Syndrome of Lowe (OCRL) gene leads to variation in clinical phenotypes of kidney disease. (A) Exon structure of human OCRL gene (NM_001318784) with arrowheads indicating exon positions wherein pathogenic variants lead to Dent disease (exons 4-15) and Lowe Syndrome (exon 9-22). (B) Protein domain structures of OCRL gene. Figure created using BioRender.com (14).
Dent disease
Dent disease is a familial renal tubular disorder with a X-linked recessive mode of inheritance (88). It is caused by mutations in the Chloride Channel, Voltage-Sensitive 5 (CLCN5), or Oculocerenrorenal Syndrome of Lowe (OCRL) (phosphatidylinositol 4,5-polyphosphate-5-phosphatase) (88). First discovered by Dent and Friedman in 1964, the disease is classified as hypercalciuric rickets associated with renal tubular damage (89). The clinical manifestations of this disease include poor growth, proximal renal tubule defect, decreased renal tubular phosphate reabsorption, nephrocalcinosis, nephrolithiasis, rickets, osteomalacia, increased fractures, bone pain, lower limb deformities, low weight proteinuria, hypercalciuria, hypophosphatemia, hyperphosphaturia, aminoaciduria, glycosuria, and microscopic hematuria (89, 90). Symptoms generally arise in males before the age of 10, and by the age of 30, 30-50% of patients are diagnosed with ESKD (86). Genetic testing has an important role in confirming diagnosis, and to determine the specific gene that is mutated (88). Since Dent Disease is a congenital genetic disease, available treatment focuses on relieving symptoms including preventing nephrolithiasis, and reduction of hypercalciuria (88). Approximately 50-60% of patients with Dent Disease have CLCN5 mutations, 15% have mutations to OCRL and the remaining 25% have possible defects in other genes (86). The majority of CLCN5 mutations are nonsense resulting in an early stop codon and truncated protein leading to loss of chloride conductance (86). Mutations in OCRL mainly occur in the 5’ region of the gene in exons 4 to 15 which is the phosphatase domain of the protein (86). Dent Disease is also considered to be a form of hereditary nephrocalcinosis (91). With the loss of renal chloride channel CLNC5 in the proximal tubule, there is a decrease in chloride reabsorption and therefore a decrease in calcium reabsorption, as well as prevention of charge dissipation creating an acidic environment, both leading to the development of calcium kidney stones (Figure 4) (91, 92). Previous studies have found that approximately 30% of patients with Dent Disease caused by CLCN5 mutations have nephrolithiasis, and this has an early age of onset with a mean of 23 (93). OCRL is involved in the trans-Golgi system, and in the processes of receptor endocytosis and recycling by proximal tubular cells (94). Wu et al. (2012) also described OCRL as an inhibitor of the Transient receptor potential vanilloid subfamily member 6 (TRPV6)calcium channel which could cause hypercalciuria and calcium nephrolithiasis due to increased calcium absorption (95).
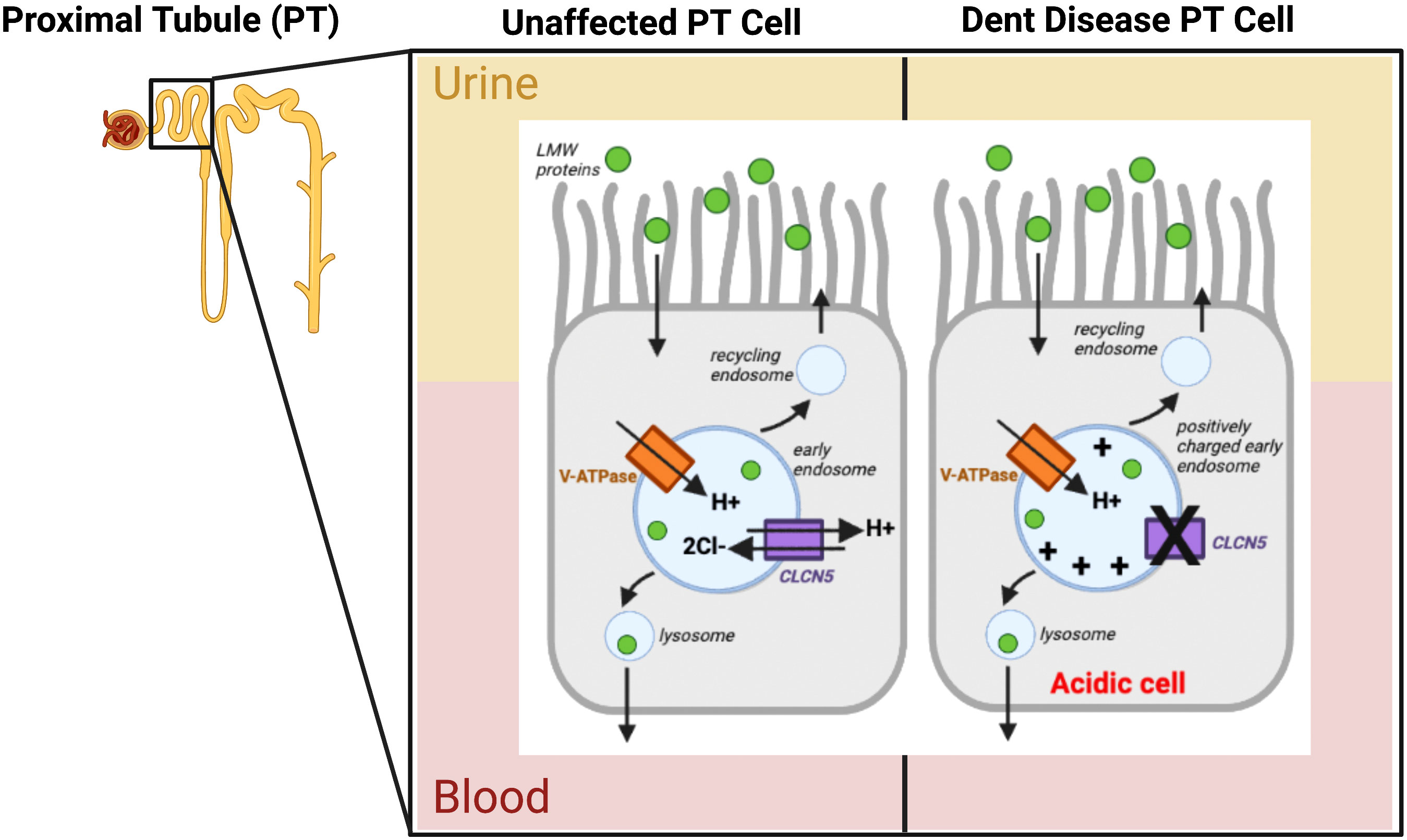
Figure 4 Pathophysiology of Dent Disease caused by Chloride channel, voltage-sensitive 5 (CLC5) mutation. The Vacuolar-type ATPase (V-ATPase) is represented by the orange rectangle, CLC5 is depicted by the purple rectangle, with their arrows representing the flow of ions into/out of the early endosome. The green circles represent low molecular weight proteins (LMW proteins) with an arrow showing their flow into the cell. The X in CLCN5 represents the dysfunctional protein, causing a charge build up in the early endosome and an overall acidic cell. PT cells are proximal tubule cells in the nephron of the kidney. Other arrows show the endosome cycle. Figure created using BioRender.com (14).
Lowe syndrome
Lowe syndrome is a rare multisystem disorder with X-linked recessive inheritance, affecting the eyes, nervous system, and kidneys (96). About 1 in 500,000 individuals suffer from Lowe Syndrome (96). This disorder is characterized clinically by symptoms including short stature, cataracts, glaucoma, renal failure, skeletal defects, osteomalacia, renal rickets, hypertonia, intellectual disability, seizures, Renal Fanconi syndrome, proximal renal tubular acidosis, bicarbonaturia, proteinuria, aminoaciduria, phosphaturia, elevated serum acid phosphatase, and elevated serum protein (97). In nearly half of nephrolithiasis cases, stones are composed of calcium oxalate and calcium phosphate (98). Most patients are in the pediatric population as Lowe Syndrome is a congenital genetic disorder (99). Life expectancy is no greater than 40 years, as the CKD is seen in the first year of life (stages 1 and 2) and progresses to stages 4 to 5 after age 20 (99). Treatment consists of removing cataracts, oral supplements of sodium and potassium bicarbonate or citrate to manage acidosis, oral supplementation of phosphate and calcitriol to manage hypophosphatemia and renal rickets, as well as growth hormones (100). The disorder is caused by mutations to the OCRL gene which encodes the protein phosphatidylinositol 4,5-polyphosphate-5-phosphatase (96). The OCRL gene on chromosome at position Xq26.1 when mutated causes a reduction in this protein and accumulation of the substrate phosphatidylinositol 4,5-polyphosphate (96). Affected patients have altered cell signaling pathways, defective actin cytoskeleton polymerization essential for formation of tight and adherens junctions in the renal proximal tubule, and protein trafficking abnormalities (96). Recent findings have also shown that OCRL is necessary for closure of endocytic vesicles which is important for recycling the megalin receptor in the proximal tubule (99). This defective recycling results in low molecular weight proteins in patients with Lowe syndrome (99). Due to allelism described above (Figure 3), pathogenic mutations in OCRL also cause Dent Disease. However, unlike Lowe Syndrome, patients do not have metabolic acidosis, ocular nor brain involvement (96). In patients with Lowe Syndrome, mutations to OCRL occur closer to the 3’ end of the gene in exons 9 to 22 which encode large functional domains (86). This contrasts Dent Disease which, as previously mentioned, has most deleterious mutations at the 5’ end of OCRL in exons 4 to 15 (86). Figure 3 depicts the OCRL exons and disease-causing regions. Due to the broad and variable symptoms of Lowe Syndrome, gene-targeted testing for OCRL (single-gene) or other potentially causative genes is important in providing a concrete diagnosis (100). Since this is an X-linked recessive disease, when a mother is a heterozygous carrier, there is a 25% chance of an affected son, and 25% chance of a heterozygous daughter (100). Some affected families may opt to do prenatal and preimplantation genetic testing, which is available once the familial OCRL pathogenic variant is detected (100).
