- 1Department of Medicine D, University of Münster, Münster, Germany
- 2Department of Internal Medicine and Nephrology, University Hospital of Münster Marienhospital Steinfurt, Steinfurt, Germany
The calcineurin inhibitor (CNI) Tacrolimus (Tac) is the most prescribed immunosuppressant drug after solid organ transplantation. After renal transplantation (RTx) approximately 95% of recipients are discharged with a Tac-based immunosuppressive regime. Despite the high immunosuppressive efficacy, its adverse effects, narrow therapeutic window and high intra- and interpatient variability (IPV) in pharmacokinetics require therapeutic drug monitoring (TDM), which makes treatment with Tac a major challenge for physicians. The C/D ratio (full blood trough level normalized by daily dose) is able to classify patients receiving Tac into two major metabolism groups, which were significantly associated with the clinical outcomes of patients after renal or liver transplantation. Therefore, the C/D ratio is a simple but effective tool to identify patients at risk of an unfavorable outcome. This review highlights the challenges of Tac-based immunosuppressive therapy faced by transplant physicians in their daily routine, the underlying causes and pharmacokinetics (including genetics, interactions, and differences between available Tac formulations), and the latest data on potential solutions to optimize treatment of high-risk patients.
1. Introduction
Currently, the calcineurin inhibitor (CNI) Tacrolimus (Tac) is the most prescribed immunosuppressant after renal transplantation (RTx), as approximately 95% of recipients are discharged with a Tac-based immunosuppressive regimen (1). It is recommended by the The Kidney Disease: Improving Global Outcomes (KDIGO) guideline as the main cornerstone immunosuppressant after RTx (2) because it offers a significant reduction in acute rejection rates as well as an increase in long-term allograft survival (3). Despite being highly effective, its numerous adverse effects such as nephrotoxicity, infection rates, post-transplant diabetes, neurotoxicity, hypertension, and malignancies are limiting its usage (4). Therefore, many efforts have been made to develop alternative immunosuppressive regimens or improve drug galenic, especially for patients suffering from adverse effects or at risk of unfavorable outcome. Multiple studies on dose reduction by combining Tac with mechanistic target of rapamycin (mTor) inhibitors or belatacept, as well as elimination by switching to other immunosuppressant drugs have been published lately (5, 6). Therein, everolimus-based immunosuppression showed comparable efficacy in combination with (reduced) Tac compared with a standard regimen in renal and liver transplant patients, with comparable increases in renal function and treatment failure rates, but lower cytomegalo- and BK polyomavirus infection rates (7–9), most likely by retaining CMV-specific T-cell functionality (10), leaving both protocols safe and effective (11).
Besides the adverse effects, the high inter- and intraindividual variability (IPV) in pharmacokinetics (PK) and -dynamics pose a major challenge to physicians at reaching a given target trough level within the narrow therapeutic window. While therapeutic drug monitoring (TDM) is essential to prevent underexposure associated with increased rejection risk, and overexposure, which leads to an increase in adverse events, it has limitations in predicting the required Tac dose, due to the many factors that impact the Tac metabolism (12). These include external influencing factors such as drugs, foods, and herbs along with internal aspects like gastrointestinal factors as well as sex, age, serum albumin, hematocrit, and genetic variants, i.e., in the cytochrome P450 (CYP3A4/5) expression. Beside of that, the individual response of patients to Tac treatment and the different galenics of the available Tac formulations lead to distinct pharmacokinetic profiles, which can result in over- or underexposure, although the measured trough concentrations are always within the therapeutic window (13).
Despite the aforementioned potentially toxic profile and limitations of TDM, Tac remains the most relevant immunosuppressant in therapy of patients after solid organ transplantation, which has led to a broad variety of studies addressing Tac metabolism and optimization of dosing. Even with machine-learning based approaches, statistic modelling, and genetic analyses, which have been proven to be effective in dose calculation (14–16), a simple and cost-effective tool for clinical practice is not yet available.
With this review, we want to offer an update on the current knowledge of Tac pharmacokinetics and provide assistance to physicians in clinical practice by presenting a simple and cost-effective tool, the C/D ratio, which can support the management of Tac-based immunosuppressive therapy.
2. Factors influencing Tac pharmacokinetics
2.1. CYP3A4, CYP3A5 and P-glycoprotein
One of the most important determinants of Tac PK is the first-pass effect, regulated by Cytochrome P450 enzymes CYP3A4 and CYP3A5 as well as the P-glycoprotein (P-gp), which are predominantly expressed in the liver and gut, but can also been found in other tissues. Their function depends on genetic polymorphisms (12). It is important to note that CYP3A4/5 and P-gp are involved in the metabolization process of a wide variety of drugs and susceptible to induction or inhibition (17, 18).
P-gp is an ATP-dependent efflux pump located in the cell membrane that, among other drugs, transports Tac back into the intestinal lumen. CYP3A4 and CYP3A5 are monooxygenases and involved in the so-called phase I metabolism by catalyzing many reactions of multiple different drugs (19, 20). Phase II metabolism on the other hand occurs exclusively in the liver by glucuronidation, conjugation, acetylation, sulfation, and demethylation. After phase I and phase II, Tac is metabolized into at least 8 different products (21), potentially involved in mechanisms of Tac toxicity (22). Some metabolites are described in literature, such as M-I, M-II and M-III which are mono-demethylated, M-V/VI/VII which are di-demethylated, the mono-hydroxylated M-IV and M-VIII, which is modified by multiple reactions (23, 24). Despite being known for some years, data on this subject are inconsistent, and the pharmacological activity of Tac metabolites requires further investigation. Overall, Tac is largely metabolized by the liver and excreted via the bile (∼97%) and urine (2%), with less than 1% of the compound remaining unmetabolized in urine and feces (25).
2.2. Gastrointestinal parameters
After oral Tac intake, it undergoes presystemic metabolism, which mainly involves CYP3A4 and CYP3A5 metabolism in gut and liver as well as P-gp transport into the intestinal lumen (26, 27). When Tac enters an enterocyte of the intestinal mucosa as a highly lipophilic substance, it either migrates to the basolateral side and into the blood or is eliminated by CYP3A enzymes and P-gp. The latter keeps the intracellular Tac concentration low and therefore prevents CYP3A from saturation (28–30). Elimination of Tac metabolites occurs primarily through biliary excretion (more than 95%), while only 0.5% of the parent drug could be found unchanged in the urine or feces (30). On top of that, Guo et al. recently found an additional elimination route in which commensal gut bacteria (mainly Clostridiales) convert Tac into the 15-fold less potent metabolite M-I (31).
Because of several gastrointestinal parameters, absorption and bioavailability of Tac (when administered orally) are highly variable (32), leading to the high IPV mentioned earlier. First of all, gastric pH and motility impact Tac absorption directly (33). The latter could be an explanation for the circadian and time-dependent changes in Tac PK, as studies showed a significantly lower Tac peak level (Cmax) and area under the curve (AUC) after nighttime administration than after morning administration, while it is known that the gastric emptying rate physiologically decreases in the evening time (13, 34, 35). This is supported by the fact that CYP3A expression decreases in the distant intestine and an influence of the CYP3A expressor status on Tac chronopharmacokinetics could not be found (36). In addition, the Tac level appears to be relatively constant during the day and night when using continuous i.v. application, as described in a small number of 10 patients (37). Interestingly, despite the lower measured AUC after nocturnal dosing, a recent study showed no significant reduction in pharmacodynamic effect (38).
Tac bioavailability is generally influenced by food intake. In particular, high-fat content food reduces Tac absorption in the gut, which is why it is recommended to eat 2 h before or 1 h after taking Tac (39, 40). In addition to that, several food components, dietary supplements and herbs can have an influence on Tac metabolization, i.e., an inhibition of CYP3A4 by grapefruit juice should be noticed (41, 42).
As mentioned before, the intestinal first-pass mechanism is highly responsible for the IPV after Tac exposure, with the hepatic metabolic component likely predominating (43). One explanation could be the different distribution of CYP3A and P-gp within the intestine, as CYP3A expression decreases more distally and P-gp expression, though having a very high variability, tends to increase and reaches its maximum in the colon (44–46). Analogous to the decrease of CYP3A expression, Tac absorption mainly occurs in the distal part of the intestine despite the higher P-gp concentration. This leads to higher Tac absorption rates with decreased intestinal transit time as the Tac concentration increases in the distal intestine (47, 48). Which again could be an explanation for the significant reduction of the necessary dose in patients receiving LCP-Tac, an extended-release formulation, in comparison to an immediate release Tac intake (13).
It is well known that patients suffering from diarrhea show increased Tac trough levels. This can be partially explained by the aforementioned mechanism as the main absorption area shifts from the proximal to the distal intestine. Additionally, besides increased hemoconcentration and decreased hepatic blood flow (which negatively influences Tac metabolism), intestinal damage and inflammation could lead to increased drug permeability and negatively affect CYP3A and P-gp expression, particularly in the lower intestine (49, 50).
2.3. Genetic polymorphisms
As mentioned before, the high inter- and intrapatient variability of Tac PK poses a problem for clinical management, especially when defining the starting dose after transplantation as well as dose adjustment of ongoing therapy. While this can partially be attributed to different genetic variations of participating factors in Tac metabolism such as CYP3A enzymes and membrane drug transporters, pharmacogenetics emerges as a possible tool to assist physicians in clinical practice (51, 52).
Nowadays it is evident that fast metabolizers mainly express CYP3A5*1, while CYP3A5*3 expressors have been identified as slow metabolizers (53). CYP3A4 activity, however, showed no correlation to Tac parent/metabolite ratios, confirming CYP3A5 as the single most important determinant of Tac metabolism (54). Nevertheless, CYP3A5 status was not shown to have a decisive influence on IPV (55, 56). Therefore, it is not surprising that efforts trying to integrate CYP3A5 status into Tac dosing considerations were often disappointing regarding safety or outcome (57–59). This could be due to the fact that CYP3A5 is only a part of a broad variety of factors that influence Tac metabolism (51). Recent studies showed that approximately 16.3% of Tac's biovariability is explained with CYP3A5 expression, 43.3% in combination with clinical factors such as red blood counts and albumin, and only 70% when they included a total of 44 gene variants (15, 60). The latter shows the complexity of this topic as high throughput genetic screening was necessary, which requires high effort in terms of cost and interpretation and is therefore not suited for daily clinical practice (15). At least an approach considering clinical and demographical factors (with age and body surface area being the most effective ones) next to CYP3A genotyping showed promising results regarding individualizing Tac dose early after transplantation (14), but further studying on this topic is needed.
To date there is no general recommendation regarding pre-emptive genotyping, even though doubling the first Tac dose after solid organ transplantation is recommend in known CYP3A5 expressors. This leaves TDM as the main tool for managing Tac dose adjustment in clinical practice, as there are no further recommendations regarding pharmacogenetic biomarkers to date (51).
