- 1Department of Surgery, Center for Engineering in Medicine and Surgery, Massachusetts General Hospital, Harvard Medical School, Boston, MA, United States
- 2Research Department, Shriners Children’s Boston, Boston, MA, United States
- 3Department of Surgery, University of Minnesota, Minneapolis, MN, United States
- 4Departments of Mechanical and Biomedical Engineering, University of Minnesota, Minneapolis, MN, United States
Organ transplantation remains the only treatment option for patients with end-stage organ failure. The last decade has seen a flurry of activity in improving organ preservation technologies, which promise to increase utilization in a dramatic fashion. They also bring the promise of extending the preservation duration significantly, which opens the doors to sharing organs across local and international boundaries and transforms the field. In this work, we review the recent literature on machine perfusion of livers across various protocols in development and clinical use, in the context of extending the preservation duration. We then review the next generation of technologies that have the potential to further extend the limits and open the door to banking organs, including supercooling, partial freezing, and nanowarming, and outline the opportunities arising in the field for researchers in the short and long term.
1. Introduction
Organ transplantation remains the only treatment option for patients with end-stage organ failure. If combined, the number of deaths due to organ failure exceeds cancer and all other causes (1). The discrepancy between patient need and the number of organs available results in a transplant waiting list of over 100,000 in the United States alone, which is generally considered the tip of the iceberg for those in need. Geographic differences in organ availability and other factors further lead to inequity in access to transplant medicine (2). Although there has been rapid growth in organ transplantation since 2013, and a record number of total solid organ transplants were performed in 2020, the need for more transplantable grafts remains desperately high (2, 3).
Clinical transplantation began with the pioneering work of Joseph Murray, who performed the first successful organ transplantation in 1954 (Figure 1A) (4). In 1967, Thomas Starzl performed the first successful liver transplantation under immunosuppression, with survival exceeding 1 year (Figure 1B) (5). Once a solution for immune rejection was identified, preserving organs in a viable, transplantable condition for an extended period became the key technological barrier for providing access to this life-saving treatment (1). As noted by Southard and Belzer, who developed the University of Wisconsin organ preservation solution, preservation technology is “the supply line for organ transplantation” (6). In its current state, organ transplants can be described as a unique supply chain management problem, where the stakes are simply the lives of the patients.
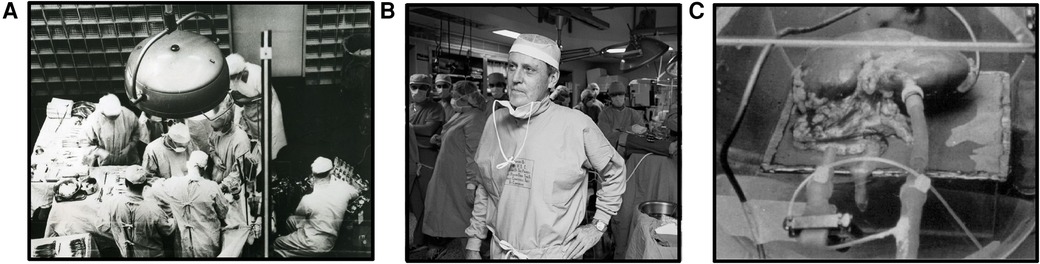
Figure 1. The evolution of machine perfusion. (A) Dr. Joseph Murray, while performing the first successful organ transplantation, 1954 (113). (B) Dr. Thomas Starzl, after performing a transplant surgery (115). (C) Dr. Folkert Belzer's kidney perfusion machine (116).
In the 1960s, Belzer developed the first hypothermic machine perfusion (HMP) device that was clinically applicable (Figure 1C) and performed the first HMP-preserved human kidney transplantation in 1967 (7). However, this method was considered expensive and difficult to implement at the time. In 1969, Collins et al. started the era of cold storage by demonstrating the successful transplantation of canine kidneys after storage in a small box with ice for 30 h (8). The introduction of the University of Wisconsin solution in the 1980s by Belzer and Southard represented a breakthrough in organ preservation (9). Static cold storage (SCS) replaced machine perfusion and became the clinical gold standard, enabling organ transplantation to be a vast success that can reach very high success rates—unlike those early days when it was seen as a last resort.