Familial hypomagnesaemia hypercalciuria and nephrocalcinosis
FHHNC is an autosomal recessive renal tubular disorder characterized mainly by excessive calcium and magnesium excretion, and has early onset (101). Additionally, patients with FHHNC suffer from polyuria, nephrocalcinosis, bilateral nephrocalcinosis, hypomagnesemia, hyperuricemia, hypermegnesiuria, hypercalciuria, hypocitraturia, hematuria, recurrent urinary tract infection, nephrolithiasis, progressive chronic renal failure, seizures, and issues with eyesight (101). Due to progressive loss of kidney function, 50% of patients require renal replacement therapy as early as age 20 years (102). As with other rare genetic disorders, there is no specific treatment for FHHNC, though patients are given oral magnesium supplementation and thiazide diuretics to help combat the progression of nephrolithiasis and nephrocalcinosis, though this does not protect renal function (101). FHHNC is caused by mutations to members of the claudin protein family, CLDN16 and CLDN19 (102). Claudin proteins are transmembrane proteins required for tight junction barrier formation in epithelia (102). Both CLDN16 and CLDN19 are necessary for the reabsorption of calcium and magnesium into the blood (103). When these proteins are dysfunctional, calcium and magnesium build up in the urine and cause nephrolithiasis and nephrocalcinosis (Figure 5) (103). CLDN16 is located on chromosome 3q28 and is expressed exclusively in renal epithelial cells in the thick ascending limb of Henle and is important for reabsorption of divalent cations (104). Allelism has been in observed in patients with FHHNC. For example, a cohort of 69 patients with FHHNC due to pathogenic mutation to CLDN16 showed that the age of onset for complete loss-of-function mutations is 2.2 years compared to mutations resulting in partial loss-of-function, which is 5.6 years (105). CLDN19 is located on chromosome 1p34.2 and is expressed in the thick ascending limb of Henle as well as in the retinal pigment epithelium causing ocular defects in patients with CLDN19 mutation distinguishing them from CLDN16 patients (106). A study conducted by Val-Palomar found that in a Spanish cohort with 30 FHHNC patients who underwent exonic sequencing of CLDN19 and CLND16, 90% of the patients analyzed had a mutation in CLDN19 (106). Of the 90% with pathogenic CLDN19 mutations, 74% had the homozygous glycine to aspartic acid change at amino acid position 20 mutation (p.G20D) (106). This mutation is common in the South of Europe due to a common ancestor and has no change in CKD survival rate compared to patients with CLND19 mutations (106). The mean age of onset of disease was 1.71 years (106). Nephrolithiasis has been reported in FHHNC patients with CLDN16 and CLDN19 mutations at a rate of 25% and 42% respectively (107). Variable expressivity has been described in families with FHHNC mutations; some present with isolated nephrolithiasis and/or hypercalciuria, but no other symptoms, suggesting that CLDN16 may be associated with idiopathic calcium kidney stones (107). Recent research has also suggested CLDN14 as a possible risk factor for the development of calcium-containing stone (108). CLDN16 and CLDN19 complex with Claudin 3, allowing for cation permeability in the thick ascending limb (TAL) tight junction of the loop of Henle (109). Claudin 14 (CLDN14) interacts with Claudin 16 (CLDN16) allowing calcium to flow through the junction, but mutations to CLDN14 upregulate the protein and can cause nephrolithiasis (109). It has also been hypothesized that due to the interactions with CLDN16 and the calcium-sensing receptor CASR, overexpression of CLDN14 could cause hypomagnesemia, hypercalciuria and nephrocalcinosis as well, though this has not yet been related specifically to FHHNC (108).
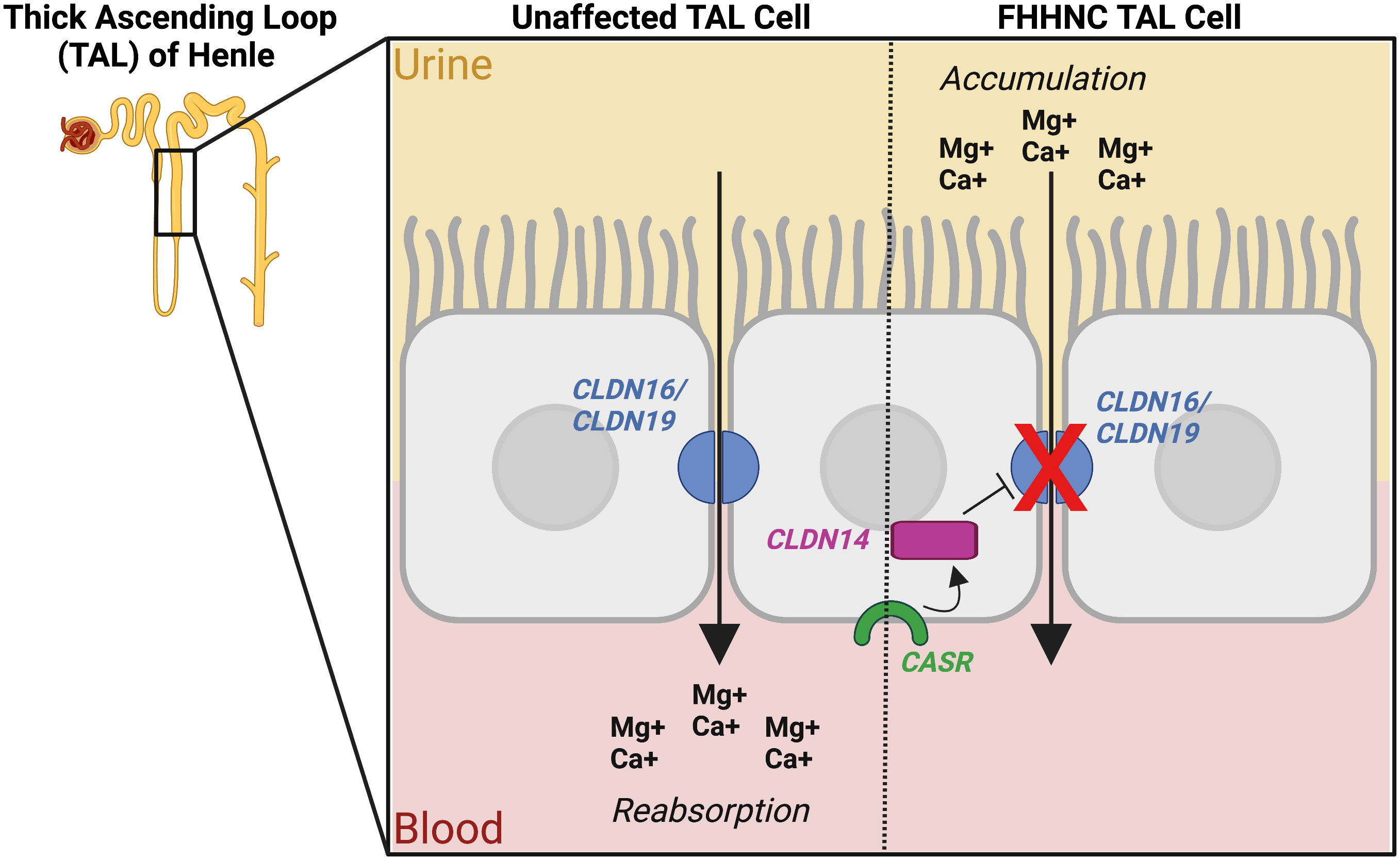
Figure 5 Pathophysiology of Familial Hypomagnesaemia Hypercalciuria and Nephrocalcinosis (FHHNC) caused by mutation in the genes Claudin 16 (CLDN16) and Claudin 19 (CLDN19). The figure also shows the inhibiting role of calcium-sensing receptor (CASR) and Claudin 14 (CLDN14) on CLDN16 and CLDN 19 in some cases. The blue semi circles represent the tight junctions formed by the CLDN16 and CLND19 proteins, the green curve depicts CASR, and the magenta rectangle represents CLDN14. The arrows depict the flow of ions from the urine, through the tight junctions between cells, to the blood, and the red X depicts a dysfunctional, mutated CLDN16 or CLDN19, preventing ion flow. TAL cell (Thick ascending loop of Henle cell, a cell found in the nephron of the kidney), Mg+ (Magnesium ion), Ca+ (Calcium ion). Figure created using BioRender.com (14).
Distal renal tubular acidosis
Distal RTA is an autosomal recessive or dominant kidney tubulopathy characterized by the inability to decrease urine pH to 5.3-5.5 when systemic metabolic acidosis is present (110). In this disease there is a defect in excreting H+ in the distal tubule and collecting duct of the nephron which leads to alkaline urine pH as well as calcium phosphate precipitation ultimately leading to kidney stones (111). General symptoms include nephrolithiasis, nephrocalcinosis, rickets, osteomalacia, primary distal renal tubular acidosis, hyperchloremic hypokalemic metabolic acidosis, urine pH>6.4 despite systemic acidosis, hypercalciuria, and hypocitraturia (110). Treatment of this disease includes alkali treatment which aims to correct metabolic acidosis and prevent rickets and stunted growth (112). There are three genes known to cause distal RTA including ATPase, H+ Transporting, Lysosomal V0 subunit A4 (ATP6V0A4), ATP6V1B1, and Solute Carrier Family 4, Anion Exchanger, Member 1 (SLC4A1) (110). ATP6V0A4 and ATP6V1B1 encode for different components of a multiunit V-ATPase enzyme that mediates acidification of eukaryotic intracellular compartments (110). In addition to the previously listed symptoms, individuals with a pathogenic or likely pathogenic mutation in ATP6V0A4 or ATP6V1B1 may also suffer from mild to severe levels of sensorineural hearing loss, as well as a higher risk in developing nephrolithiasis and CKD (112). The average age of diagnosis for distal RTA caused by mutation to ATP6V0A4 or ATP6V1B1 is 5.5 years, as clinical manifestations normally begin at infancy (112). A member from the solute carrier family, SLC4A1, encodes for a protein expressed in the erythrocyte plasma membrane and in kidney cells and acts as a chloride/bicarbonate transporter for carbon dioxide transport from tissues to lungs, and in tubular acid excretion respectively (113). Some mutations to SLC4A1 can therefore have pleiotropic effects in the kidney and red blood cells, which manifests as the autosomal recessive disease, distal RTA 4 with hemolytic anemia, while other mutations cause the autosomal dominant disease distal RTA 1 (113). For individuals with family history of pathogenic distal RTA mutations, prenatal genetic testing is available (114). Genetic testing to confirm the disease is also important since diagnosis can be challenging as it requires the physician’s conjecture and measurement of urinary pH after an acid load, which would normally lower urine pH to 5.3 (111, 114).