2.4. Blood and tissue distribution
After transfer into blood, lipophilic Tac is mainly bound to red blood cells through the FK506 binding proteins (FKBP), leading to a 4-114-fold higher Tac concentration in full blood compared to plasma (61, 62). Despite poor correlation with AUC, TDM by measuring full blood concentration 12 (C12)- or 24 (C24) hours after administration is most commonly used and currently state of the art (51). Other assessment points with better correlation to AUC (especially for once-daily formulations) have been published, i.e., C6 as the most accurate single point or in combination with C2 as a two-point model for AUC0-12 (63) and C2 plus C10 for AUC0-24 (64), but have not yet gained acceptance in clinical practice, mainly due to practical reasons (65). Analytical methods for Tac measurement include either different immunoassays or liquid chromatography coupled to tandem mass spectrometry (LC-MS/MS). LC-MS/MS is the current gold standard due to its high sensitivity and specificity and its ability to quantify multiple compounds simultaneously, but its usage is limited by the need for specialized trained staff and by high efforts and costs. This carries the risk of handling errors and, in addition to high assay heterogeneity, leads to high inter-laboratory variation, making global standardization challenging (66–68). As a result, almost half of laboratories worldwide primarily use immunoassays that are produced as commercial kits (67). However, these are less accurate due to cross-reactivity of the immunoassay antibodies with Tac metabolites and are associated with higher result variability (66, 69). Therefore, current research is focused on the development of new fully automated LC-MS/MS instruments (70, 71).
Because Tac binding to red blood cells is temperature sensitive as well as nonlinear, and the uptake by red blood cells is strongly dependent on FKBP concentration, it is practically difficult to separate full blood from plasma Tac (72). Recently, Yoshikawa et al. also suggested FKBP as a potential biomarker for predicting Tac PK, because of its important role in the distribution of Tac in red blood cells (73). However, plasma Tac is predominantly bound to albumin, lipoproteins, α1-acid glycoprotein, and globulins, whereas the unbound fraction is mainly responsible for therapeutic effects (32). Only unbound Tac is cleared by the liver, but because it is only released slowly by red blood cells, hepatic clearance is comparatively low (74). Therefore, Tac is a low-clearance substance.
As expected, whole blood concentration of the clinically relevant unbound fraction is strongly dependent to hematocrit and albumin levels. Lower hematocrit and albumin levels mean less full blood concentration, since excess unbound Tac is quickly eliminated by the liver with no difference in therapeutic effect or toxicity. Therefore, Tac full blood concentration in patients with anemia or hypalbuminemia may be underestimated, which may result in higher toxicity after increasing the Tac dose to maintain target trough levels (12, 75–77). Sikma et al. recently confirmed this correlation in early-stage patients after thoracic organ transplantation and suggested measuring hematocrit-corrected whole blood levels or using i.v.-application, aiming for a lower therapeutic range (78, 79). However, it should be noted that i.v.-administration of tacrolimus should be dose-reduced and continuous over 24 h to avoid bolus-associated toxicity (25).
For its therapeutic effect, Tac binds to and forms a complex with FKBP12 in lymphocytes and inhibits the activity of calcineurin (73). Intracellular concentration of Tac in lymphocytes correlates with full blood concentration (albeit poorly) and is influenced by age, sex, albumin levels, hematocrit, transplant duration and P-gp expression while determining the degree of T-cell-activation. However, it was not found to correlate with genetic variation in CYP3A enzymes and clinical outcome such as rejection rates (80–82).
Tacrolimus is extensively distributed in the whole body but its distribution in solid organ tissue behaves similarly to lymphocytes and is dependent on P-gp expression and intracellular metabolism. Tac clearance is not affected by low-grade renal or liver dysfunction (83). Tac, however, impairs renal function directly in an acute and chronical manner through vasoconstriction and nephrotoxicity (84). Besides systemic Tac levels, recent studies also showed a significant involvement of intrarenal accumulation in CNI-induced nephrotoxicity. Tac accumulation in the allograft was shown to be associated with Tac dose, full blood concentration and, most notably, acute nephrotoxicity. An association with CYP3A5 or ABCB1 genetics could not be found (85, 86). In addition, we found that the development and extent of acute Tac-induced nephrotoxicity were related to peak drug concentration; higher peak levels were significantly more often associated with toxicity in histology than lower levels (87).
2.5. Drug-drug interactions
Besides Tac, cytochrome P450 enzymes CYP3A4/5 metabolize a broad spectrum of different drugs, which can also act as inducers or inhibitors and thus influence Tac metabolism (88–90). In addition, Tac competes with other drugs on binding capacities of P-gp. For example, various antibiotics (i.e., macrolide), calcium antagonists, protease-/kinase inhibitors [antivirals for HIV (e.g., booster), SARS-Cov2 (e.g., nirmatrelvir/ritonavir), or CMV (e.g., maribavir)], or antimycotics such as azoles can inhibit the activity of CYP3A enzymes (47, 91, 92). With regard to azoles, Huppertz et al. described only very small increases in Tac exposure when using LCP-Tac, a new once daily Tac formulation, after CYP3A inhibition with voriconazole, resulting in comparatively little effect on AUC and biovariability, in contrast to immediate-release Tac (IR-Tac), and elegantly demonstrating the influence of drug galenics and CYP3A5 distribution in the gut (93).
Inducers of CYP3A enzymes relevant to transplant physicians medicine are isoniazid, rifampicin, several anticonvulsive drugs and most importantly St. John's wort, a long time used herb for the treatment of depression, which is still commonly used (94). Because it depends on the regulation of certain nuclear receptors, induction of CYP3A and P-gp occurs with a delay (47). Importantly, steroids, which are part of many immunosuppressive regimes after solid organ transplantation, also induce Tac metabolism and increase Tac clearance (95, 96).
2.6. Age
The age dependence of Tac metabolism has been the subject of several studies. Pediatric patients require weight-adjusted and usually higher Tac doses compared to adults to reach a certain trough level (97, 98). This effect was attributed to increased hepatic clearance because liver size is relatively larger in children normalized to body weight, whereas no age-related difference was seen with a Tac dose normalized to body surface area (99).
As for elderly patients, things become more difficult as physicians are faced with more comorbidities and a higher potential of drug-drug interactions (polypharmacy) as well as a different immune response (immunosenescence) and susceptibility to adverse effects (99). With age, the immune system weakens, which leads to more infectious complications but also lower rejection rates. Consequently, it was shown in vitro and in a mice model, that a compromised CD4+ T cell response is related to augmented immunosuppressive capacities of Tac in elderly patients (100). This leads to the suggestion to aim at lower target trough level. In addition, it has been suggested that renal susceptibility to Tac increases with kidney age (84), and since older recipients tend to receive older grafts than younger ones, this must be taken into account. On the other hand, older kidneys are more immunogenic than younger ones (101).
Regarding Tac PK, studies showed contradictory results regarding an age dependency. Unfortunately, the number of elderly patients in these studies was mostly small, so their power with respect to this question is limited (102–104). In theory, an age-dependence of Tac PK would be logical, as a reduced splanchnic and hepatic blood flow, higher body fat, and changes in gastric pH in the elderly should have an influence on the distribution and clearance of Tac. This is supported by data from Jacobson et al., describing higher Tac trough levels with increasing age (105) and our data, since we found a higher rate of slow metabolizers in elderly than in younger patients (106). In addition to that, David-Neto et al. showed a lower Tac clearance and need for higher doses in elderly patients up to 6 months after transplantation (107). The fact that the once daily Tac formulation (LCP-Tac) results in lower treatment failure rates than twice-daily capsules (IR-Tac) in patients with age >65 years also supports an impact of age on Tac PK (108). Beside of that, safety and efficacy studies in this patient group are still lacking and need to be further investigated in light of the increasing number of elderly renal transplant recipients.
2.7. Sex and pregnancy
According to several studies, female patients require higher Tac doses (83, 109, 110) and have a greater risk of experiencing adverse effects (111). In pregnant women, it is necessary to increase the Tac dose (about 25%–50% by the end of the first trimester) to maintain a target trough level even though the effective drug concentration is most likely overestimated (112). This is due to an increased Tac metabolism from a higher CYP3A4 activity as well as an increase in plasma volume next to hypoalbuminemia and anaemia (77). In a review from Chandra et al., the author suggests to stay in the target trough level, albeit risking over-immunosuppression, and only reduce Tac dose if nephrotoxicity is suspected (113). Congenital malformations have not been associated to Tac exposure, which is most likely because of an active P-gp efflux transport towards the mother even though Tac crosses the placenta. Although this prevents the foetus from higher Tac exposure, physicians should be aware that nephrotoxicity and hyperkalaemia can occur in the foetus as well. Although it is very unlikely that Tac excretion in the breast milk leads to adverse effects in the child, it is generally not recommended according to the SmPC (25, 77).
2.8. Ethnicity
Several studies have reported that African Americans require higher Tac doses compared to Caucasians or Asians (96, 114, 115) mainly due to differences in intestinal P-gp and CYP3A variants (105, 116). A recent observational study showed an association of CYP3A5*3 with higher doses in all ancestries, but CYP3A5*6 and CYP3A5*7 were present only in African Americans (117). The influence of CYPs in this context was confirmed by a study comparing the administration of LCP-Tac and IR-Tac in African Americans and showing that PK was significantly less affected in the case of LCP-Tac than IR-Tac (118). Native Americans usually require lower Tac doses due to a decreased oral Tac clearance (119).
2.9. Time after transplantation
After transplantation, high doses of steroids are initially administered, which induces CYP3A4 activity, requiring higher doses of Tac to reach target levels. Then, steroids are reduced over time, resulting in increased absorption of Tac and decreased CYP3A activity (120). Thus, required Tac doses decrease over time after transplantation (“maturation”), so TDM should be executed in a higher frequency (104, 121), especially in CYP3A5 expressors. In addition to that, haematocrit and albumin levels increasing as well, which amplifies the aforementioned effects (120).
2.10. Tac formulation
After its introduction as Prograf® by Astellas in Japan in 1993, several generics of IR-Tac were produced when the patent expired in 2008, the first being tacrolimus from Sandoz in 2009. Bioequivalence between the generic or branded drugs and the original Prograf® has been shown in at least 10 publications, recently reviewed by Kocur et al. (122), with the exception of the granular formulation Modigraf®, which showed a 23% and 18% higher mean for Cmax and AUC, respectively (123).