The last decade has seen a flurry of activity in improving organ-preservation technologies (Figure 2): machine perfusion, abandoned in the 1980s due to cost and practicality concerns, has made a dramatic comeback and is now permeating into the operating rooms (ORs) rapidly (10, 11). In parallel, there is a convergent arch of major technological development in the field of cryobiology, which was long limited to cells and reproductive medicine, now offering many innovative alternatives for long-term organ preservation in subzero temperatures. Perhaps, surprisingly, these new cryobiology approaches critically leverage machine perfusion as a platform technology and build on its success to enable these next-generation methods. In this review, we present a brief update on the developments, achievements, and innovations that have taken place in the field of extended organ preservation over approximately the last 10 years. To make the task tractable, we focus primarily on liver preservation. However, we have allowed limited side notes to other organ systems where horizontal translation of technologies appears obvious, where appropriate.
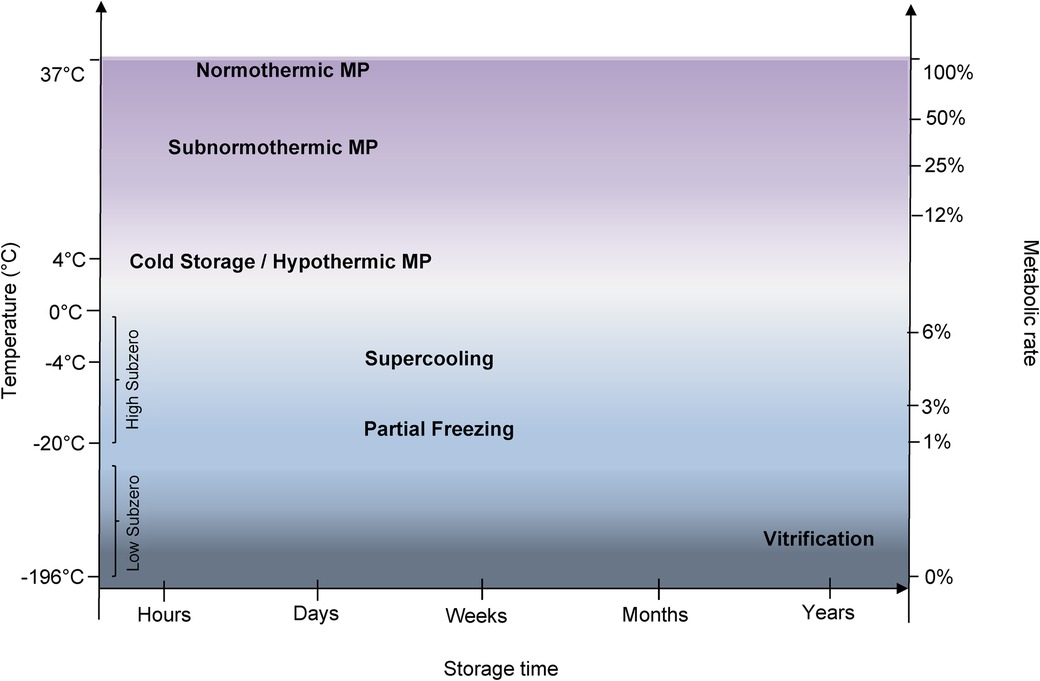
Figure 2. Key organ preservation techniques and the interplay of temperature and metabolic rates. Metabolic rates are estimated based on the van't Hoff rule, which estimates the metabolic rate approximately doubles with every 10°C increase in temperature (115).
2. Static cold storage
Cold storage has been an enabling technology that has allowed transplantation to be an accessible treatment for organ failure. Even though this method is very practical and cost-effective, it is insufficient for the following three key reasons: (1) the organs actively suffocate during storage, and even the high-quality organs can only be stored for a few hours (12); (2) non-ideal donors organs, particularly extended criteria donors (ECDs), have a higher sensitivity to cold ischemia (13) exacerbating the ischemia-reperfusion injury (IRI) (14). Briefly, when the organs are deprived of oxygen, mitochondria function and adenosine triphosphate levels decrease, resulting in cellular swelling and apoptosis. After organs are reperfused, cell injury is aggravated by triggering the formation of reactive oxygen species (ROS) and immune response, which is associated with delayed graft function and primary graft failure (15). In practice, this means that marginal organs often present a higher risk of failure, and many of these are not used; (3) since the organ is not fully functional at ice-cold temperatures, it is difficult to assess if it will function as expected. To avoid the risk of primary non-function, population statistics-based approaches, such as the Donor Risk Index, are used to select higher-quality donor organs. But, by their nature, these scoring systems are conservative, and many potentially transplantable organs are not used (16). These disadvantages led to the development of alternative preservation techniques, leading to the rise of an old, almost forgotten process that Murray himself had actually used.