Renal tubular acidosis with osteoporosis
RTA is caused by tubulopathy that creates alkaline urine due to the inability to lower urinary pH (110). Osteoporosis is a common disease with little known about pathogenesis, although data now shows that acid-base balance has a profound impact on the homeostasis of bone calcium, in the presence of a positive acid balance, alkali is released from the bone (115). This prolonged release of alkali from the bone causes increased bone resorption and a decrease in total bone substance, increasing the likelihood of developing osteoporosis (115). The clinical manifestations of this autosomal recessive disease are outlines in Table 2. RTA with Osteoporosis is caused by a mutation to the gene Carbonic Anhydrase II (CA2), which encodes a protein that catalyzes reversible hydration of carbon dioxide (116). CA2 deficiency has also been determined to play a direct role in the loss of calcium from mineralized substrates and contributes to a rapid loss of bone mass, leading to osteoporosis (116). Both RTA type 1 (distal) and 2 (proximal) can lead to osteoporosis, though it is more common in type 2 (117). Osteoporosis is increasingly prevalent in the pediatric population due to increased disorders associated with bone loss including RTA with osteoporosis (118).
Hereditary hypophosphatemic rickets with hypercalciuria
HHRH is a rare autosomal recessive disorder caused by a defective sodium-phosphate co-transporter NPT2c due to loss-of-function mutations in the gene Solute Carrier Family 34 Member 3 (SLC34A3) (119). Pathogenic mutations in this gene are characterized by poor growth, renal phosphate wasting, decreased tubular maximum for phosphate reabsorption per glomerular filtration rate, calcium nephrolithiasis, rickets, increased fractures, bone pain, limb deformities, widened cranial sutures, muscle weakness, hypophosphatemia, increased serum 1,25-didroxyvitamin D3, increased serum alkaline phosphatase, decreased serum parathyroid hormone (PTH), and hypercalciuria (Figure 6) (119). The age of onset for HHRH is during infancy or early childhood. Treatment includes taking oral phosphate supplementation and potassium citrate, which can be enhanced by treatment with recombinant human growth hormone, fluconazole, and salt restriction (119). The solute transporter, SLC34A3, is expressed in proximal tubule cells of the kidney and transports phosphate into cells via sodium transport; its loss of function results in renal tubular defects (47). HHRH caused by mutations to both SLC34A3 alleles (i.e. recessive disease) increase the risk of kidney stones to 46% compared to the general population risk of approximately 10% (120). It has been determined that heterozygous carriers of SLC34A3 mutations can also develop isolated nephrolithiasis (120). Mutational screening for pathogenic variants in genes either through whole exome sequencing or gene panel testing is the method of choice to confirm the diagnosis (121). Genetic testing is also important in the family members since heterozygous mutations can also lead to stone disease, owing to the loss-of-function nature of SLC34A3 (121).
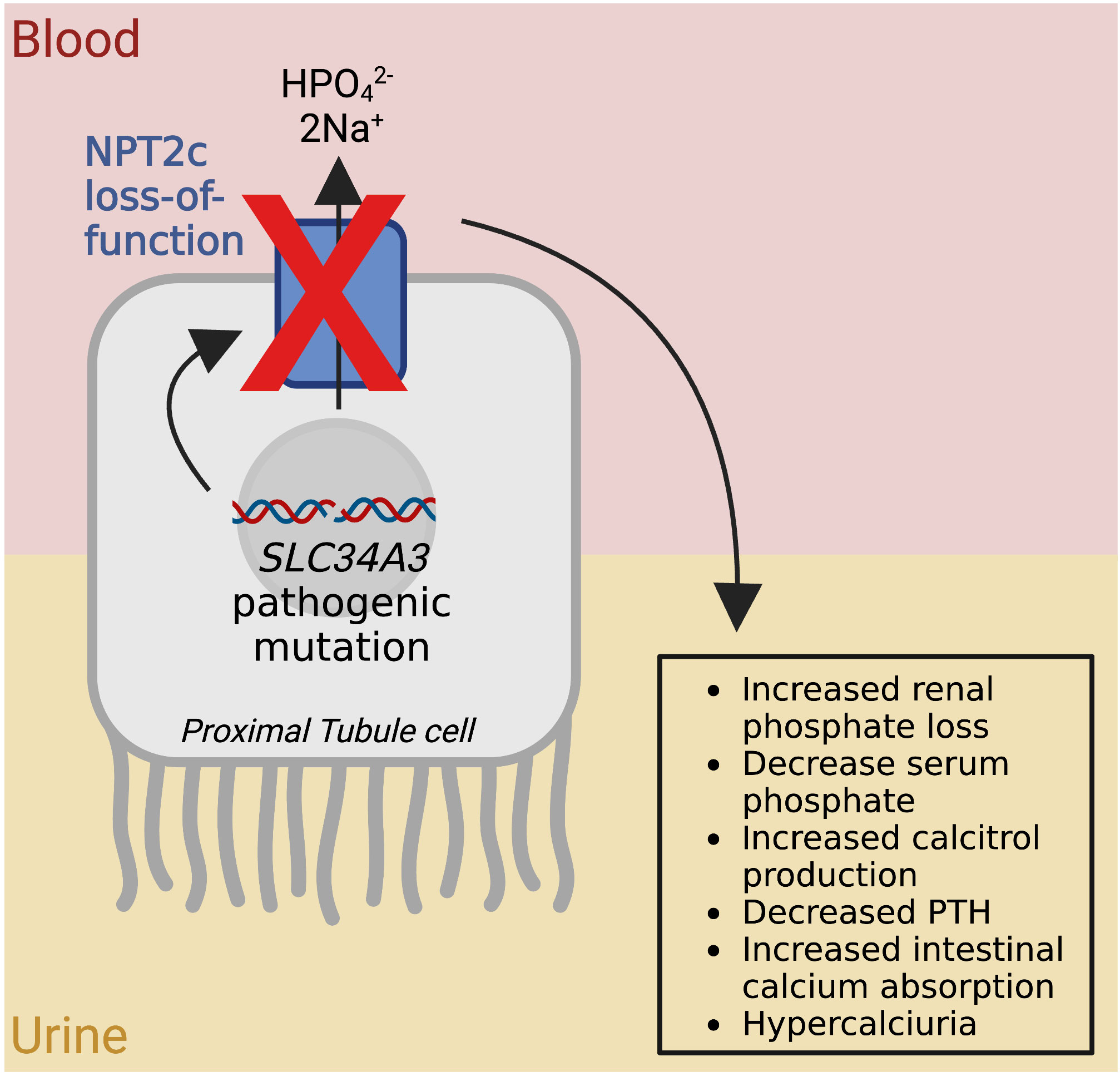
Figure 6 Pathophysiology of Hereditary Hypophosphatemic Rickets with Hypercalciuria. The red X represents the loss of function of the Type IIc Sodium-Dependent Phosphate Transporter (NPT2c) shown as a blue rectangle, due to a mutation to the Solute Carrier Family 34 Member 3 gene (SLC34A3). The arrow through NPT2c represents the flow of ions, and the other arrows show the flow from genotype to phenotype. HPO42- (hydrogen phosphate), 2Na+ (2 sodium ions). Figure created using BioRender.com (14).
Hypercalciuria
Hypercalciuria is a metabolic abnormality that causes excess calcium in the urine and is closely linked to causing calcium oxalate stones (122). There are many genetic disorders which are related to hypercalciuria and nephrolithiasis, including Dent Disease, FHHNC, HHRH, Autosomal Dominant Hypocalcemic Hypercalciuria (ADHH) and some forms of Bartter Syndrome, which are discussed in this review (122). Another form of hypercalciuria called Familial Idiopathic Hypercalciuria (FIH) is dominantly inherited and is caused by mutation to the gene, Soluble Adenyly Cyclase (ADCY10). The role of the ADCY10 protein is to catalyze the formation of the cAMP (123). FIH is common in children, and the main goal of treatment is to prevent recurrence of stones by reducing urinary calcium oxalate which can be accomplished by increasing fluid intake, low-salt diet, and some drugs including thiazides, diuretics, and potassium citrate (124). It has also been shown that in 69% of pediatric patients who suffered from nephrolithiasis and hypercalciuria there is a family history of the disorders (125).
Hypercalcemia
Hypercalcemia occurs when calcium is two standard deviations above normal calcium levels over a period of three months (126). Genetic forms of hypercalcemia including Familial Hypocalciuric Hypercalcemia (FHH), and Infantile Idiopathic Hypercalcemia (IIH) (126).