As a reaction to the high variability in intrapatient trough levels, which are associated with worse outcomes (124–129), once-daily administered Tac formulations with prolonged drug release have been developed to improve bioavailability and adherence as well as lower intraday fluctuation and peak concentrations (Cmax), as illustrated in Figure 1. In addition to the widely established IR-Tac, granulate formulation extended release (ER)-Tac (Advagraf®) with a prolonged drug release (90% absorption after 6–12 h) and LCP-Tac (Envarsus®) with an additional improved bioavailability have been established in clinical practice, reviewed in detail by Piotti et al. (130). The latest formulation, LCP-Tac, uses MeltDose® technique, which improves solubility by breaking the particles down into the smallest possible units. This results in a progressive release of the drug in the distal intestine, which has much lower CYP3A activity and therefore lower Tac clearance (131). A recent randomized controlled trial compared the three Tac formulations in stable kidney transplanted patients and focused on PK (13). It showed a lower intraday fluctuation, a prolonged time (Tmax) to peak concentration (Cmax) and a significantly higher exposure on a per milligram basis for LCP-Tac than for ER- and IR-Tac. After exposure normalization, Cmax was noticeably lower for LCP-Tac vs. IR-/ER-Tac (roughly 17%), while Cmin only differed slightly to ER- (6% lower) and IR-Tac (3% higher). In accordance with other studies in liver and kidney transplant recipients (132, 133), a dose reduction of ∼30% after converting from IR- and 36% from ER- to LCP-Tac has been suggested. These different dose requirements also result in lower therapy costs (131, 134). In the ASTCOFF trial, ER-Tac did not show any differences in terms of exposure, Cmax, Tmax or fluctuation vs. IR-Tac, which is not always consistent with previous studies reporting slightly lower Cmax, longer Tmax and in some studies even a lower PK variability of ER-Tac compared to IR-Tac (135–137). The dose conversion rate from IR- to ER-Tac has been calculated to +8% (13). This means that higher doses are required (138), possibly attributed to lower saturation of CYP3A and P-gp by a prolonged release in the intestine and therefore resulting in an increase of the metabolism rate (47). Following the findings mentioned above, the recommended starting dose of IR-Tac with 0.1–0.3 mg/kg/day remained similar with ER-Tac. Because of lower dose requirements for reaching effective trough levels in de novo renal transplant recipients of 30%, as shown in a randomized controlled trial, the starting dose for LCP-Tac was reduced to 0.17 mg/kg/day (139). Interestingly, LCP-Tac was not able to significantly reduce IPV compared with IR-Tac or ER-Tac (140). This might be related to the limited influence of CYP3A5 status on IPV mentioned earlier.
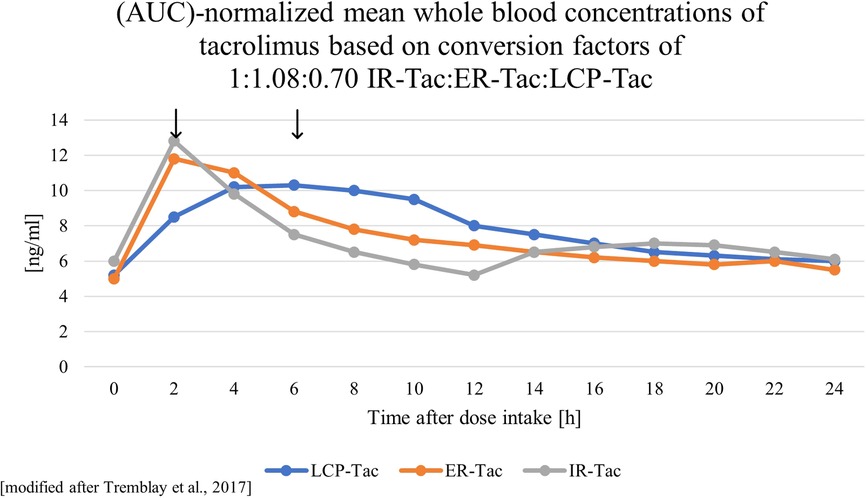
Figure 1. Schematic visualizations of the pharmacokinetics of different tacrolimus formulations. ER-Tac, extended-release tacrolimus, Advagraf®; IR-Tac, immediate-release tacrolimus, Prograf®; LCP-Tac, once-daily, Envarsus®, arrows mark the different peak concentrations.
However, despite differences in PK between the Tac formulations, prospective trials showing superiority of LCP-Tac in terms of patient outcome remain rare. Recently, trials demonstrated similar clinical outcomes of recipients treated with LCP-Tac or other tacrolimus formulations during the first months following de novo kidney transplantation (141, 142). However, a benefit for LCP-Tac has been demonstrated in patients at high risk of treatment failure (108) and with Tac-induced tremor (131, 143). Since the tremor and also the nephrotoxicity of Tac is related with a certain degree to Cmax, LCP-Tac (with lower Cmax) might be beneficial in susceptible patients, especially rapid metabolizers (87, 144). Using retrospective data, we observed that switching to LCP-Tac was associated with a noticeable recovery of renal function in fast metabolizers (145). In liver transplant recipients, improvement in renal function was observed 12 months after conversion from standard-release Tac (IR- or ER-Tac) to LCP-Tac (146). Because non-adherence is significantly associated with higher rates of antibody-mediated rejection and graft failure (147), one of the main aims in the development of once-daily Tac formulations was the improvement of drug adherence, which has been demonstrated by several studies (148–150).
3. Relevance of current Tac dose finding models and the role of the C/D ratio
The high inter- and intrapatient variability in Tac PK poses a problem to physicians in predicting Tac exposure in their patients. Given its association with impaired long-term allograft outcome, recent efforts have been made to develop models to optimize individual immunosuppressive therapy. As previously described, genetic testing strategies were related to high efforts and costs and often disappointing regarding safety or outcome (57–59, 151–153). Population PK models for Tac dose prediction have been established but are complicated and need further research, especially regarding newer Tac formulations (154, 155).
Despite the drawbacks such as high IPV and the narrow therapeutic window, Tac remains the most important immunosuppressant in patients after solid organ transplantation. To assist clinicians with a practical method for risk assessment rather than dose calculation, we previously proposed the calculation of the C/D ratio (106, 144). As presented in Figure 2 below, the C/D ratio is a simple and cost-effective tool that helps clinicians to optimize individual immunosuppressive therapy and identify patients at risk for adverse effects and unfavorable outcome.
The C/D ratio is calculated by dividing the Tac trough level, which is routinely measured, through the daily Tac dose at a specific time (usually later than 1 months after surgery) in the steady state after solid organ transplantation (106, 156). It should be noted that the C/D ratio can be calculated for every Tac formulation but cut-offs for definition of different metabolism groups are different. The performance of the C/D ratio has been extensively tested by our group and others in renal transplant patients. However, it should be noted that although there are many studies from different continents and transplant programmes with mostly consistent results, they are based on retrospective evaluations. A prospective study of liver transplant patients is currently recruiting in Germany, and several studies of kidney transplant patients are underway. Herein, we previously found a C/D ratio of 1.05 ng/ml × 1/mg to be a suitable cut off for distinguishing slow metabolizers (C/D ratio ≥1.05 ng/ml × 1/mg) from fast metabolizers (C/D ratio <1.05 ng/ml × 1/mg) when using IR-Tac. We demonstrated that fast metabolizers needed more indication biopsies, were more likely to experience biopsy proven CNI nephrotoxicity and BK virus infection, as well as to develop lower estimated glomerular filtration rate (eGFR) values during a 24-month follow-up after renal transplantation (106, 157). Following studies by our center showed that a low C/D ratio increases the risk of developing acute CNI-induced nephrotoxicity and was associated with a faster decline of eGFR, higher rejection rates and, most importantly, a reduced patient as well as overall graft survival within 5 years after RTx (87, 144). This was confirmed in 2020 by the TOMATO study, in which the authors described the C/D ratio as a predictor of death-censored graft survival (158). Interestingly, the French study included patients on IR-Tac and ER-Tac in their analysis. Recently we were able to demonstrate that the C/D ratio is a valuable tool in kidney transplanted patients treated with ER-Tac, showing that the concept of the C/D ratio works for different formulations (159). A recent Korean study also showed that a high C/D ratio correlates with lower delayed graft function rates, less acute rejection lower and increased eGFR 6 months after RTx (160). An association of high Tac clearance with the development of interstitial fibrosis and tubular atrophy (IFTA) was shown by Egeland et al. in 2019 and confirmed to be true for a low C/D ratio in a study 2 years later (161–163). However, we found no differences between slow and fast metabolizers in the incidence of diabetes mellitus, urinary tract infections, and dyslipidemia after transplantation (164–166).
As mentioned above, the results of prospective studies are still lacking. These are needed to make a definitive assessment of the appropriateness of the C/D ratio. In particular, it needs to be clarified whether, and if so which, changes in treatment lead to better outcomes after a low C/D ratio has been detected in individual transplant recipients.
CYP3A5-positivity (mainly CYP3A5*1) is known to be mostly present in fast metabolizers and to be associated with a lower eGFR as well as significantly increased risk of Tac-induced nephrotoxicity (167, 168). Therefore, a correlation of CYP3A5*1 with a low C/D ratio has been expected. Recent studies gained evidence of this assumption and found a strong association of CYP3A5 genotype with a low C/D ratio and a decline in eGFR values (60, 169, 170).
With growing evidence that calculation of the C/D ratio can identify Tac-fast metabolizers and therefore at-risk patients, the timing for assessment remains to be discussed. In a study from our center, patients with a Tac C/D ratio <1.05 ng/ml × 1/mg, calculated 3 months after renal transplantation, were characterized as fast metabolizers and had a significant reduced 5-year patient and overall graft survival, a faster decline in eGFR as well as higher rejection rates within 5 years after renal transplantation (144). An assessment 6 months after RTx also had good discriminative results for eGFR and acute rejection rates (160, 171). However, early assessment of the C/D ratio at day 0–7 did not predict results because too many influencing factors probably converge in the first days after transplantation (172). We conclude from our data that assessment of the C/D ratio should occur no earlier than 1 month, preferably 3 months, after transplantation, because by this time many highly variable factors such as hemoglobin, albumin, steroid dose have undergone some degree of stabilization and the data for the time points are compelling.
So how can the clinical physician optimize the immunosuppressive therapy after identifying a patient with a suspected unfavorable outcome because the patient is a fast metabolizer?
In the last years, we and others investigated this topic by modifying the immunosuppressive regimen either by changing the Tac formulation or switching the immunosuppressive regimen to everolimus or belatacept in selected patients. As fast metabolizers receiving ER-Tac also showed a decline in eGFR and increase of acute rejection rates similar to IR-Tac (159), patients with side effects were indication-based switched from IR- to LCP-Tac. In fast metabolizers LCP-Tac increased the bioavailability, the C/D ratio and was associated with a noticeable recovery of renal function while being safe (145). The same effect on C/D ratio was observed when African Americans, who have predominantly fast metabolism, were switched from IR-Tac to LCP-Tac (118). Conversion to everolimus led to a noticeable increase in renal function in both slow and fast metabolizer groups with a tendency towards a higher increase in fast Tac metabolizers (173). In addition, conversion to belatacept could also be an option, as an increase in eGFR was observed at 12 months post-transplant (174, 175). We hypothesize that the higher rate of adverse effect in fast metabolizers is caused by the higher daily doses required, which ultimately result in higher peak levels with comparable AUC and trough levels (87, 144, 176).
4. Conclusion
Despite its relevant side effects and narrow therapeutic window, Tac is the most commonly prescribed immunosuppressant after renal transplantation. Hence, regular TDM and dose adjustment is necessary, which poses difficulties for clinicians given the high PK variability. Therefore, it is relevant to physicians to know factors influencing its metabolism. A simple and effective tool, the C/D ratio, can classify patients receiving Tac into two major metabolism groups, and therefore helps to predict patientś risk for an unfavorable outcome. Based on this assessment, physicians could optimize and individualize immunosuppressive therapy, e.g., by switching to a different Tac formulation, everolimus or belatacept.
Author contributions
Writing—original draft, LH; Writing—review and editing, UJ, GT, SR. All authors contributed to the article and approved the submitted version.
Funding
The APC was funded by the Open Access Publication Fund of the University of Münster.
Conflict of interest
SR and GT received travel support and lecture fees from Chiesi.
The remaining authors declare that the research was conducted in the absence of any commercial or financial relationships that could be construed as a potential conflict of interest.