3. Oxygenated machine perfusion
As the number of ideal organs is insufficient to meet the transplant demand, marginal organs, particularly those from donors after cardiac death (DCD), have become one approach to expand the donor organ pool. For DCD organs, the method for preservation is more important than heart-beating donor organs [donors after brain death (DBD)] because there is already an existing level of warm ischemic injury and a higher risk for graft malfunction (17). Machine perfusion continuously provides oxygen and nutrients, which enables the graft to restore tissue energy (18), thus allowing a certain amount of repair and recovery of an ischemic organ ex situ before transplantation (19). As a functional preservation modality, it also allows full organ functional assessment, in contrast to SCS, which effectively only slows the rate of ischemic injury. The operation temperature of machine perfusion can be simplistically divided into three main categories: HMP at 4°C–10°C; subnormothermic machine perfusion (SNMP; also referred to as mid-thermic perfusion) at 20°C–33°C; and normothermic machine perfusion (NMP) at 36°C–38°C, depending on the species used in the study (a more rigorous breakdown including recommendations for standardized terminology can be found in Karangwa et al. (20)). Since perfusion is synergistic with all extended preservation approaches, and itself allows significant opportunities to extend preservation beyond the current clinical limits, we first summarize key recent studies in liver machine perfusion (Table 1).
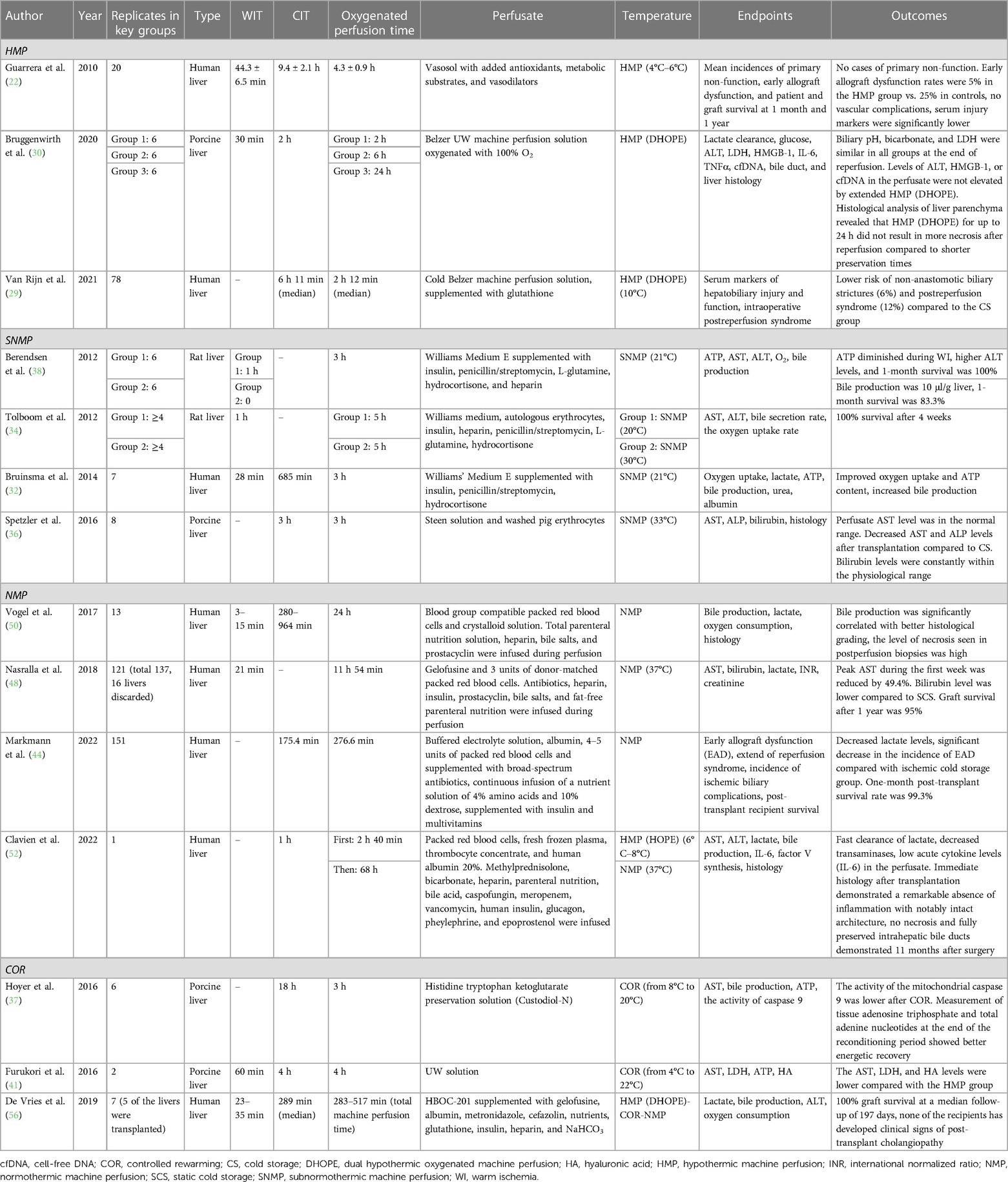
Table 1. A brief summary of key studies in liver perfusion categorized based on operating temperatures.