FHH follows an autosomal dominant pattern with 65% of cases being caused by heterozygous inactivating mutations of the CASR on chromosome 3, and has variable age of onset (127). FHH is normally benign and asymptomatic, therefore diagnosis may be missed, or misdiagnosed as Primary Hyperparathyroidism which can result in unwarranted treatment or surgery if not correctly classified (127). Features of disease may include nephrolithiasis, pancreatitis, parathyroid adenoma, hypocalciuria, hypercalciuria, hypercalcemia, hypermagnesemia, and normal PTH (127). Most FHH cases do not require treatment, though calcimimetic drugs may be used if patients suffer from pancreatitis (127). Most cases of FHH that lead to death are due to improper and unnecessary treatment and surgery, which is why genetic testing for CASR is crucial (127).
Infantile Idiopathic Hypercalcemia (IIH) is an autosomal recessive disorder that is characterized by severe hypercalcemia (128). There are two types of IIH, type one is caused by mutation in the Cytochrome P450, Family 24, Subfamily A, Polypeptide 1 (CYP24A1), and type two is caused by mutations in the Solute Carrier Family 34 (SLC34A1) (128). General symptoms include failure to thrive, polyuria, nephrocalcinosis, hypotonia, hypercalciuria and hypercalcemia (128). Additionally, patients with Type 1 IIH may experience weight loss, vomiting, nephrolithiasis, central nervous system lethargy, and dehydration, whereas patients with Type 2 IIH may experience reduced tubular phosphate reabsorption, hypophosphatermia, elevated calcitriol, and low PTH (128). The age of onset for IIH is between 20 days and 10 months with failure to thrive and polyuria the most common presenting symptoms (128). There is strong evidence exhibiting nephrolithiasis in IIH Type 1 patients, though further research is needed to address the association of nephrolithiasis to IIH Type 2 (128).
Bartter syndrome
Bartter Syndrome is composed of a group of rare autosomal recessive genetic disorders causing defects in the kidney’s ability to reabsorb salt in the thick ascending loop of Henle (129). Bartter Syndrome is characterized by renal salt wasting, polyuria, hypokalemic metabolic alkalosis, elevated renin and aldosterone levels, and variable risk of developing kidney stones (129). There are 6 types of Bartter Syndrome with varying symptoms, and causative genes (129). Bartter Syndrome I (BARTS1) is antenatal in onset and is caused by mutation to the gene, SLC12A1 (129). BARTS1 is a life-threatening form of the disease which presents with intrauterine growth retardation, premature delivery, low birth weight, failure to thrive, severe dehydration in the neonatal period, hypochloremia, hypercalciuria, hypercalcemia, increased urinary potassium and chloride, nephrocalcinosis, muscle weakness and cramping, as well as developmental delay (130). Currently, there are over 100 described mutations in the gene SLC12A1 described in patients with BARTS1 (131). Bartter Syndrome II (BARTS2) is caused by mutations to Potassium Channel Inwardly Rectifying Subfamily J, Member 1 (KCNJ1), a gene that codes for the renal outer medullary potassium channel, ROMK, which controls resting potential, membrane excitability and is necessary for sodium chloride reabsorption in the ascending loop of Henle (132). Infants presenting with BARTS2 have polyhydramnios and premature delivery, failure to thrive, dehydration, nephrocalcinosis, muscle weakness, hypercalciuria, increased urine potassium and chloride, hyposthenuria, hyperprostaglandinuria, large head, prominent forehead, and large eyes, as well as neurological issues and muscle weakness and cramps (133). Studies have shown that 1 in 5 patients with BARTS2 also have nephrolithiasis (134). Although it is uncommon, there have been few cases reporting late-onset BARTS2 in adolescence and adulthood (132). Bartter Syndrome III (BARTS3) is caused by mutations to the kidney Chloride Channel, Voltage-sensitive Kb (CLCNKB), resulting in reduction of chloride and sodium reabsorption in renal tubules and subsequent loss of salt in urine (63). Additional symptoms of individuals with BARTS3 include low blood pressure, choroidal lesions and placoid calcified lesions to the eye, renal potassium wasting, impaired reabsorption of chloride, muscle weakness, dehydration, and increased serum bicarbonate, urinary potassium, and chloride (63). Pathogenic mutations in CLCNKB have also been proven to cause hypercalciuric nephrolithiasis (79). Previous work by Cheng et al. correlated severity of CLCNKB genotypes to age of onset, plasma chloride concentration, and urine excretion rate: milder missense mutations have a later age of onset (135), while truncating variants of CLCNKB cause a more severe, early onset BARTS3 phenotype (63). Bartter Syndrome IVA is caused by mutations to the gene Barttin CLCNK-type Accessory Subunit Beta, BSND. This disease normally develops in neonates during the second trimester (136). Infants with this disorder have additional symptoms including failure to thrive, sensorineural deafness, inability to concentrate urine, decreased glomerular filtration rate, renal failure, glomerulosclerosis, intellectual and motor disability, hyponatremia, hypochloremia, as well as increased urinary sodium, potassium, and chloride (136). Bartter Syndrome IVB is a digenic disorder caused by mutations to both kidney chloride channels, CLCNKB and Chloride Voltage-gated Channel Ka (CLCNKA) (129). Bartter Syndrome type 5 (BARTS5) is caused by X-linked recessive mutation of the gene Melanoma Antigen, Family D2 (MAGED2), and is a severe antenatal form of Bartter Syndrome (137). Almost all infants born with BARTS5 are premature, with 38% being born before gestational week 8 (137). These patients have a very high mortality rate and additional symptoms including severe renal sodium and chloride loss, hypercalciuria, nephrocalcinosis, preterm delivery, early onset severe polyhydramnios, hyponatremia hypochloremia, hyperreninemia, and elevated calcium to creatinine ratio (137). Prenatal genetic testing for MAGED2 is important to prevent unnecessary diagnostic measures and harmful treatment in pregnant women (137). Studies have also classified an autosomal dominant form of BARTS5 as Hypocalcemia with Bartter Syndrome, which is caused by mutation to the gene CASR, and symptoms include kidney stones (129).
Hypocalcemia
Hypocalcemia is an electrolyte imbalance that can be potentially fatal, and in children, persistent hypocalcemia can be detrimental to bone growth and overall health (138). Patients with hypocalcemia have symptoms including short stature, laryngospasm, hypercalciuria, nephrocalcinosis, nephrolithiasis, decreased renal function, osteoarthritis, seizures, paresthesia, hypocalcemia, low PTH concentration, mildly elevated serum phosphate, hypomagnesemia, hypokalemia, hyperreninemia, and hyperaldosteronemia (139). Treatment for hypocalcemia aims to raise serum calcium to reduce symptoms therefore knowing the etiology of the disease is essential (139). Heterozygous mutations to the calcium-sensing receptor, CASR, cause genetic forms of hypocalemia (140). The protein encoded by CASR is a plasma membrane G-protein-coupled receptor that senses changes in circulating calcium concentration, coupling this information with parathyroid hormone regulatory pathways (140). A genetic form of hypocalcemia is ADHH (122). These patients have mild hypocalcemia that is generally asymptomatic but is can be associated with carpo-pedal spasm and seizures. The causative mutations is a gain of function mutation in the CASR gene (122), leading to hypercalciuria in these patients and subsequently nephrolithiasis (141). There are over 40 different CASR mutations, with many families having a unique deleterious mutation (122). Some forms of hypocalcemia do not have hypercalciuria, which is classified as Autosomal Dominant Hypocalcemia (ADH) (142). A study by Roszko showed that the 81% of patients were diagnosed with ADH1 before the age of 18, and the median age for diagnosis with hypocalcemia-related disorders is 4 years (141). These patients also have mutations to CASR which causes its activation and can lead to development of ADH with BARTS5 (142). About 50% of patients with ADH have symptomatic hypocalcemia, and over 30% have intracerebral calcifications (142). Patients with ADH are treated with calcium supplements and active vitamin D metabolites to raise serum calcium, though this in itself can predispose patients to development of nephrolithiasis, as well as nephrocalcinosis and hypercalciuria (142). Genetic testing has an important place in diagnosis and treatment for the various types of hypocalcemia and should be performed along with genetic counselling for result interpretation and subsequent disclosures and explanations (141).
Conclusion
Nephrolithiasis is at an all-time high, with an increasing incidence in the pediatric population. Monogenic causation accounts for 30% of all nephrolithiasis cases, with a total of 41 known pathogenic genes. Mutations in a causative gene can lead to various monogenic nephrolithiasis disorders including Dent disease, Lowe Syndrome, FHHNC, RTA, RTA with osteoporosis, HHRH, hypercalciuria, hypercalcemia, Bartter Syndrome, and hypocalcemia. These diseases pose their own unique risks and symptoms associated with nephrolithiasis and diagnosis can be verified through genetic testing. There is a high inheritance correlation with a 3-fold increase in nephrolithiasis development in children with a family history. Nephrolithiasis may lead to CKD and ESKD, which is why precise diagnosis and treatment is vital. Current CUA guidelines do not recommend genetic testing as a first line diagnostic testing strategy for patients with isolated nephrolithiasis, though it is listed as a future direction to diagnose and treat genetic stones diseases (70). There is also increasing evidence that early diagnosis through genetic testing in the pediatric population may prevent the development of chronic kidney disease. Genetic testing options are now more available at ever reducing costs and include single gene testing, panel testing and large scale genomic testings such as exome or genome sequencing. With increasing available is it however imperative that all genetic testing and the subsequent interpretation of the results adheres to international guidelines. The American College of Medical Genetics and Genomics (ACMG) have published internationally accepted guidelines on how to determine whether a genetic finding is indeed disease causing. The ACMG recommend that all clinical molecular genetic tests fall into the pathogenic or likely pathogenic category (143). In addition, it is also recommended that all clinical molecular genetics results be reviewed and interpreted by a multidisciplinary team with an expertise in clinical genetics, and that pre- and post-counselling is provided to patients as necessary (143). With the growing numbers of novel genes and increased availability of genomic testing, both exome and genome sequencing is now proven techniques for detection of genetic kidney stones in pediatric populations and represents a future option for in the diagnosis and individualized treatment plans for children with suspected monogenic kidney stone disease.