The author SR declared that they were an editorial board member of Frontiers, at the time of submission. This had no impact on the peer review process and the final decision.
Publisher's note
All claims expressed in this article are solely those of the authors and do not necessarily represent those of their affiliated organizations, or those of the publisher, the editors and the reviewers. Any product that may be evaluated in this article, or claim that may be made by its manufacturer, is not guaranteed or endorsed by the publisher.
References
1. Hart A, Smith JM, Skeans MA, Gustafson SK, Wilk AR, Castro S, et al. OPTN/SRTR 2018 annual data report: kidney. Am J Transplant. (2020) 20(Suppl 1):20–130. doi: 10.1111/ajt.15672
2. Kidney Disease: Improving Global Outcomes (KDIGO) Transplant Work Group. KDIGO clinical practice guideline for the care of kidney transplant recipients. Am J Transplant. (2009) 9 (Suppl 3):S1–155. doi: 10.1111/j.1600-6143.2009.02834.x
3. Mayer AD, Dmitrewski J, Squifflet JP, Besse T, Grabensee B, Klein B, et al. Multicenter randomized trial comparing tacrolimus (FK506) and cyclosporine in the prevention of renal allograft rejection: a report of the European tacrolimus multicenter renal study group. Transplantation. (1997) 64(3):436–43. doi: 10.1097/00007890-199708150-00012
4. Ekberg H, Tedesco-Silva H, Demirbas A, Vítko S, Nashan B, Gürkan A, et al. Reduced exposure to calcineurin inhibitors in renal transplantation. N Engl J Med. (2007) 357(25):2562–75. doi: 10.1056/NEJMoa067411
5. Adams AB, Goldstein J, Garrett C, Zhang R, Patzer RE, Newell KA, et al. Belatacept combined with transient calcineurin inhibitor therapy prevents rejection and promotes improved long-term renal allograft function. Am J Transplant. (2017) 17(11):2922–36. doi: 10.1111/ajt.14353
6. de Fijter JW, Holdaas H, Øyen O, Sanders JS, Sundar S, Bemelman FJ, et al. Early conversion from calcineurin inhibitor- to everolimus-based therapy following kidney transplantation: results of the randomized ELEVATE trial. Am J Transplant. (2017) 17(7):1853–67. doi: 10.1111/ajt.14186
7. Berger SP, Sommerer C, Witzke O, Tedesco H, Chadban S, Mulgaonkar S, et al. Two-year outcomes in de novo renal transplant recipients receiving everolimus-facilitated calcineurin inhibitor reduction regimen from the TRANSFORM study. Am J Transplant. (2019) 19(11):3018–34. doi: 10.1111/ajt.15480
8. Sommerer C, Suwelack B, Dragun D, Schenker P, Hauser IA, Witzke O, et al. An open-label, randomized trial indicates that everolimus with tacrolimus or cyclosporine is comparable to standard immunosuppression in de novo kidney transplant patients. Kidney Int. (2019) 96(1):231–44. doi: 10.1016/j.kint.2019.01.041
9. Sterneck M, Kaiser GM, Heyne N, Richter N, Rauchfuss F, Pascher A, et al. Long-term follow-up of five year shows superior renal function with everolimus plus early calcineurin inhibitor withdrawal in the PROTECT randomized liver transplantation study. Clin Transplant. (2016) 30(6):741–8. doi: 10.1111/ctr.12744
10. Hauser IA, Marx S, Sommerer C, Suwelack B, Dragun D, Witzke O, et al. Effect of everolimus-based drug regimens on CMV-specific T-cell functionality after renal transplantation: 12-month ATHENA subcohort-study results. Eur J Immunol. (2021) 51(4):943–55. doi: 10.1002/eji.202048855
11. Tedesco-Silva H, Pascual J, Viklicky O, Basic-Jukic N, Cassuto E, Kim DY, et al. Safety of everolimus with reduced calcineurin inhibitor exposure in de novo kidney transplants: an analysis from the randomized TRANSFORM study. Transplantation. (2019) 103(9):1953–63. doi: 10.1097/TP.0000000000002626
12. Sikma MA, van Maarseveen EM, van de Graaf EA, Kirkels JH, Verhaar MC, Donker DW, et al. Pharmacokinetics and toxicity of tacrolimus early after heart and lung transplantation. Am J Transplant. (2015) 15(9):2301–13. doi: 10.1111/ajt.13309
13. Tremblay S, Nigro V, Weinberg J, Woodle ES, Alloway RR. A steady-state head-to-head pharmacokinetic comparison of all FK-506 (tacrolimus) formulations (ASTCOFF): an open-label, prospective, randomized, two-arm, three-period crossover study. Am J Transplant. (2017) 17(2):432–42. doi: 10.1111/ajt.13935
14. Andrews LM, Hesselink DA, van Schaik RHN, van Gelder T, de Fijter JW, Lloberas N, et al. A population pharmacokinetic model to predict the individual starting dose of tacrolimus in adult renal transplant recipients. Br J Clin Pharmacol. (2019) 85(3):601–15. doi: 10.1111/bcp.13838
15. Damon C, Luck M, Toullec L, Etienne I, Buchler M, Hurault de Ligny B, et al. Predictive modeling of tacrolimus dose requirement based on high-throughput genetic screening. Am J Transplant. (2017) 17(4):1008–19. doi: 10.1111/ajt.14040
16. Tang J, Liu R, Zhang YL, Liu MZ, Hu YF, Shao MJ, et al. Application of machine-learning models to predict tacrolimus stable dose in renal transplant recipients. Sci Rep. (2017) 7(1):42192. doi: 10.1038/srep42192
17. Englund G, Lundquist P, Skogastierna C, Johansson J, Hoogstraate J, Afzelius L, et al. Cytochrome p450 inhibitory properties of common efflux transporter inhibitors. Drug Metab Dispos. (2014) 42(3):441–7. doi: 10.1124/dmd.113.054932
18. Pal D, Mitra AK. MDR- and CYP3A4-mediated drug-herbal interactions. Life Sci. (2006) 78(18):2131–45. doi: 10.1016/j.lfs.2005.12.010
19. Masuda S, Uemoto S, Hashida T, Inomata Y, Tanaka K, Inui K. Effect of intestinal P-glycoprotein on daily tacrolimus trough level in a living-donor small bowel recipient. Clin Pharmacol Ther. (2000) 68(1):98–103. doi: 10.1067/mcp.2000.107912
20. Joy MS, Hogan SL, Thompson BD, Finn WF, Nickeleit V. Cytochrome P450 3A5 expression in the kidneys of patients with calcineurin inhibitor nephrotoxicity. Nephrol Dial Transplant. (2007) 22(7):1963–8. doi: 10.1093/ndt/gfm133
21. Iwasaki K. Metabolism of tacrolimus (FK506) and recent topics in clinical pharmacokinetics. Drug Metab Pharmacokinet. (2007) 22(5):328–35. doi: 10.2133/dmpk.22.328
22. de Jonge H, Naesens M, Kuypers DRJ. New insights into the pharmacokinetics and pharmacodynamics of the calcineurin inhibitors and mycophenolic acid: possible consequences for therapeutic drug monitoring in solid organ transplantation. Ther Drug Monit. (2009) 31(4):416–35. doi: 10.1097/FTD.0b013e3181aa36cd
23. Iwasaki K, Shiraga T, Nagase K, Tozuka Z, Noda K, Sakuma S, et al. Isolation, identification, and biological activities of oxidative metabolites of FK506, a potent immunosuppressive macrolide lactone. Drug Metab Dispos. (1993) 21(6):971–7.7507815
24. Zegarska J, Hryniewiecka E, Zochowska D, Samborowska E, Jazwiec R, Borowiec A, et al. Tacrolimus metabolite M-III may have nephrotoxic and myelotoxic effects and increase the incidence of infections in kidney transplant recipients. Transplant Proc. (2016) 48(5):1539–42. doi: 10.1016/j.transproceed.2015.12.133
25. EMA. Prograf—article 30 referral—annex I, II, III. (2006). Available at: https://www.ema.europa.eu/en/documents/referral/prograf-article-30-referral-annex-i-ii-iii_en.pdf.
26. Tuteja S, Alloway RR, Johnson JA, Gaber AO. The effect of gut metabolism on tacrolimus bioavailability in renal transplant recipients. Transplantation. (2001) 71(9):1303–7. doi: 10.1097/00007890-200105150-00021
27. Zhang Y, Benet LZ. The gut as a barrier to drug absorption: combined role of cytochrome P450 3A and P-glycoprotein. Clin Pharmacokinet. (2001) 40(3):159–68. doi: 10.2165/00003088-200140030-00002
28. Sattler M, Guengerich FP, Yun CH, Christians U, Sewing KF. Cytochrome P-450 3A enzymes are responsible for biotransformation of FK506 and rapamycin in man and rat. Drug Metab Dispos. (1992) 20(5):753–61.1385058
29. Perotti BY, Okudaira N, Prueksaritanont T, Benet LZ. FK 506 metabolism in male and female rat liver microsomes. Drug Metab Dispos. (1994) 22(1):85–9.7512020
30. Möller A, Iwasaki K, Kawamura A, Teramura Y, Shiraga T, Hata T, et al. The disposition of 14C-labeled tacrolimus after intravenous and oral administration in healthy human subjects. Drug Metab Dispos. (1999) 27(6):633–6.