3.1. Hypothermic machine perfusion
Notably, there are multiple liver perfusion protocols that employ hypothermic temperatures and oxygenation; for simplicity in presentation, we refer to all of these as HMP, with brief comments to their differences where needed.
HMP relies on decreased cellular metabolism at lower temperatures (4°C–10°C). HMP of the kidneys, but without oxygenation, has become standard practice in kidney perfusion, but with limited benefits compared to SCS (21). Guarrera et al. reported the first successful human liver transplantation after HMP (22), without active oxygenation. With HMP protocols featuring active oxygenation, studies have shown that despite lower temperatures, HMP helps organs to restore ATP levels (23), leading to reduced post-transplant complications (24). A similar study where DCD livers were treated with a short period of dual HMP (termed DHOPE) at the end of the ischemic period showed a reduced IRI of the biliary tree (25). Short-term (2 h) end-ischemic oxygenated HMP after SCS has also been shown to result in better endothelial cell function of ECD livers compared to SCS preservation alone (26). Clinical trials have shown superior transplantation outcomes of DCD livers after end-ischemic HMP (HOPE) compared to untreated DCD and DBD liver transplants: Schlegel et al. demonstrated higher 5-year graft survival (27), and Mueller et al. demonstrated reduced cancer recurrence in liver recipients who presented with hepatocellular cancer before the transplant (28). A key randomized controlled trial of HMP for DCD livers has shown a lower risk of non-anastomotic biliary strictures compared with SCS (6% in the machine-perfusion group vs. 18% in the control group) (29).
In terms of using HMP to extend the duration of preservation, one study tested HMP (DHOPE) for up to 24 h with success (30). The viability assessment at 24 h of HMP (DHOPE) was similar to the assessment of livers perfused for 2 and 6 h, and superior to 24-h cold-stored livers. Overall, these studies clearly demonstrated the utility of HMP and its superiority to SCS in most cases. The fact that HMP allowed ATP recovery of the grafts was somewhat surprising given the low temperature, where mitochondrial activity was assumed to be minimal at best. Indeed, many investigators explored a selection of warmer temperatures to enable improved mitochondrial function.
3.2. Subnormothermic machine perfusion
SNMP offers a balance between reducing the metabolic needs of the tissue and allowing the mitochondria to work and replenish ATP stores (31, 32). Another reason behind the development of SNMP has been operational simplicity. At normothermic temperatures, an oxygen carrier is required to meet the oxygen demands of the tissue, whereas at 21°C, supraphysiological levels of oxygenation suffice (33). Further, SNMP eliminates the need for a secondary dialysis circuit that is necessary for NMP to provide additional nutritional supply and removal of liver by-products (34). Multiple studies using animal or human liver models suggest that SNMP can positively affect liver viability and predict post-transplantation graft function (35–37). SNMP has also been shown to be equal to NMP in terms of allowing resuscitation of 1-h warm ischemic rat livers to transplantability (34, 38). A more thorough metabolomic analysis has shown that SNMP is superior in terms of faster recovery of ATP levels to nominal compared to NMP, but at the cost of somewhat increased oxidative stress, as indicated by depletion of glutathione and its precursors (39, 40).