Author contributions
CS conducted thorough literature review, developed Figures 1–6, edited Table 1, developed Table 2, wrote the drafts, carefully edited and revised various versions of the manuscript, collated all changes, added intellectual content, and approved the final manuscript. AP conducted thorough literature review, created Figure 3, aided in writing and editing the drafts, added intellectual content, approved the final manuscript. DMC conceived this project, added major intellectual input, provided Table 1, made multiple edits, approved the final manuscript.
Funding
Financial support for this project was provided by the Department of Medicine, Schulich School of Medicine and Dentistry, University of Western Ontario. DMC is funded by the Eugen Drewlo Chair for Kidney Research and Innovation at the Schulich School of Medicine & Dentistry at Western University, London, Ontario, Canada, and the Academic Medical Organization of Southwestern Ontario (AMOSO) Innovation Fund.
Conflict of interest
The authors declare that the research was conducted in the absence of any commercial or financial relationships that could be construed as a potential conflict of interest.
The reviewer PW declared a shared affiliation with the authors to the handling editor at the time of review.
Publisher’s note
All claims expressed in this article are solely those of the authors and do not necessarily represent those of their affiliated organizations, or those of the publisher, the editors and the reviewers. Any product that may be evaluated in this article, or claim that may be made by its manufacturer, is not guaranteed or endorsed by the publisher.
References
1. Finlayson B. Physicochemical aspects of urolithiasis. Kidney Int (1978) 13(5):344–60. doi: 10.1038/ki.1978.53
2. Evan AP. Physiopathology and etiology of stone formation in the kidney and the urinary tract. Pediatr Nephrol (2010) 25(5):831–41. doi: 10.1007/s00467-009-1116-y
3. Ferraro PM, Bargagli M, Trinchieri A, Gambaro G. Risk of kidney stones: Influence of dietary factors, dietary patterns, and vegetarian–vegan diets. Nutrients (2020) 12(3):779. doi: 10.3390/nu12030779
4. Evan AP, Worcester EM, Coe F, W. JJR. Mechanisms of human kidney stone formation. Urolithiasis (2016) 43(1):19–32. doi: 10.1007/s00240-014-0701-0
5. Fontenelle LF, Sarti TD. Kidney Stones: Treatment and Prevention. Am Fam Phys (2019) 99(8):491–6.
6. Miah T, Kamat D. Pediatric nephrolithiasis: A review. Pediatr Ann (2017) 46(6):e242–4. doi: 10.3928/19382359-20170517-02
7. Dhondup T. Risk of ESRD and mortality in kidney and bladder stone formers. AJKD (2018) 72:8. doi: 10.1053/j.ajkd.2018.06.012
8. Arora V, Anand K, Chander Verma I. Genetic testing in pediatric kidney disease. Indian J Pediatr (2020) 87(9):706–15. doi: 10.1007/s12098-020-03198-y
9. Scales CD, Smith AC, Hanley JM, Saigal CS. Urologic diseases in America project. prevalence of kidney stones in the united states. Eur Urol (2012) 62(1):160–5. doi: 10.1016/j.eururo.2012.03.052
10. Ward JB, Feinstein L, Pierce C, Lim J, Abbott KC, Bavendam T, et al. Pediatric urinary stone disease in the United States: The Urologic Diseases in America Project. Pediatric Urol (2020) 4:180–7. doi: 10.1016/j.urology.2019.04.012
11. Dwyer ME, Krambeck AE, Bergstralh EJ, Milliner DS, Lieske JC, Rule AD. Temporal trends in incidence of kidney stones among children: A 25-year population based study. J Urol (2012) 188(1):247–52. doi: 10.1016/j.juro.2012.03.021
12. Khan SR, Pearle MS, Robertson WG, Gambaro G, Canales BK, Doizi S, et al. Kidney stones. Nat Rev Dis Primers (2016) 2(1):16008. doi: 10.1038/nrdp.2016.8
13. Halbritter J. Update on hereditary kidney stone disease and introduction of a new clinical patient registry in Germany. Front Pediatr (2018) 6:7. doi: 10.3389/fped.2018.00047
14. BioRender. Available at: https://app.biorender.com/.
15. Moe OW. Kidney stones: pathophysiology and medical management. Lancet (2006) 367:12. doi: 10.1016/S0140-6736(06)68071-9
16. Borghi L, Schianchi T, Meschi T, Guerra A, Allegri F, Maggiore U, et al. Comparison of two diets for the prevention of recurrent stones in idiopathic hypercalciuria. N Engl J Med (2002) 346(2):77–84. doi: 10.1056/NEJMoa010369
17. Pak CYC. Citrate and renal calculi: New insights and future directions. Am J Kidney Diseases (1991) 17(4):420–5. doi: 10.1016/S0272-6386(12)80635-4
18. Daga A, Majmundar AJ, Braun DA, Gee HY, Lawson JA, Shril S, et al. Whole exome sequencing frequently detects a monogenic cause in early onset nephrolithiasis and nephrocalcinosis. Kidney Int (2018) 93(1):204–13. doi: 10.1016/j.kint.2017.06.025
19. Fink HA, Wilt TJ, Eidman KE, Garimella PS, MacDonald R, Rutks IR, et al. Medical management to prevent recurrent nephrolithiasis in adults: a systematic review for an American college of physicians clinical guideline. Ann Intern Med (2013) 158(7):535–43. doi: 10.7326/0003-4819-158-7-201304020-00005
20. Lieske JC, Wang X. Heritable traits that contribute to nephrolithiasis. Urolithiasis (2020) 47(1):5–10. doi: 10.1007/s00240-018-1095-1.
21. Howles SA. Genetics of kidney stone disease. Nature Reviews Urology (2022) 17:407–21. doi: 10.1038/s41585-020-0332-x
22. Singh P, Harris PC, Sas DJ, Lieske JC. The genetics of kidney stone disease and nephrocalcinosis. Nat Rev Nephrol (2022) 18(4):224–40. doi: 10.1038/s41581-021-00513-4
23. Gambaro G, Vezzoli G, Casari G, Rampoldi L, D’Angelo A, Borghi L. Genetics of hypercalciuria and calcium nephrolithiasis: From the rare monogenic to the common polygenic forms. Am J Kidney Diseases (2004) 44(6):963–86. doi: 10.1053/j.ajkd.2004.06.030
24. de Castro JM. Genetic influences on daily intake and meal patterns of humans. Physiol Behavior (1993) 53(4):777–82. doi: 10.1016/0031-9384(93)90188-L
25. Huang L, Qi C, Zhu G, Ding J, Yuan L, Sun J, et al. Genetic testing enables a precision medicine approach for nephrolithiasis and nephrocalcinosis in pediatrics: a single-center cohort. Mol Genet Genomics (2022) 297(4):1049–61. doi: 10.1007/s00438-022-01897-z
26. Purdue PE, Allsop J, Isaya G, Rosenberg LE, Danpure CJ. Mistargeting of peroxisomal l-alanine:glyoxylate aminotransferase to mitochondria in primary hyperoxaluria patients depends upon activation of a cryptic mitochondrial targeting sequence by a point mutation. Proc Natl Acad Sci USA (1991) 88(23):10900–4. doi: 10.1073/pnas.88.23.10900
27. Paolella G, Santamaria R, Buono P, Salvatore F. Mapping of a restriction fragment length polymorphism within the human aldolase b gene. Hum Genet (1987) 77(2):115–7. doi: 10.1007/BF00272375
28. Weiss MJ, Cole DE, Ray K, Whyte MP, Lafferty MA, Mulivor RA, et al. A missense mutation in the human liver/bone/kidney alkaline phosphatase gene causing a lethal form of hypophosphatasia. Proc Natl Acad Sci USA (1988) 85(20):7666–9. doi: 10.1073/pnas.85.20.7666
29. Hidaka Y, Palella TD, O’Toole TE, Tarlé SA, Kelley WN. Human adenine phosphoribosyltransferase. identification of allelic mutations at the nucleotide level as a cause of complete deficiency of the enzyme. J Clin Invest (1987) 80(5):1409–15.
30. Smith AN, Skaug J, Choate KA, Nayir A, Bakkaloglu A, Ozen S, et al. Mutations in ATP6N1B, encoding a new kidney vacuolar proton pump 116-kD subunit, cause recessive distal renal tubular acidosis with preserved hearing. Nat Genet (2000) 26(1):71–5. doi: 10.1038/79208
31. Karet FE, Finberg KE, Nelson RD, Nayir A, Mocan H, Sanjad SA, et al. Mutations in the gene encoding B1 subunit of h+-ATPase cause renal tubular acidosis with sensorineural deafness. Nat Genet (1999) 21(1):84–90. doi: 10.1038/5022
32. Gromadzka G, Schmidt HHJ, Genschel J, Bochow B, Rodo M, Tarnacka B, et al. Frameshift and nonsense mutations in the gene for ATPase7B are associated with severe impairment of copper metabolism and with an early clinical manifestation of wilson’s disease: Genotype and phenotype in wilson’s disease. Clin Genet (2005) 68(6):524–32. doi: 10.1111/j.1399-0004.2005.00528.x
33. Venta PJ, Tashian RE. Carbonic anhydrase 11 deficiency syndrome in a Belgian family is caused by a point mutation at an invariant histidine residue (107 his–tyr): Complete structure of the normal human CA 11 gene. Am J Hum Genet (1991) 49:1082–90.