31. Guo Y, Crnkovic CM, Won KJ, Yang X, Lee JR, Orjala J, et al. Commensal gut bacteria convert the immunosuppressant tacrolimus to less potent metabolites. Drug Metab Dispos. (2019) 47(3):194–202. doi: 10.1124/dmd.118.084772
32. Venkataramanan R, Swaminathan A, Prasad T, Jain A, Zuckerman S, Warty V, et al. Clinical pharmacokinetics of tacrolimus. Clin Pharmacokinet. (1995) 29(6):404–30. doi: 10.2165/00003088-199529060-00003
33. Kuypers DRJ, Claes K, Evenepoel P, Maes B, Vanrenterghem Y. The rate of gastric emptying determines the timing but not the extent of oral tacrolimus absorption: simultaneous measurement of drug exposure and gastric emptying by carbon-14-octanoic acid breath test in stable renal allograft recipients. Drug Metab Dispos. (2004) 32(12):1421–5. doi: 10.1124/dmd.104.001503
34. Park S-I, Felipe CR, Pinheiro-Machado PG, Garcia R, Tedesco-Silva H, Medina-Pestana JO. Circadian and time-dependent variability in tacrolimus pharmacokinetics. Fundam Clin Pharmacol. (2007) 21(2):191–7. doi: 10.1111/j.1472-8206.2007.00468.x
35. Hardinger KL, Park JM, Schnitzler MA, Koch MJ, Miller BW, Brennan DC. Pharmacokinetics of tacrolimus in kidney transplant recipients: twice daily versus once daily dosing. Am J Transplant. (2004) 4(4):621–5. doi: 10.1111/j.1600-6143.2004.00383.x
36. Satoh S, Kagaya H, Saito M, Inoue T, Miura M, Inoue K, et al. Lack of tacrolimus circadian pharmacokinetics and CYP3A5 pharmacogenetics in the early and maintenance stages in Japanese renal transplant recipients. Br J Clin Pharmacol. (2008) 66(2):207–14. doi: 10.1111/j.1365-2125.2008.03188.x
37. Satoh S, Tada H, Tachiki Y, Tsuchiya N, Shimoda N, Akao T, et al. Chrono and clinical pharmacokinetic study of tacrolimus in continuous intravenous administration. Int J Urol. (2001) 8(7):353–8. doi: 10.1046/j.1442-2042.2001.00313.x
38. Fontova P, Colom H, Rigo-Bonnin R, van Merendonk LN, Vidal-Alabró A, Montero N, et al. Influence of the circadian timing system on tacrolimus pharmacokinetics and pharmacodynamics after kidney transplantation. Front Pharmacol. (2021) 12:636048. doi: 10.3389/fphar.2021.636048
39. Bekersky I, Dressler D, Mekki QA. Effect of low- and high-fat meals on tacrolimus absorption following 5 mg single oral doses to healthy human subjects. J Clin Pharmacol. (2001) 41(2):176–82. doi: 10.1177/00912700122009999
40. Bekersky I, Dressler D, Mekki Q. Effect of time of meal consumption on bioavailability of a single oral 5 mg tacrolimus dose. J Clin Pharmacol. (2001) 41(3):289–97. doi: 10.1177/00912700122010104
41. Liu C, Shang YF, Zhang XF, Zhang XG, Wang B, Wu Z, et al. Co-administration of grapefruit juice increases bioavailability of tacrolimus in liver transplant patients: a prospective study. Eur J Clin Pharmacol. (2009) 65(9):881–5. doi: 10.1007/s00228-009-0702-z
42. Miedziaszczyk M, Bajon A, Jakielska E, Primke M, Sikora J, Skowrońska D, et al. Controversial interactions of tacrolimus with dietary supplements, herbs and food. Pharmaceutics. (2022) 14(10):2154. doi: 10.3390/pharmaceutics14102154
43. Kolars J, Watkins P, Merion R, Awni W. First-pass metabolism of cyclosporin by the gut. Lancet. (1991) 338(8781):1488–90. doi: 10.1016/0140-6736(91)92302-i
44. Berggren S, Gall C, Wollnitz N, Ekelund M, Karlbom U, Hoogstraate J, et al. Gene and protein expression of P-glycoprotein, MRP1, MRP2, and CYP3A4 in the small and large human intestine. Mol Pharm. (2007) 4(2):252–7. doi: 10.1021/mp0600687
45. Canaparo R, Finnström N, Serpe L, Nordmark A, Muntoni E, Eandi M, et al. Expression of CYP3A isoforms and P-glycoprotein in human stomach, jejunum and ileum. Clin Exp Pharmacol Physiol. (2007) 34(11):1138–44. doi: 10.1111/j.1440-1681.2007.04691.x
46. Mouly S, Paine MF. P-glycoprotein increases from proximal to distal regions of human small intestine. Pharm Res. (2003) 20(10):1595–9. doi: 10.1023/a:1026183200740
47. Vanhove T, Annaert P, Kuypers DRJ. Clinical determinants of calcineurin inhibitor disposition: a mechanistic review. Drug Metab Rev. (2016) 48(1):88–112. doi: 10.3109/03602532.2016.1151037
48. Tsunashima D, Kawamura A, Murakami M, Sawamoto T, Undre N, Brown M, et al. Assessment of tacrolimus absorption from the human intestinal tract: open-label, randomized, 4-way crossover study. Clin Ther. (2014) 36(5):748–59. doi: 10.1016/j.clinthera.2014.02.021
49. Lemahieu W, Maes B, Verbeke K, Rutgeerts P, Geboes K, Vanrenterghem Y. Cytochrome P450 3A4 and P-glycoprotein activity and assimilation of tacrolimus in transplant patients with persistent diarrhea. Am J Transplant. (2005) 5(6):1383–91. doi: 10.1111/j.1600-6143.2005.00844.x
50. Sato K, Amada N, Sato T, Miura S, Ohashi Y, Sekiguchi S, et al. Severe elevations of FK506 blood concentration due to diarrhea in renal transplant recipients. Clin Transplant. (2004) 18(5):585–90. doi: 10.1111/j.1399-0012.2004.00232.x
51. Picard N, Bergan S, Marquet P, van Gelder T, Wallemacq P, Hesselink DA, et al. Pharmacogenetic biomarkers predictive of the pharmacokinetics and pharmacodynamics of immunosuppressive drugs. Ther Drug Monit. (2016) 38(Suppl 1):S57–69. doi: 10.1097/FTD.0000000000000255
52. Oetting WS, Schladt DP, Guan W, Miller MB, Remmel RP, Dorr C, et al. Genomewide association study of tacrolimus concentrations in African American kidney transplant recipients identifies multiple CYP3A5 alleles. Am J Transplant. (2016) 16(2):574–82. doi: 10.1111/ajt.13495
53. Thölking G, Gerth HU, Schuette-Nuetgen K, Reuter S. Influence of tacrolimus metabolism rate on renal function after solid organ transplantation. World J Transplant. (2017) 7(1):26. doi: 10.5500/wjt.v7.i1.26
54. Vanhove T, de Jonge H, de Loor H, Oorts M, de Hoon J, Pohanka A, et al. Relationship between in vivo CYP3A4 activity, CYP3A5 genotype, and systemic tacrolimus metabolite/parent drug ratio in renal transplant recipients and healthy volunteers. Drug Metab Dispos. (2018) 46(11):1507–13. doi: 10.1124/dmd.118.081935
55. Nuchjumroon A, Vadcharavivad S, Singhan W, Poosoonthornsri M, Chancharoenthana W, Udomkarnjananun S, et al. Comparison of tacrolimus intra-patient variability during 6-12 months after kidney transplantation between CYP3A5 expressers and nonexpressers. J Clin Med. (2022) 11(21):6320. doi: 10.3390/jcm11216320
56. Choi JS, Ko H, Kim HK, Chung C, Han A, Min SK, et al. Effects of tacrolimus intrapatient variability and CYP3A5 polymorphism on the outcomes of pediatric kidney transplantation. Pediatr Transplant. (2022) 26(6):e14297. doi: 10.1111/petr.14297
57. Chen SY, Li JL, Meng FH, Wang XD, Liu T, Li J, et al. Individualization of tacrolimus dosage basing on cytochrome P450 3A5 polymorphism–a prospective, randomized, controlled study. Clin Transplant. (2013) 27(3):E272–81. doi: 10.1111/ctr.12101
58. Boughton O, Borgulya G, Cecconi M, Fredericks S, Moreton-Clack M, MacPhee IAM. A published pharmacogenetic algorithm was poorly predictive of tacrolimus clearance in an independent cohort of renal transplant recipients. Br J Clin Pharmacol. (2013) 76(3):425–31. doi: 10.1111/bcp.12076
59. Shuker N, Bouamar R, van Schaik RH, Clahsen-van Groningen MC, Damman J, Baan CC, et al. A randomized controlled trial comparing the efficacy of Cyp3a5 genotype-based with body-weight-based tacrolimus dosing after living donor kidney transplantation. Am J Transplant. (2016) 16(7):2085–96. doi: 10.1111/ajt.13691
60. Cheng F, Li Q, Cui Z, Wang Z, Zeng F, Zhang Y. Tacrolimus concentration is effectively predicted using combined clinical and genetic factors in the perioperative period of kidney transplantation and associated with acute rejection. J Immunol Res. (2022) 2022:3129389. doi: 10.1155/2022/3129389
61. Venkataramanan R, Jain A, Warty VW, Abu-Elmagd K, Furakawa H, Imventarza O, et al. Pharmacokinetics of FK 506 following oral administration: a comparison of FK 506 and cyclosporine. Transplant Proc. (1991) 23(1 Pt 2):931–3.1703355
62. Biagiotti S, Paoletti MF, Fraternale A, Rossi L, Magnani M. Drug delivery by red blood cells. IUBMB Life. (2011) 63(8):621–31. doi: 10.1002/iub.478
63. Nakazawa R, Yoshiike M, Nozawa S, Aida K, Katsuoka Y, Fujimoto E, et al. Clinically useful limited sampling strategy to estimate area under the concentration-time curve of once-daily tacrolimus in adult Japanese kidney transplant recipients. PLoS One. (2019) 14(12):e0225878. doi: 10.1371/journal.pone.0225878
64. El-Nahhas T, Popoola J, MacPhee I, Johnston A. Limited sampling strategies for estimation of tacrolimus exposure in kidney transplant recipients receiving extended-release tacrolimus preparation. Clin Transl Sci. (2022) 15(1):70–8. doi: 10.1111/cts.12990
65. Vadcharavivad S, Susomboon T, Kulabusaya B, Avihingsanon Y, Praditpornsilpa K, Townamchai N. Validation of a 2-point limited sampling strategy to predict the tacrolimus area-under-the-12-hour-curve in kidney transplant recipients. Ther Drug Monit. (2016) 38(5):614–20. doi: 10.1097/FTD.0000000000000317
66. Wallemacq P, Armstrong VW, Brunet M, Haufroid V, Holt DW, Johnston A, et al. Opportunities to optimize tacrolimus therapy in solid organ transplantation: report of the European consensus conference. Ther Drug Monit. (2009) 31(2):139–52. doi: 10.1097/FTD.0b013e318198d092
67. Seger C, Shipkova M, Christians U, Billaud EM, Wang P, Holt DW, et al. Assuring the proper analytical performance of measurement procedures for immunosuppressive drug concentrations in clinical practice: recommendations of the international association of therapeutic drug monitoring and clinical toxicology immunosuppressive drug scientific committee. Ther Drug Monit. (2016) 38(2):170–89. doi: 10.1097/FTD.0000000000000269
68. Seger C. Usage and limitations of liquid chromatography-tandem mass spectrometry (LC-MS/MS) in clinical routine laboratories. Wien Med Wochenschr. (2012) 162(21-22):499–504. doi: 10.1007/s10354-012-0147-3
69. Shigematsu T, Suetsugu K, Yamamoto N, Tsuchiya Y, Masuda S. Comparison of 4 commercial immunoassays used in measuring the concentration of tacrolimus in blood and their cross-reactivity to its metabolites. Ther Drug Monit. (2020) 42(3):400–6. doi: 10.1097/FTD.0000000000000696
70. Mathieu E, Duterme C, Fage D, Cotton F. Cascadion™ SM clinical analyzer: evaluation of the whole blood immunosuppressants quantification and routine usability. Clin Chim Acta. (2023) 539:97–104. doi: 10.1016/j.cca.2022.11.029
71. Shipkova M, Valbuena H. Liquid chromatography tandem mass spectrometry for therapeutic drug monitoring of immunosuppressive drugs: achievements, lessons and open issues. Trends Anal Chem. (2016) 84:23–33. doi: 10.1016/j.trac.2016.01.031
72. Jusko WJ, D'Ambrosio R. Monitoring FK 506 concentrations in plasma and whole blood. Transplant Proc. (1991) 23(6):2732–5.1721260
73. Yoshikawa N, Yokota T, Matsuo A, Matsumoto N, Iwakiri T, Ikeda R. Role of FK506 binding protein on tacrolimus distribution in red blood cells. Pharm Res. (2020) 37(7):143. doi: 10.1007/s11095-020-02875-z
74. Piekoszewski W, Jusko WJ. Plasma protein binding of tacrolimus in humans. J Pharm Sci. (1993) 82(3):340–1. doi: 10.1002/jps.2600820325
75. Størset E, Holford N, Midtvedt K, Bremer S, Bergan S, Åsberg A. Importance of hematocrit for a tacrolimus target concentration strategy. Eur J Clin Pharmacol. (2014) 70(1):65–77. doi: 10.1007/s00228-013-1584-7
76. Staatz CE, Størset E, Bergmann TK, Hennig S, Holford N. Tacrolimus pharmacokinetics after kidney transplantation–influence of changes in haematocrit and steroid dose. Br J Clin Pharmacol. (2015) 80(6):1475–6. doi: 10.1111/bcp.12729
77. Hebert MF, Zheng S, Hays K, Shen DD, Davis CL, Umans JG, et al. Interpreting tacrolimus concentrations during pregnancy and postpartum. Transplantation. (2013) 95(7):908–15. doi: 10.1097/TP.0b013e318278d367
78. Sikma MA, Hunault CC, Van Maarseveen EM, Huitema ADR, Van de Graaf EA, Kirkels JH, et al. High variability of whole-blood tacrolimus pharmacokinetics early after thoracic organ transplantation. Eur J Drug Metab Pharmacokinet. (2020) 45(1):123–34. doi: 10.1007/s13318-019-00591-7
79. Sikma MA, Van Maarseveen EM, Hunault CC, Moreno JM, Van de Graaf EA, Kirkels JH, et al. Unbound plasma, total plasma, and whole-blood tacrolimus pharmacokinetics early after thoracic organ transplantation. Clin Pharmacokinet. (2020) 59(6):771–80. doi: 10.1007/s40262-019-00854-1
80. Francke MI, Hesselink DA, Li Y, Koch BCP, de Wit LEA, van Schaik RHN, et al. Monitoring the tacrolimus concentration in peripheral blood mononuclear cells of kidney transplant recipients. Br J Clin Pharmacol. (2021) 87(4):1918–29. doi: 10.1111/bcp.14585
81. Francke MI, Andrews LM, Lan Le H, van de Velde D, Dieterich M, Udomkarnjananun S, et al. Monitoring intracellular tacrolimus concentrations and its relationship with rejection in the early phase after renal transplantation. Clin Biochem. (2022) 101:9–15. doi: 10.1016/j.clinbiochem.2021.12.002
82. Han SS, Yang SH, Kim MC, Cho JY, Min SI, Lee JP, et al. Monitoring the intracellular tacrolimus concentration in kidney transplant recipients with stable graft function. PLoS One. (2016) 11(4):e0153491. doi: 10.1371/journal.pone.0153491
83. Bekersky I, Dressler D, Alak A, Boswell GW, Mekki QA. Comparative tacrolimus pharmacokinetics: normal versus mildly hepatically impaired subjects. J Clin Pharmacol. (2001) 41(6):628–35. doi: 10.1177/00912700122010519
84. Naesens M, Kuypers DRJ, Sarwal M. Calcineurin inhibitor nephrotoxicity. Clin J Am Soc Nephrol. (2009) 4(2):481–508. doi: 10.2215/CJN.04800908
85. Sallustio BC, Noll BD, Hu R, Barratt DT, Tuke J, Coller JK, et al. Tacrolimus dose, blood concentrations and acute nephrotoxicity, but not CYP3A5/ABCB1 genetics, are associated with allograft tacrolimus concentrations in renal transplant recipients. Br J Clin Pharmacol. (2021) 87(10):3901–9. doi: 10.1111/bcp.14806
86. Zhang M, Tajima S, Shigematsu T, Fu R, Noguchi H, Kaku K, et al. Donor CYP3A5 gene polymorphism alone cannot predict tacrolimus intrarenal concentration in renal transplant recipients. Int J Mol Sci. (2020) 21(8):2976. doi: 10.3390/ijms21082976
87. Thölking G, Schütte-Nütgen K, Schmitz J, Rovas A, Dahmen M, Bautz J, et al. A low tacrolimus concentration/dose ratio increases the risk for the development of acute calcineurin inhibitor-induced nephrotoxicity. J Clin Med. (2019) 8(10):1586. doi: 10.3390/jcm8101586
88. Christians U, Jacobsen W, Benet LZ, Lampen A. Mechanisms of clinically relevant drug interactions associated with tacrolimus. Clin Pharmacokinet. (2002) 41(11):813–51. doi: 10.2165/00003088-200241110-00003
89. Zhou S-F, Xue CC, Yu X-Q, Li C, Wang G. Clinically important drug interactions potentially involving mechanism-based inhibition of cytochrome P450 3A4 and the role of therapeutic drug monitoring. Ther Drug Monit. (2007) 29(6):687–710. doi: 10.1097/FTD.0b013e31815c16f5
90. Bril F, Castro V, Centurion IG, Espinosa J, Keller GA, Gonzalez CD, et al. A systematic approach to assess the burden of drug interactions in adult kidney transplant patients. Curr Drug Saf. (2016) 11(2):156–63. doi: 10.2174/157488631102160429003742
91. Lemaitre F, Budde K, Van Gelder T, Bergan S, Lawson R, Noceti O, et al. Therapeutic drug monitoring and dosage adjustments of immunosuppressive drugs when combined with nirmatrelvir/ritonavir in patients with COVID-19. Ther Drug Monit. (2023) 45(2):191–9. doi: 10.1097/FTD.0000000000001014
92. Pescovitz MD, Bloom R, Pirsch J, Johnson J, Gelone S, Villano SA. A randomized, double-blind, pharmacokinetic study of oral maribavir with tacrolimus in stable renal transplant recipients. Am J Transplant. (2009) 9(10):2324–30. doi: 10.1111/j.1600-6143.2009.02768.x
93. Huppertz A, Ott C, Bruckner T, Foerster KI, Burhenne J, Weiss J, et al. Prolonged-release tacrolimus is less susceptible to interaction with the strong CYP3A inhibitor voriconazole in healthy volunteers. Clin Pharmacol Ther. (2019) 106(6):1290–8. doi: 10.1002/cpt.1529
94. Bolley R, Zülke C, Kammerl M, Fischereder M, Krämer BK. Tacrolimus-induced nephrotoxicity unmasked by induction of the CYP3A4 system with St John’s wort. Transplantation. (2002) 73(6):1009. doi: 10.1097/00007890-200203270-00035
95. Anglicheau D, Flamant M, Schlageter MH, Martinez F, Cassinat B, Beaune P, et al. Pharmacokinetic interaction between corticosteroids and tacrolimus after renal transplantation. Nephrol Dial Transplant. (2003) 18(11):2409–14. doi: 10.1093/ndt/gfg381
96. Stratta P, Quaglia M, Cena T, Antoniotti R, Fenoglio R, Menegotto A, et al. The interactions of age, sex, body mass index, genetics, and steroid weight-based doses on tacrolimus dosing requirement after adult kidney transplantation. Eur J Clin Pharmacol. (2012) 68(5):671–80. doi: 10.1007/s00228-011-1150-0
97. Wallemacq PE, Verbeeck RK. Comparative clinical pharmacokinetics of tacrolimus in paediatric and adult patients. Clin Pharmacokinet. (2001) 40(4):283–95. doi: 10.2165/00003088-200140040-00004
98. Shishido S, Asanuma H, Tajima E, Honda M, Nakai H. Pharmacokinetics of tacrolimus in pediatric renal transplant recipients. Transplant Proc. (2001) 33(1-2):1066–8. doi: 10.1016/s0041-1345(00)02418-0
99. Fanta S, Jönsson S, Backman JT, Karlsson MO, Hoppu K. Developmental pharmacokinetics of ciclosporin–a population pharmacokinetic study in paediatric renal transplant candidates. Br J Clin Pharmacol. (2007) 64(6):772–84. doi: 10.1111/j.1365-2125.2007.03003.x.