34. Simon DB, Karet FE, Hamdan JM, Pietro AD, Sanjad SA, Lifton RP. Bartter’s syndrome, hypokalaemic alkalosis with hypercalciuria, is caused by mutations in the Na–K–2CI cotransporter NKCC2. Nat Genet (1996) 13(2):183–8. doi: 10.1038/ng0696-183
35. Simon DB, Lu Y, Choate KA, Velazquez H, Al-Sabban E, Praga M, et al. Paracellin-1, a renal tight junction protein required for paracellular mg 2+ resorption. Science (1999) 285(5424):103–6. doi: 10.1126/science.285.5424.103
36. Konrad M, Schaller A, Seelow D, Pandey AV, Waldegger S, Lesslauer A, et al. Mutations in the tight-junction gene claudin 19 (CLDN19) are associated with renal magnesium wasting, renal failure, and severe ocular involvement. Am J Hum Genet (2006) 79(5):949–57. doi: 10.1086/508617
37. Town M, Jean G, Cherqui S, Attard M, Forestier L, Whitmore SA, et al. A novel gene encoding an integral membrane protein is mutated in nephropathic cystinosis. Nat Genet (1998) 18(4):319–24. doi: 10.1038/ng0498-319
38. Schlingmann KP, Kaufmann M, Weber S, Irwin A, Goos C, John U, et al. Mutations in CYP24A1 and idiopathic infantile hypercalcemia. N Engl J Med (2011) 365(5):410–21. doi: 10.1056/NEJMoa1103864
39. Aponte JL, Sega GA, Hauser LJ, Dhar MS, Withrow CM, Carpenter DA, et al. Point mutations in the murine fumarylacetoacetate hydrolase gene: Animal models for the human genetic disorder hereditary tyrosinemia type 1. Proc Natl Acad Sci U S A (2001) 98(2):641–5. doi: 10.1073/pnas.98.2.641
40. Jaureguiberry G, de la Dure-Molla M, Parry D, Quentric M, Himmerkus N, Koike T, et al. Nephrocalcinosis (Enamel renal syndrome) caused by autosomal recessive FAM20A mutations. Nephron Physiol (2013) 122(1–2):1–6. doi: 10.1159/000349989
41. Seydewitz HH, Matern D. Molecular genetic analysis of 40 patients with glycogen storage disease type ia: 100% mutation detection rate and 5 novel mutations. Hum Mutat (2000) 15(1):115–6. doi: 10.1002/(SICI)1098-1004(200001)15:1<115::AID-HUMU23>3.0.CO;2-W
42. Cramer S. The gene encoding hydroxypyruvate reductase (GRHPR) is mutated in patients with primary hyperoxaluria type II. Hum Mol Genet (1999) 8(11):2063–9. doi: 10.1093/hmg/8.11.2063
43. Belostotsky R, Seboun E, Idelson GH, Milliner DS, Becker-Cohen R, Rinat C, et al. Mutations in DHDPSL are responsible for primary hyperoxaluria type III. Am J Hum Genet (2010) 87(3):392–9. doi: 10.1016/j.ajhg.2010.07.023
44. Bockenhauer D, Feather S, Stanescu HC, Bandulik S, Zdebik AA, Reichold M, et al. Epilepsy, ataxia, sensorineural deafness, tubulopathy, and KCNJ10 mutations. N Engl J Med (2009) 360(19):1960–70. doi: 10.1056/NEJMoa0810276
45. Gee HY, Jun I, Braun DA, Lawson JA, Halbritter J, Shril S, et al. Mutations in SLC26A1 cause nephrolithiasis. Am J Hum Genet (2016) 98(6):1228–34. doi: 10.1016/j.ajhg.2016.03.026
46. Akagi M, Inui K, Nakajima S, Shima M, Nishigaki T, Muramatsu T, et al. Mutation analysis of two Japanese patients with fanconi-bickel syndrome. J Hum Genet (2000) 45(1):60–2. doi: 10.1007/s100380050013
47. Lorenz-Depiereux B, Benet-Pages A, Eckstein G, Tenenbaum-Rakover Y, Wagenstaller J, Tiosano D, et al. Hereditary hypophosphatemic rickets with hypercalciuria is caused by mutations in the sodium-phosphate cotransporter gene SLC34A3. Am J Hum Genet (2006) 78:9. doi: 10.1086/499410
48. Ichida K, Amaya Y, Kamatani N, Nishino T, Hosoya T, Sakai O. Identification of two mutations in human xanthine dehydrogenase gene responsible for classical type I xanthinuria. J Clin Invest (1997) 99(10):2391–7. doi: 10.1172/JCI119421
49. Hamilton AJ, Bingham C, McDonald TJ, Cook PR, Caswell RC, Weedon MN, et al. The HNF4A R76W mutation causes atypical dominant fanconi syndrome in addition to a β cell phenotype. J Med Genet (2014) 51(3):165–9. doi: 10.1136/jmedgenet-2013-102066
50. Karim Z, Bénédicte G, Naziha B, Rohia A, Christine L, Laurent B, et al. NHERF1 mutations and responsiveness of renal parathyroid hormone. n Engl J Med (2008) 8:1128–35. doi: 10.1056/NEJMoa0802836
51. Pearce SHS, Williamson C, Kifor O, Bai M, Coulthard MG, Davies M, et al. A familial syndrome of hypocalcemia with hypercalciuria due to mutations in the calcium-sensing receptor. N Engl J Med (1996) 335(15):1115–22. doi: 10.1056/NEJM199610103351505
52. Enomoto A, Kimura H, Chairoungdua A, Shigeta Y, Jutabha P, Ho Cha S, et al. Molecular identification of a renal urate–anion exchanger that regulates blood urate levels. Nature (2002) 417(6887):447–52. doi: 10.1038/nature742
53. Matsuo H, Chiba T, Nagamori S, Nakayama A, Domoto H, Phetdee K, et al. Mutations in glucose transporter 9 gene SLC2A9 cause renal hypouricemia. Am J Hum Genet (2008) 83(6):744–51. doi: 10.1016/j.ajhg.2008.11.001
54. Prié D, Huart V, Bakouh N, Planelles G, Dellis O, Gérard B, et al. Nephrolithiasis and osteoporosis associated with hypophosphatemia caused by mutations in the type 2a sodium–phosphate cotransporter. N Engl J Med (2002) 347(13):983–91. doi: 10.1056/NEJMoa020028
55. Calonge MJ, Gasparini P, Chillarón J, Chillón M, Gallucci M, Rousaud F, et al. Cystinuria caused by mutations in rBAT, a gene involved in the transport of cystine. Nat Genet (1994) 6(4):420–5. doi: 10.1038/ng0494-420
56. Bruce LJ, Cope DL, Jones GK, Schofield AE, Burley M, Povey S, et al. Familial distal renal tubular acidosis is associated with mutations in the red cell anion exchanger (Band 3, AE1) gene. J Clin Invest (1997) 100(7):1693–707. doi: 10.1172/JCI119694
57. Feliubadaló L, Font M, Purroy J, Rousaud F, Estivill X, Nunes V, et al. Non-type I cystinuria caused by mutations in SLC7A9, encoding a subunit (bo,+AT) of rBAT. Nat Genet (1999) 23(1):52–7. doi: 10.1038/12652
58. Scott P, Ouimet D, Valiquette L, Guay G, Proulx Y, Trouve ML, et al. Suggestive evidence for a susceptibility gene near the vitamin d receptor locus in idiopathic calcium stone formation. JASN (1999) 10(5):1007–13. doi: 10.1681/ASN.V1051007
59. Lloyd SE, Pearce SHS, Fisher SE, Steinmeyer K, Schwappach B, Scheinman SJ, et al. A common molecular basis for three inherited kidney stone diseases. Nature (1996) 379(6564):445–9. doi: 10.1038/379445a0
60. Davidson BL, Tarle SA, Watts RWE. Identification of 17 independent mutations responsible for human hypoxanthine-guanine phosphoribosyltransferase (HPRT) deficiency. Am J Hum Genet (1991) 48:951–8.
61. Reilly DS, Lewis RA, Ledbetter DH, Nussbaum RL. Tightly linked flanking markers for the Lowe oculocerebrorenal syndrome, with application to carrier assessment. Am J Hum Genet (1988) 42(5):748–55.
62. Zikánová M, Wahezi D, Hay A, Stibůrková B, Pitts C, Mušálková D, et al. Clinical manifestations and molecular aspects of phosphoribosylpyrophosphate synthetase superactivity in females. Rheumatology (2018) 57(7):1180–5. doi: 10.1093/rheumatology/key041
63. Wang C, Han Y, Zhou J, Zheng B, Zhou W, Bao H, et al. Splicing characterization of CLCNKB variants in four patients with type III bartter syndrome. Front Genet (2020) 11:81. doi: 10.3389/fgene.2020.00081
64. Jabalameli MR, Fitzpatrick FM, Colombo R, Howles SA, Leggatt G, Walker V, et al. Exome sequencing identifies a disease variant of the mitochondrial ATP-Mg/Pi carrier SLC25A25 in two families with kidney stones. Mol Genet Genomic Med (2021) 9(12):e1749. doi: 10.1002/mgg3.1749
65. Yucheng G, Ruichao Z, Lei W, Chen N, Yuan D, Jun L, et al. Characteristics of genotype of monogenic nephrolithiasis in Chinese pediatric patients with nephrolithiasis. Natl Med J China (2021) 101(38):3115–20. doi: 10.3760/cma.j.cn112137-20210210-00404
66. Ang AJS, Sharma AA, Sharma A. Nephrolithiasis: Approach to diagnosis and management. Indian J Pediatr (2020) 87(9):716–25. doi: 10.1007/s12098-020-03424-7
67. Reusz GS, Hosszu A, Kis E. Evaluation of a child with suspected nephrolithiasis. Curr Opin Pediatr (2020) 32(2):265–72. doi: 10.1097/MOP.0000000000000880
68. Marra G, Taroni F, Berrettini A, Montanari E, Manzoni G, Montini G. Pediatric nephrolithiasis: a systematic approach from diagnosis to treatment. J Nephrol (2019) 32(2):199–210. doi: 10.1007/s40620-018-0487-1
69. Saadeh SA. Pediatric nephrolithiasis: Risk factors, evaluation, and prevention. Pediatr Ann (2020) 49(6):e262–7. doi: 10.3928/19382359-20200518-01
70. Bhojani N, Bjazevic J, Wallace B, Lee L, Kaler KS, Dion M, et al. Update – 2022 Canadian urological association guideline: Evaluation and medical management of the kidney stone patient. CUAJ (2022) 16(6):175–88. doi: 10.5489/cuaj.7872
71. Edvardsson VO, Goldfarb DS, Lieske JC, Beara-Lasic L, Anglani F, Milliner DS, et al. Hereditary causes of kidney stones and chronic kidney disease. Pediatr Nephrol (2013) 20:1923–42. doi: 10.1007/s00467-012-2329-z
72. Halbritter J, Baum M, Hynes AM, Rice SJ, Thwaites DT, Gucev ZS, et al. Fourteen monogenic genes account for 15% of Nephrolithiasis/Nephrocalcinosis. JASN (2015) 26(3):543–51. doi: 10.1681/ASN.2014040388
73. Lee IH, Lin Y, Alvarez WJ, Hernandez-Ferrer C, Mandl KD, Kong SW. WEScover: selection between clinical whole exome sequencing and gene panel testing. BMC Bioinf (2021) 22:259. doi: 10.1186/s12859-021-04178-5
74. Connaughton DM, Kennedy C, Shril S, Mann N, Murray SL, Williams PA, et al. Monogenic causes of chronic kidney disease in adults. Kidney Int (2019) 95(4):914–28. doi: 10.1016/j.kint.2018.10.031
75. Schwarze K, Buchanan J, Taylor JC, Wordsworth S. Are whole-exome and whole-genome sequencing approaches cost-effective? a systematic review of the literature. Genet Med (2018) 20(10):1122–30. doi: 10.1038/gim.2017.247
76. McCabe MJ, Gauthier MEA, Chan CL, Thompson TJ, De Sousa SMC, Puttick C, et al. Development and validation of a targeted gene sequencing panel for application to disparate cancers. Sci Rep (2019) 9(1):17052. doi: 10.1038/s41598-019-52000-3
77. Chu DI, Tasian GE, Copelovitch L. Pediatric kidney stones–avoidance and treatment. Curr Treat Options Peds (2016) 2(2):104–11. doi: 10.1007/s40746-016-0046-8
78. Van Batavia JP, Tasian GE. Clinical effectiveness in the diagnosis and acute management of pediatric nephrolithiasis. Int J Surg (2016) 36:698–704. doi: 10.1016/j.ijsu.2016.11.030
79. Mokhless I, Zahran AR, Youssif M, Fahmy A. Tamsulosin for the management of distal ureteral stones in children: A prospective randomized study. J Pediatr Urol (2012) 8(5):544–8. doi: 10.1016/j.jpurol.2011.09.008
80. Erturhan S, Bayrak O, Sarica K, Seckiner I, Baturu M, Sen H. Efficacy of medical expulsive treatment with doxazosin in pediatric patients. Urology (2013) 81(3):640–3. doi: 10.1016/j.urology.2012.11.031
81. Routh JC, Graham DA, Nelson CP. Trends in imaging and surgical management of pediatric urolithiasis at American pediatric hospitals. J Urol (2010) 184(4S):1816–22. doi: 10.1016/j.juro.2010.03.117
82. Liu X. SLC family transporters. In: Liu X, Pan G, editors. Drug transporters in drug disposition, effects and toxicity. Singapore: Springer (2019). p. 101–202. 2022 Sep 8Advances in Experimental Medicine and Biology.
83. Hopp K, Cogal AG, Bergstralh EJ, Seide BM, Olson JB, Meek AM, et al. Phenotype-genotype correlations and estimated carrier frequencies of primary hyperoxaluria. J Am Soc Nephrol (2015) 26(10):2559–70. doi: 10.1681/ASN.2014070698
84. Fuller ZL, Berg JJ, Mostafavi H, Sella G, Przeworski M. Measuring intolerance to mutation in human genetics. Nat Genet (2019) 51(5):772–6. doi: 10.1038/s41588-019-0383-1
85. Connaughton DM, Hildebrandt F. Personalized medicine in chronic kidney disease by detection of monogenic mutations. Nephrol Dialysis Transplantation (2020) 35(3):390–7. doi: 10.1093/ndt/gfz028
86. Devuyst O, Thakker RV. Dent’s disease. Orphanet J Rare Dis (2010) 5(1):28. doi: 10.1186/1750-1172-5-28
87. Pontecorvo G. Allelism. Cold Spring Harb Symp Quant Biol (1956) 21:171–4. doi: 10.1101/SQB.1956.021.01.014
88. Jin YY, Huang LM, Quan XF, Mao JH. Dent disease: classification, heterogeneity and diagnosis. World J Pediatr (2021) 17(1):52–7. doi: 10.1007/s12519-020-00357-1
89. Dent CE, Friedman M. Hypercalcuric rickets associated with renal tubular damage. Arch Dis Childhood (1964) 39(205):240–9. doi: 10.1136/adc.39.205.240
90. Wrong OM, Norden AG, Feest TG. Dent’s disease; a familial proximal renal tubular syndrome with low-molecular-weight proteinuria, hypercalciuria, nephrocalcinosis, metabolic bone disease, progressive renal failure and a marked male predominance. QJM (1994) 87(8):473–93.
91. Thakker RV. Pathogenesis of dent’s disease and related syndromes of X-linked nephrolithiasis. Kidney Int (2000) 57(3):787–93. doi: 10.1046/j.1523-1755.2000.00916.x
92. Anglani F, Gianesello L, Beara-Lasic L, Lieske J. Dent disease: A window into calcium and phosphate transport. J Cell Mol Med (2019) 23(11):7132–42. doi: 10.1111/jcmm.14590
93. Anglani F, D’Angelo A, Bertizzolo LM, Tosetto E, Ceol M, Cremasco D, et al. Nephrolithiasis, kidney failure and bone disorders in dent disease patients with and without CLCN5 mutations. SpringerPlus (2015) 4(1):492. doi: 10.1186/s40064-015-1294-y
94. Erdmann KS, Mao Y, McCrea HJ, Zoncu R, Lee S, Paradise S, et al. A role of the Lowe syndrome protein OCRL in early steps of the endocytic pathway. Dev Cell (2007) 13(3):377–90. doi: 10.1016/j.devcel.2007.08.004
95. Wu G, Zhang W, Na T, Jing H, Wu H, Peng JB. Suppression of intestinal calcium entry channel TRPV6 by OCRL, a lipid phosphatase associated with Lowe syndrome and dent disease. Am J Physiology-Cell Physiol (2012) 302(10):C1479–91. doi: 10.1152/ajpcell.00277.2011
97. Charnas LR, Bernardini I, Rader D, Hoeg JM, Gahl WA. Clinical and laboratory findings in the oculocerebrorenal syndrome of Lowe, with special reference to growth and renal function. N Engl J Med (1991) 324(19):1318–25. doi: 10.1056/NEJM199105093241904
98. Bockenhauer D, Bokenkamp A, van’t Hoff W, Levtchenko E, Kist-van Holthe JE, Tasic V, et al. Renal phenotype in Lowe syndrome: A selective proximal tubular dysfunction. CJASN (2008) 3(5):1430–6. doi: 10.2215/CJN.00520108
99. Bökenkamp A, Ludwig M. The oculocerebrorenal syndrome of Lowe: an update. Pediatr Nephrol (2016) 31(12):2201–12. doi: 10.1007/s00467-016-3343-3
100. Lewis RA, Nussbaum RL, Brewer ED. Lowe Syndrome. In: GeneReviews®. Seattle: University of Washington (2019). Available at: https://www-ncbi-nlm-nih-gov.proxy1.lib.uwo.ca/books/NBK1480/. 2022 Sep 8.
101. Claverie-Martin F. Familial hypomagnesaemia with hypercalciuria and nephrocalcinosis: clinical and molecular characteristics. Clin Kidney J (2015) 8(6):656–64. doi: 10.1093/ckj/sfv081
102. Müller D, Kausalya PJ, Meij IC, Hunziker W. Familial hypomagnesemia with hypercalciuria and nephrocalcinosis: blocking endocytosis restores surface expression of a novel claudin-16 mutant that lacks the entire c-terminal cytosolic tail. Hum Mol Genet (2006) 15(7):1049–58. doi: 10.1093/hmg/ddl020
103. Vianna JGP, Simor TG, Senna P, Bortoli MRD, Costalonga EF, Seguro AC, et al. Atypical presentation of familial hypomagnesemia with hypercalciuria and nephrocalcinosis in a patient with a new claudin-16 gene mutation. CNCS (2019) 7(01):27–34. doi: 10.5414/CNCS109595
104. Kausalya PJ, Amasheh S, Günzel D, Wurps H, Müller D, Fromm M, et al. Disease-associated mutations affect intracellular traffic and paracellular Mg2+ transport function of claudin-16. J Clin Invest (2006) 116(4):878–91. doi: 10.1172/JCI26323
105. Konrad M, Hou J, Weber S, Dötsch J, Kari JA, Seeman T, et al. CLDN16 genotype predicts renal decline in familial hypomagnesemia with hypercalciuria and nephrocalcinosis. J Am Soc Nephrol (2008) 19(1):171–81. doi: 10.1681/ASN.2007060709
106. Vall-Palomar M. Heterogeneity is a common ground in familial hypomagnesemia with hypercalciuria and nephrocalcinosis caused by CLDN19 gene mutations. J Nephrol (2021) 10:2053–62. doi: 10.1007/s40620-021-01054-6
107. Faller N. Nephrolithiasis secondary to inherited defects in the thick ascending loop of henle and connecting tubules. Urolithiasis (2019) 47:43–56. doi: 10.1007/s00240-018-1097-z
108. Elshamaa MF, Fadel FI, Kamel S, Farouk H, Alahmady M, Ramadan Y. Genetic polymorphisms in CLDN14 (rs219780) and ALP (rs1256328) genes are associated with risk of nephrolithiasis in Egyptian children. Turkish J Urol (2021) 47(1):73–80. doi: 10.5152/tud.2020.20141
109. Plain A, Alexander RT. Claudins and nephrolithiasis: Current opinion in nephrology and hypertension. Curr Opin Nephrol Hypertens (2018) 27(4):268–76. doi: 10.1097/MNH.0000000000000426
110. Soares SBM, de Menezes Silva LAW, de Carvalho Mrad FC, Simões e Silva AC. Distal renal tubular acidosis: genetic causes and management. World J Pediatr (2019) 15(5):422–31. doi: 10.1007/s12519-019-00260-4
111. Magni G, Unwin RJ, Moochhala SH. Renal tubular acidosis (RTA) and kidney stones: Diagnosis and management. Arch Esp Urol (2021) 74(1):123–8. doi: 10.1016/j.juro.2010.03.117
112. Mohebbi N. Pathophysiology, diagnosis and treatment of inherited distal renal tubular acidosis. J Nephrol (2018) 12:511–22. doi: 10.1007/s40620-017-0447-1
113. More TA, Kedar PS. Genotypic analysis of SLC4A1 A858D mutation in Indian population associated with distal renal tubular acidosis (dRTA) coupled with hemolytic anemia. Gene (2021) 769:145241. doi: 10.1016/j.gene.2020.145241
114. Alexander RT, Law L, Gil-Peña H, Greenbaum A, Santos F. Hereditary distal renal tubular acidosis. In: Adam MP, Everman DB, Mirzaa GM, Pagon RA, Wallace SE, Bean LJH, et al editors. GeneReviews® [Internet] Seattle (WA): University of Washington, Seattle (2019), 1993–2022.