100. Krenzien F, Quante M, Heinbokel T, Seyda M, Minami K, Uehara H, et al. Age-dependent metabolic and immunosuppressive effects of tacrolimus. Am J Transplant. (2017) 17(5):1242–54. doi: 10.1111/ajt.14087
101. Krenzien F, ElKhal A, Quante M, Rodriguez Cetina Biefer H, Hirofumi U, Gabardi S, et al. A rationale for age-adapted immunosuppression in organ transplantation. Transplantation. (2015) 99(11):2258–68. doi: 10.1097/TP.0000000000000842
102. Miura M, Satoh S, Kagaya H, Saito M, Inoue T, Tsuchiya N, et al. No impact of age on dose-adjusted pharmacokinetics of tacrolimus, mycophenolic acid and prednisolone 1 month after renal transplantation. Eur J Clin Pharmacol. (2009) 65(10):1047–53. doi: 10.1007/s00228-009-0721-9
103. Staatz CE, Tett SE. Pharmacokinetic considerations relating to tacrolimus dosing in the elderly. Drugs Aging. (2005) 22(7):541–57. doi: 10.2165/00002512-200522070-00001
104. Passey C, Birnbaum AK, Brundage RC, Oetting WS, Israni AK, Jacobson PA. Dosing equation for tacrolimus using genetic variants and clinical factors. Br J Clin Pharmacol. (2011) 72(6):948–57. doi: 10.1111/j.1365-2125.2011.04039.x
105. Jacobson PA, Schladt D, Oetting WS, Leduc R, Guan W, Matas AJ, et al. Lower calcineurin inhibitor doses in older compared to younger kidney transplant recipients yield similar troughs. Am J Transplant. (2012) 12(12):3326–36. doi: 10.1111/j.1600-6143.2012.04232.x
106. Thölking G, Fortmann C, Koch R, Gerth HU, Pabst D, Pavenstädt H, et al. The tacrolimus metabolism rate influences renal function after kidney transplantation. PLoS One. (2014) 9(10):e111128. doi: 10.1371/journal.pone.0111128
107. David-Neto E, Romano P, Kamada Triboni AH, Ramos F, Agena F, Almeida Rezende Ebner P, et al. Longitudinal pharmacokinetics of tacrolimus in elderly compared with younger recipients in the first 6 months after renal transplantation. Transplantation. (2017) 101(6):1365–72. doi: 10.1097/TP.0000000000001369
108. Bunnapradist S, Rostaing L, Alloway RR, West-Thielke P, Denny J, Mulgaonkar S, et al. LCPT once-daily extended-release tacrolimus tablets versus twice-daily capsules: a pooled analysis of two phase 3 trials in important de novo and stable kidney transplant recipient subgroups. Transpl Int. (2016) 29(5):603–11. doi: 10.1111/tri.12770
109. Kuypers DR, Claes K, Evenepoel P, Maes B, Coosemans W, Pirenne J, et al. Time-related clinical determinants of long-term tacrolimus pharmacokinetics in combination therapy with mycophenolic acid and corticosteroids: a prospective study in one hundred de novo renal transplant recipients. Clin Pharmacokinet. (2004) 43(11):741–62. doi: 10.2165/00003088-200443110-00005
110. Velicković-Radovanović R, Mikov M, Paunović G, Djordjević V, Stojanović M, Cvetković T, et al. Gender differences in pharmacokinetics of tacrolimus and their clinical significance in kidney transplant recipients. Gend Med. (2011) 8(1):23–31. doi: 10.1016/j.genm.2011.01.003
111. Tornatore KM, Meaney CJ, Attwood K, Brazeau DA, Wilding GE, Consiglio JD, et al. Race and sex associations with tacrolimus pharmacokinetics in stable kidney transplant recipients. Pharmacotherapy. (2022) 42(2):94–105. doi: 10.1002/phar.2656
112. Aktürk S, Çelebi ZK, Erdoğmuş Ş, Kanmaz AG, Yüce T, Şengül Ş, et al. Pregnancy after kidney transplantation: outcomes, tacrolimus doses, and trough levels. Transplant Proc. (2015) 47(5):1442–4. doi: 10.1016/j.transproceed.2015.04.041
113. Chandra A, Midtvedt K, Åsberg A, Eide IA. Immunosuppression and reproductive health after kidney transplantation. Transplantation. (2019) 103(11):e325–33. doi: 10.1097/TP.0000000000002903
114. Felipe CR, Silva HT, Machado PGP, Garcia R, Da Silva Moreira SR, Pestana JOM. The impact of ethnic miscegenation on tacrolimus clinical pharmacokinetics and therapeutic drug monitoring. Clin Transplant. (2002) 16(4):262–72. doi: 10.1034/j.1399-0012.2002.01103.x
115. Kim JS, Aviles DH, Silverstein DM, Leblanc PL, Matti Vehaskari V. Effect of age, ethnicity, and glucocorticoid use on tacrolimus pharmacokinetics in pediatric renal transplant patients. Pediatr Transplant. (2005) 9(2):162–9. doi: 10.1111/j.1399-3046.2005.00263.x
116. Felipe C, Garcia C, Moreira S, Olsen N, Silva H, Pestana O. Choosing the right dose of new immunossuppressive drugs for new populations: importance of pharmacokinetic studies. Transplant Proc. (2001) 33(1–2):1095–6. doi: 10.1016/s0041-1345(00)02432-5
117. Mohamed ME, Schladt DP, Guan W, Wu B, van Setten J, Keating BJ, et al. Tacrolimus troughs and genetic determinants of metabolism in kidney transplant recipients: a comparison of four ancestry groups. Am J Transplant. (2019) 19(10):2795–804. doi: 10.1111/ajt.15385
118. Trofe-Clark J, Brennan DC, West-Thielke P, Milone MC, Lim MA, Neubauer R, et al. Results of ASERTAA, a randomized prospective crossover pharmacogenetic study of immediate-release versus extended-release tacrolimus in African American kidney transplant recipients. Am J Kidney Dis. (2018) 71(3):315–26. doi: 10.1053/j.ajkd.2017.07.018
119. Grover A, Frassetto LA, Benet LZ, Chakkera HA. Pharmacokinetic differences corroborate observed low tacrolimus dosage in native American renal transplant patients. Drug Metab Dispos. (2011) 39(11):2017–9. doi: 10.1124/dmd.111.041350
120. de Jonge H, Vanhove T, de Loor H, Verbeke K, Kuypers DRJ. Progressive decline in tacrolimus clearance after renal transplantation is partially explained by decreasing CYP3A4 activity and increasing haematocrit. Br J Clin Pharmacol. (2015) 80(3):548–59. doi: 10.1111/bcp.12703
121. Undre NA, Schäfer A. Factors affecting the pharmacokinetics of tacrolimus in the first year after renal transplantation. Transplant Proc. (1998) 30(4):1261–3. doi: 10.1016/s0041-1345(98)00234-6
122. Kocur A, Kunicki PK, Pawiński T. Generic medicinal products in immunosuppressive therapy-should it be a challenge for therapeutic drug monitoring? Ther Drug Monit. (2023) 45(2):173–90. doi: 10.1097/FTD.0000000000001041
123. Food and Drug Administration. 210115Orig1s000. (2018). Available at: https://www.accessdata.fda.gov/drugsatfda_docs/nda/2018/210115Orig1s000ClinPharmR.pdf.
124. Shuker N, Shuker L, van Rosmalen J, Roodnat JI, Borra LC, Weimar W, et al. A high intrapatient variability in tacrolimus exposure is associated with poor long-term outcome of kidney transplantation. Transpl Int. (2016) 29(11):1158–67. doi: 10.1111/tri.12798
125. Park Y, Lee H, Eum SH, Ko EJ, Min JW, Yoon SH, et al. Combined impact of the inter and intra-patient variability of tacrolimus blood level on allograft outcomes in kidney transplantation. Front Immunol. (2022) 13:1037566. doi: 10.3389/fimmu.2022.1037566
126. Mendoza Rojas A, Hesselink DA, van Besouw NM, Dieterich M, de Kuiper R, Baan CC, et al. High tacrolimus intrapatient variability and subtherapeutic immunosuppression are associated with adverse kidney transplant outcomes. Ther Drug Monit. (2022) 44(3):369–76. doi: 10.1097/FTD.0000000000000955
127. Shen C-L, Yang A-H, Lien T-J, Tarng D-C, Yang C-Y. Tacrolimus blood level fluctuation predisposes to coexisting BK virus nephropathy and acute allograft rejection. Sci Rep. (2017) 7(1):1986. doi: 10.1038/s41598-017-02140-1
128. Rodrigo E, Segundo DS, Fernández-Fresnedo G, López-Hoyos M, Benito A, Ruiz JC, et al. Within-patient variability in tacrolimus blood levels predicts kidney graft loss and donor-specific antibody development. Transplantation. (2016) 100(11):2479–85. doi: 10.1097/TP.0000000000001040
129. Shemesh E, Bucuvalas JC, Anand R, Mazariegos GV, Alonso EM, Venick RS, et al. The medication level variability index (MLVI) predicts poor liver transplant outcomes: a prospective multi-site study. Am J Transplant. (2017) 17(10):2668–78. doi: 10.1111/ajt.14276
130. Piotti G, Cremaschi E, Maggiore U. Once-daily prolonged-release tacrolimus formulations for kidney transplantation: what the nephrologist needs to know. J Nephrol. (2017) 30(1):53–61. doi: 10.1007/s40620-016-0316-3
131. Sánchez Fructuoso A, Ruiz JC, Franco A, Diekmann F, Redondo D, Calviño J, et al. Effectiveness and safety of the conversion to MeltDose® extended-release tacrolimus from other formulations of tacrolimus in stable kidney transplant patients: a retrospective study. Clin Transplant. (2020) 34(1):e13767. doi: 10.1111/ctr.13767
132. Gaber AO, Alloway RR, Bodziak K, Kaplan B, Bunnapradist S. Conversion from twice-daily tacrolimus capsules to once-daily extended-release tacrolimus (LCPT): a phase 2 trial of stable renal transplant recipients. Transplantation. (2013) 96(2):191–7. doi: 10.1097/TP.0b013e3182962cc1
133. Alloway RR, Eckhoff DE, Washburn WK, Teperman LW. Conversion from twice daily tacrolimus capsules to once daily extended-release tacrolimus (LCP-tacro): phase 2 trial of stable liver transplant recipients. Liver Transpl. (2014) 20(5):564–75. doi: 10.1002/lt.23844
134. Glander P, Waiser J, Kasbohm S, Friedersdorff F, Peters R, Rudolph B, et al. Bioavailability and costs of once-daily and twice-daily tacrolimus formulations in de novo kidney transplantation. Clin Transplant. (2018) 32(8):e13311. doi: 10.1111/ctr.13311
135. Niioka T, Satoh S, Kagaya H, Numakura K, Inoue T, Saito M, et al. Comparison of pharmacokinetics and pharmacogenetics of once- and twice-daily tacrolimus in the early stage after renal transplantation. Transplantation. (2012) 94(10):1013–9. doi: 10.1097/TP.0b013e31826bc400
136. Tsuchiya T, Ishida H, Tanabe T, Shimizu T, Honda K, Omoto K, et al. Comparison of pharmacokinetics and pathology for low-dose tacrolimus once-daily and twice-daily in living kidney transplantation: prospective trial in once-daily versus twice-daily tacrolimus. Transplantation. (2013) 96(2):198–204. doi: 10.1097/TP.0b013e318296c9d5
137. Stifft F, Stolk LML, Undre N, van Hooff JP, Christiaans MHL. Lower variability in 24-hour exposure during once-daily compared to twice-daily tacrolimus formulation in kidney transplantation. Transplantation. (2014) 97(7):775–80. doi: 10.1097/01.TP.0000437561.31212.0e
138. Barreto P, Malheiro J, Vieira P, Pedroso S, Almeida M, Martins LS, et al. Conversion from twice-daily to once-daily tacrolimus in stable kidney graft recipients. Transplant Proc. (2016) 48(7):2276–9. doi: 10.1016/j.transproceed.2016.06.020
139. Grinyó JM, Petruzzelli S. Once-daily LCP-tacro MeltDose tacrolimus for the prophylaxis of organ rejection in kidney and liver transplantations. Expert Rev Clin Immunol. (2014) 10(12):1567–79. doi: 10.1586/1744666X.2014.983903
140. Bunthof KLW, Al-Hassany L, Nakshbandi G, Hesselink DA, van Schaik RHN, Ten Dam MAGJ, et al. A randomized crossover study comparing different tacrolimus formulations to reduce intrapatient variability in tacrolimus exposure in kidney transplant recipients. Clin Transl Sci. (2022) 15(4):930–41. doi: 10.1111/cts.13206
141. Budde K, Rostaing L, Maggiore U, Piotti G, Surace D, Geraci S, et al. Prolonged-release once-daily formulation of tacrolimus versus standard-of-care tacrolimus in de novo kidney transplant patients across Europe. Transpl Int. (2022) 35:10225. doi: 10.3389/ti.2021.10225
142. Fernandez Rivera C, Calvo Rodríguez M, Poveda JL, Pascual J, Crespo M, Gomez G, et al. Bioavailability of once-daily tacrolimus formulations used in clinical practice in the management of de novo kidney transplant recipients: the better study. Clin Transplant. (2022) 36(3):e14550. doi: 10.1111/ctr.14550
143. Langone A, Steinberg SM, Gedaly R, Chan LK, Shah T, Sethi KD, et al. Switching study of kidney transplant patients with tremor to LCP-TacrO (STRATO): an open-label, multicenter, prospective phase 3b study. Clin Transplant. (2015) 29(9):796–805. doi: 10.1111/ctr.12581
144. Schütte-Nütgen K, Thölking G, Steinke J, Pavenstädt H, Schmidt R, Suwelack B, et al. Fast tac metabolizers at riskis—it is time for a C/D ratio calculation. J Clin Med. (2019) 8(5):587. doi: 10.3390/jcm8050587
145. Thölking G, Tosun-Koç F, Jehn U, Koch R, Pavenstädt H, Suwelack B, et al. Improved kidney allograft function after early conversion of fast IR-tac metabolizers to LCP-tac. J Clin Med. (2022) 11(5):1290. doi: 10.3390/jcm11051290
146. von Einsiedel J, Thölking G, Wilms C, Vorona E, Bokemeyer A, Schmidt HH, et al. Conversion from standard-release tacrolimus to MeltDose® tacrolimus (LCPT) improves renal function after liver transplantation. J Clin Med. (2020) 9(6):1654. doi: 10.3390/jcm9061654
147. Sellarés J, de Freitas DG, Mengel M, Reeve J, Einecke G, Sis B, et al. Understanding the causes of kidney transplant failure: the dominant role of antibody-mediated rejection and nonadherence. Am J Transplant. (2012) 12(2):388–99. doi: 10.1111/j.1600-6143.2011.03840.x
148. Obi Y, Ichimaru N, Kato T, Kaimori JY, Okumi M, Yazawa K, et al. A single daily dose enhances the adherence to immunosuppressive treatment in kidney transplant recipients: a cross-sectional study. Clin Exp Nephrol. (2013) 17(2):310–5. doi: 10.1007/s10157-012-0713-4
149. Kuypers DR, Peeters PC, Sennesael JJ, Kianda MN, Vrijens B, Kristanto P, et al. Improved adherence to tacrolimus once-daily formulation in renal recipients: a randomized controlled trial using electronic monitoring. Transplantation. (2013) 95(2):333–40. doi: 10.1097/TP.0b013e3182725532
150. van Boekel GAJ, Kerkhofs CHH, Hilbrands LB. Treatment satisfaction in renal transplant patients taking tacrolimus once daily. Clin Ther. (2013) 35(11):1821–9.e1. doi: 10.1016/j.clinthera.2013.09.014
151. Pallet N, Etienne I, Buchler M, Bailly E, Hurault de Ligny B, Choukroun G, et al. Long-term clinical impact of adaptation of initial tacrolimus dosing to CYP3A5 genotype. Am J Transplant. (2016) 16(9):2670–5. doi: 10.1111/ajt.13788
152. Mourad M, Wallemacq P, de Meyer M, Brandt D, Van Kerkhove V, Malaise J, et al. The influence of genetic polymorphisms of cytochrome P450 3A5 and ABCB1 on starting dose- and weight-standardized tacrolimus trough concentrations after kidney transplantation in relation to renal function. Clin Chem Lab Med. (2006) 44(10):1192–8. doi: 10.1515/CCLM.2006.229
153. Størset E, Hole K, Midtvedt K, Bergan S, Molden E, Åsberg A. The CYP3A biomarker 4β-hydroxycholesterol does not improve tacrolimus dose predictions early after kidney transplantation. Br J Clin Pharmacol. (2017) 83(7):1457–65. doi: 10.1111/bcp.13248
154. Brooks E, Tett SE, Isbel NM, Staatz CE. Population pharmacokinetic modelling and Bayesian estimation of tacrolimus exposure: is this clinically useful for dosage prediction yet? Clin Pharmacokinet. (2016) 55(11):1295–335. doi: 10.1007/s40262-016-0396-1
155. Woillard J-B, de Winter BCM, Kamar N, Marquet P, Rostaing L, Rousseau A. Population pharmacokinetic model and Bayesian estimator for two tacrolimus formulations–twice daily prograf and once daily advagraf. Br J Clin Pharmacol. (2011) 71(3):391–402. doi: 10.1111/j.1365-2125.2010.03837.x
156. Rančić N, Dragojević-Simić V, Vavić N, Kovačević A, Šegrt Z, Drašković-Pavlović B, et al. Tacrolimus concentration/dose ratio as a therapeutic drug monitoring strategy: the influence of gender and comedication. Vojnosanit Pregl. (2015) 72(9):813–22. doi: 10.2298/VSP140905005R
157. Thölking G, Schmidt C, Koch R, Schuette-Nuetgen K, Pabst D, Wolters H, et al. Influence of tacrolimus metabolism rate on BKV infection after kidney transplantation. Sci Rep. (2016) 6(1):32273. doi: 10.1038/srep32273
158. Jouve T, Fonrose X, Noble J, Janbon B, Fiard G, Malvezzi P, et al. The TOMATO study (tacrolimus metabolization in kidney transplantation): impact of the concentration-dose ratio on death-censored graft survival. Transplantation. (2020) 104(6):1263–71. doi: 10.1097/TP.0000000000002920
159. Thölking G, Filensky B, Jehn U, Schütte-Nütgen K, Koch R, Kurschat C, et al. Increased renal function decline in fast metabolizers using extended-release tacrolimus after kidney transplantation. Sci Rep. (2021) 11(1):15606. doi: 10.1038/s41598-021-95201-5
160. Ro H, Jeong JC, Kong JM, Min JW, Park SK, Lee J, et al. The tacrolimus metabolism affect post-transplant outcome mediating acute rejection and delayed graft function: analysis from Korean organ transplantation registry data. Transpl Int. (2021) 34(1):163–74. doi: 10.1111/tri.13777
161. Egeland EJ, Reisaeter AV, Robertsen I, Midtvedt K, Strøm EH, Holdaas H, et al. High tacrolimus clearance—a risk factor for development of interstitial fibrosis and tubular atrophy in the transplanted kidney: a retrospective single-center cohort study. Transpl Int. (2019) 32(3):257–69. doi: 10.1111/tri.13356
162. Chamoun B, Torres IB, Gabaldón A, Sellarés J, Perelló M, Castellá E, et al. Progression of interstitial fibrosis and tubular atrophy in low immunological risk renal transplants monitored by sequential surveillance biopsies: the influence of TAC exposure and metabolism. J Clin Med. (2021) 10(1):141. doi: 10.3390/jcm10010141
163. Tomizawa M, Hori S, Inoue K, Nishimura N, Nakai Y, Miyake M, et al. A low tacrolimus concentration-to-dose ratio increases calcineurin inhibitor nephrotoxicity and cytomegalovirus infection risks in kidney transplant recipients: a single-center study in Japan. Transplant Proc. (2023) 55(1):109–15. doi: 10.1016/j.transproceed.2022.12.004
164. Jehn U, Wiedmer N, Boeckel GR, Pavenstädt H, Thölking G, Reuter S. Fast tacrolimus metabolism does not promote post-transplant diabetes Mellitus after kidney transplantation. Int J Mol Sci. (2022) 23(16):9131. doi: 10.3390/ijms23169131
165. Thölking G, Schulte C, Jehn U, Schütte-Nütgen K, Pavenstädt H, Suwelack B, et al. The tacrolimus metabolism rate and dyslipidemia after kidney transplantation. J Clin Med. (2021) 10(14):3066. doi: 10.3390/jcm10143066
166. Thölking G, Schuette-Nuetgen K, Vogl T, Dobrindt U, Kahl BC, Brand M, et al. Male kidney allograft recipients at risk for urinary tract infection? PLoS One. (2017) 12(11):e0188262. doi: 10.1371/journal.pone.0188262
167. Kuypers DRJ, Naesens M, de Jonge H, Lerut E, Verbeke K, Vanrenterghem Y. Tacrolimus dose requirements and CYP3A5 genotype and the development of calcineurin inhibitor-associated nephrotoxicity in renal allograft recipients. Ther Drug Monit. (2010) 32(4):394–404. doi: 10.1097/FTD.0b013e3181e06818
168. Genvigir FD, Salgado PC, Felipe CR, Luo EY, Alves C, Cerda A, et al. Influence of the CYP3A4/5 genetic score and ABCB1 polymorphisms on tacrolimus exposure and renal function in Brazilian kidney transplant patients. Pharmacogenet Genomics. (2016) 26(10):462–72. doi: 10.1097/FPC.0000000000000237
169. Hu R, Barratt DT, Coller JK, Sallustio BC, Somogyi AA. CYP3A5*3 and ABCB1 61AG significantly influence dose-adjusted trough blood tacrolimus concentrations in the first three months post-kidney transplantation. Basic Clin Pharmacol Toxicol. (2018) 123(3):320–6. doi: 10.1111/bcpt.13016
170. Stefanović N, Veličković-Radovanović R, Danković K, Pavlović I, Catić-Đorđević A, Bašić J, et al. Effect of the interrelation between CYP3A5 genotype, concentration/dose ratio and intrapatient variability of tacrolimus on kidney graft function: Monte Carlo simulation approach. Pharmaceutics. (2021) 13(11):1970. doi: 10.3390/pharmaceutics13111970
171. Nowicka M, Górska M, Nowicka Z, Edyko K, Edyko P, Wiślicki S, et al. Tacrolimus: influence of the posttransplant concentration/dose ratio on kidney graft function in a two-year follow-up. Kidney Blood Press Res. (2019) 44(5):1075–88. doi: 10.1159/000502290
172. Bartmann I, Schütte-Nütgen K, Suwelack B, Reuter S. Early postoperative calculation of the tacrolimus concentration-to-dose ratio does not predict outcomes after kidney transplantation. Transpl Int. (2020) 33(6):689–91. doi: 10.1111/tri.13605
173. Thölking G, Gillhaus NH, Schütte-Nütgen K, Pavenstädt H, Koch R, Suwelack B, et al. Conversion to everolimus was beneficial and safe for fast and slow tacrolimus metabolizers after renal transplantation. J Clin Med. (2020) 9(2):328. doi: 10.3390/jcm9020328
174. Pérez-Sáez MJ, Yu B, Uffing A, Murakami N, Borges TJ, Azzi J, et al. Conversion from tacrolimus to belatacept improves renal function in kidney transplant patients with chronic vascular lesions in allograft biopsy. Clin Kidney J. (2019) 12(4):586–91. doi: 10.1093/ckj/sfy115
175. Wojciechowski D, Chandran S, Vincenti F. Early post-transplant conversion from tacrolimus to belatacept for prolonged delayed graft function improves renal function in kidney transplant recipients. Clin Transplant. (2017) 31(5):e12930. doi: 10.1111/ctr.12930
176. Suwelack B, Bunnapradist S, Meier-Kriesche U, Stevens DR, Procaccianti C, Morganti R, et al. Effect of concentration/dose ratio in de novo kidney transplant recipients receiving LCP-tacrolimus or immediate-release tacrolimus: post hoc analysis of a phase 3 clinical trial. Ann Transplant. (2020) 25:e923278. doi: 10.12659/AOT.923278
Keywords: tacrolimus, tacrolimus pharmacokinetics, tacrolimus formulation, tacrolimus metabolism, kidney transplantation, renal transplantation
Citation: Henkel L, Jehn U, Thölking G and Reuter S (2023) Tacrolimus—why pharmacokinetics matter in the clinic. Front. Transplant. 2:1160752. doi: 10.3389/frtra.2023.1160752
Received: 7 February 2023; Accepted: 7 August 2023;
Published: 21 August 2023.
Edited by:
Asha B. Pillai, University of Miami, United StatesReviewed by:
Gaurav Gupta, Virginia Commonwealth University, United StatesJacek Rubik, Children’s Memorial Health Institute (IPCZD), Poland
© 2023 Henkel, Jehn, Thölking and Reuter. This is an open-access article distributed under the terms of the Creative Commons Attribution License (CC BY). The use, distribution or reproduction in other forums is permitted, provided the original author(s) and the copyright owner(s) are credited and that the original publication in this journal is cited, in accordance with accepted academic practice. No use, distribution or reproduction is permitted which does not comply with these terms.
*Correspondence: Stefan Reuter c3JldXRlckB1bmktbXVlbnN0ZXIuZGU=