115. Weger M, Deutschmann H, Weger W, Kotanko P, Skrabal F. Incomplete renal tubular acidosis in ‘Primary’ osteoporosis. Osteoporos Int (1999) 10:325–9.
116. Kogawa M, Wijenayaka AR, Ormsby RT, Thomas GP, Anderson PH, Bonewald LF, et al. Sclerostin regulates release of bone mineral by osteocytes by induction of carbonic anhydrase 2. J Bone Mineral Res (2013) 28:2433–5. doi: 10.1002/jbmr.2003
117. Furqan S, Banu S, Ram N. Osteoporosis complicating renal tubular acidosis in association with sjogren’s syndrome. Cureus (2021) 4:e18373. doi: 10.7759/cureus.18373
118. Marrani E, Giani T, Simonini G, Cimaz R. Pediatric osteoporosis: Diagnosis and treatment considerations. Drugs (2017) 77(6):679–95. doi: 10.1007/s40265-017-0715-3
119. Filler G. Growth hormone therapy in HHRH. Bone Rep (2022) 6:101591. doi: 10.1016/j.bonr.2022.101591
120. Dasgupta D, Wee MJ, Reyes M, Li Y, Simm PJ, Sharma A, et al. Mutations in SLC34A3/NPT2c are associated with kidney stones and nephrocalcinosis. J Am Soc Nephrol (2014) 25(10):2366–75. doi: 10.1681/ASN.2013101085
121. Bergwitz C. Hereditary hypophosphatemic rickets with hypercalciuria: pathophysiology, clinical presentation, diagnosis and therapy. Pflugers Arch (2019) 471:149–63. doi: 10.1007/s00424-018-2184-2
122. Stechman MJ, Loh NY, Thakker RV. Genetic causes of hypercalciuric nephrolithiasis. Pediatr Nephrol (2009) 24(12):2321–32. doi: 10.1007/s00467-008-0807-0
123. Akbari A, Pipitone GB, Anvar Z, Jaafarinia M, Ferrari M, Carrera P, et al. ADCY10 frameshift variant leading to severe recessive asthenozoospermia and segregating with absorptive hypercalciuria. Human Reproduction (2019) 24(6):1155–64. doi: 10.1093/humrep/dez048
124. Lau KK, Butani L. Treatment strategies for pediatric idiopathic hypercalciuria. Front Biosci (Elite Ed) (2009) 1(1):299–305. doi: 10.2741/S29
125. Bushinsky DA, Frick KK, Nehrke K. Genetic hypercalciuric stone-forming rats. Curr Opin Nephrol Hypertens (2006) 15:403–18. doi: 10.1097/01.mnh.0000232881.35469.a9
126. Minisola S, Pepe J, Piemonte S, Cipriani C. The diagnosis and management of hypercalcaemia. BMJ (2015) 350(jun02 15):h2723–3. doi: 10.1136/bmj.h2723
127. Lee JY, Shoback DM. Familial hypocalciuric hypercalcemia and related disorders. Best Pract Res Clin Endocrinol Metab (2018) 32(5):609–19. doi: 10.1016/j.beem.2018.05.004
128. Schlingmann KP, Ruminska J, Kaufmann M, Dursun I, Patti M, Kranz B, et al. Autosomal-recessive mutations in SLC34A1 encoding sodium-phosphate cotransporter 2A cause idiopathic infantile hypercalcemia. J Am Soc Nephrol (2016) 27(2):604–14. doi: 10.1681/ASN.2014101025
129. Hebert SC. Bartter syndrome. Curr Opin Nephrol Hypertension (2003) 12(5):527–32. doi: 10.1097/00041552-200309000-00008
130. Simon DB, Karet FE, Rodriguez-Soriano J, Hamdan JH, DiPietro A, Trachtman SH, et al. Genetic heterogeneity of bartter’s syndrome revealed by mutations in the k+ channel, ROMK. Nature (1996) 14:5.
131. Yi S, Li M, Yang Q, Zhang X, Chen F, Qin Z, et al. Novel SLC12A1 mutations cause bartter syndrome in two patients with different prognoses. Clinica Chimica Acta (2022) 531:120–5. doi: 10.1016/j.cca.2022.03.025
132. Elfert KA, Geller DS, Nelson-Williams C, Lifton RP, Al-Malki H, Nauman A. Late-onset bartter syndrome type II due to a homozygous mutation in KCNJ1 gene: A case report and literature review. Am J Case Rep (2020) 21:e924527. doi: 10.12659/AJCR.924527
133. Sharma A, Linshaw MA. A novel compound heterozygous ROMK mutation presenting as late onset bartter syndrome associated with nephrocalcinosis and elevated 1,25(OH)2 vitamin d levels. Clin Exp Nephrol (2011) 15(4):572–6. doi: 10.1007/s10157-011-0431-3
134. Zuo J, Guo W, Wang S, Lang Y, Wang S, Shi X, et al. Eight novel KCNJ1 variants and parathyroid hormone overaction or resistance in 5 probands with bartter syndrome type 2. Clinica Chimica Acta (2020) 511:248–54. doi: 10.1016/j.cca.2020.10.002
135. Cheng CJ, Lo YF, Chen JC, Huang CL, Lin SH. Functional severity of CLCNKB mutations correlates with phenotypes in patients with classic bartter’s syndrome: Genotype-phenotype analysis of CLCNKB mutations. J Physiol (2017) 595(16):5573–86. doi: 10.1113/JP274344
136. Riazuddin S, Anwar S, Fischer M, Ahmed ZM, Khan SY, Janssen AGH, et al. Molecular basis of DFNB73: Mutations of BSND can cause nonsyndromic deafness or bartter syndrome. Am J Hum Genet (2009) 85(2):273–80. doi: 10.1016/j.ajhg.2009.07.003
137. Kömhoff M, Laghmani K. MAGED2: a novel form of antenatal bartter’s syndrome. Curr Opin Nephrol Hypertension (2018) 27(4):323–8. doi: 10.1097/MNH.0000000000000422
138. Humphrey E, Clardy C. A framework for approaching refractory hypocalcemia in children. Pediatr Ann (2019) 48(5):e208–11. doi: 10.3928/19382359-20190423-01
139. Pepe J, Colangelo L, Biamonte F, Sonato C, Danese VC, Cecchetti V, et al. Diagnosis and management of hypocalcemia. Endocrine (2020) 69(3):485–95. doi: 10.1007/s12020-020-02324-2
140. Vahe C, Benomar K, Espiard S, Coppin L, Jannin A, Odou MF, et al. Diseases associated with calcium-sensing receptor. Orphanet J Rare Dis (2017) 12(1):19. doi: 10.1186/s13023-017-0570-z
141. Roszko KL, Stapleton Smith LM, Sridhar AV, Roberts MS, Hartley IR, Gafni RI, et al. Autosomal dominant hypocalcemia type 1: A systematic review. J Bone Mineral Res (2022), 1–10. doi: 10.1002/jbmr.4659
142. Hannan FM, Kallay E, Chang W, Brandi ML, Thakker RV. The calcium-sensing receptor in physiology and in calcitropic and noncalcitropic diseases. Nat Rev Endocrinol (2019) 15(1):33–51. doi: 10.1038/s41574-018-0115-0
Keywords: nephrolithiasis, kidney stones, monogenic, pediatric, genetic, kidney disease
Citation: Schott C, Pourtousi A and Connaughton DM (2022) Monogenic causation of pediatric nephrolithiasis. Front. Urol. 2:1075711. doi: 10.3389/fruro.2022.1075711
Received: 20 October 2022; Accepted: 15 November 2022;
Published: 27 December 2022.
Edited by:
Elke E. Mau, University of Saskatchewan, CanadaCopyright © 2022 Schott, Pourtousi and Connaughton. This is an open-access article distributed under the terms of the Creative Commons Attribution License (CC BY). The use, distribution or reproduction in other forums is permitted, provided the original author(s) and the copyright owner(s) are credited and that the original publication in this journal is cited, in accordance with accepted academic practice. No use, distribution or reproduction is permitted which does not comply with these terms.
*Correspondence: Dervla M. Connaughton, ZGVydmxhLmNvbm5hdWdodG9uQGxoc2Mub24uY2E=; Clara Schott, Y3NjaG90dDJAdXdvLmNh