NMP has specifically been tested to extend the preservation duration in an end-cold-storage mode (12), where it was shown to allow the doubling of total preservation time for rat livers (to 48 h) with 100% transplant success. SNMP of discarded human livers after a duration of cold ischemia resulted in remarkable improvement in liver viability factors (32). Another SNMP study demonstrated lower AST, LDH, and HA levels than HMP controls in perfused porcine livers (41).
3.3. Normothermic machine perfusion
At the most basic level, NMP aims to mimic physiological conditions precisely by maintaining body temperature (36°C–38°C), providing essential substrates for cellular metabolism—oxygen and nutrition (42)—and avoiding the cold ischemia, which, for marginal grafts, leads to an increased risk of graft failure and post-transplant mortality (43, 44). An additional benefit is that the organ is fully functional, and various markers can be readily measured and easily compared to in vivo function levels to gauge graft viability before transplantation (45, 46). NMP also allows pharmacological options on grafts to enhance graft repair (47), whereas, with HMP and SNMP, the reduced activity may render such pharmacologic compounds inactive. The first key randomized controlled trial demonstrated successful 12 h NMP for DCD livers: NMP DCD livers showed superior outcome data compared to DCD and DBD livers preserved with SCS (48). In another recent multicenter randomized controlled clinical trial, livers were preserved by either ischemic cold storage or NMP; the results showed remarkably better clinical outcomes and a higher use of DCD livers for transplantation in the NMP group (51% in the NMP group vs. 26% in the SCS group) (44).
NMP itself has shown significant potential in extending preservation durations. Even in the first clinical trials, the participating surgeons indicated they felt comfortable leaving the graft on the pump for a few more hours to better plan the surgeries (48). Studies have shown human livers successfully preserved at normal metabolic rates for 24 h (49, 50), with one preclinical study showing maintenance in a functional state for up to 1 week as assessed by bile production, ATP levels, and synthesis of blood proteins (51). A recent single clinical case by Clavien et al. showed long-term NMP for 68 h with successful transplantation, three times longer than in previous studies (52).
The concerns with NMP are that, at 37°C, the grafts are going at “full speed”, and, as sophisticated as an NMP system is, it does not capture and recapitulate the entire human body. Therefore, one concern is that extended NMP may exacerbate any shortcomings of the perfusion system. Other concerns include cost and lack of failsafe options should a pump failure occur, which has been one motivating factor behind controlled rewarming (COR).
3.4. Controlled rewarming and mixed perfusion modalities
Given that each temperature seems to have its advantages and drawbacks, one alternative approach is to use a combination therapy to prepare the graft more gently for transplant. COR aims to achieve this by starting the perfusion with SCS or HMP and linearly increasing the temperature to NMP. A key advantage is that during the initial cold preservation, a more straightforward protocol and the use of a device that is likely less costly and effort-intensive and minimizes the risk of technical issues with the pump. Moreover, should the pump fail, the fallback is SCS, which will occur in the same container without any user intervention.
Assuming everything goes as expected during the cold period, where the transportation of the graft to the recipient site is completed, the temperature is gently increased, presumably striking a balance similar to SNMP, but ramping up to NMP, where the graft can be easily assessed and is prepared for function in vivo. Indeed, better hepatic functions and increased bile production are achieved with a period of COR after the initial HMP (53). In one study, COR was compared with NMP for the resuscitation of liver grafts after cold storage. COR resulted in better energetic recovery and a higher bile production (37). Hoyer et al. demonstrated successful transplantation of human livers after COR with a 100% post-transplant survival rate at 1 and 3 years and a 93.8% survival rate at 5 years (54).
A similar approach is to sequence HMP and NMP. Treating DCD livers with HMP (DHOPE) before NMP has shown excellent results in several studies (23, 55). Using sequential HMP (DHOPE) and NMP, Porte et al. reported 100% graft survival after 197 days of follow-up without any post-transplant biliary complications (56). This protocol also increased the number of transplantable livers from rejected high-risk livers by 20% (57).
To our knowledge, COR or sequenced modalities have not been directly tested to extend preservation. However, sequencing static storage with a warm perfusion to recover from the cold and prepare the graft for transplantation has been the key pillar of subzero organ storage, which specifically aims to extend preservation durations.
4. Subzero organ preservation
Cryopreservation is the use of low temperatures, traditionally defined as −80°C (solid carbon dioxide) or −196°C (liquid nitrogen), to preserve structurally intact biological systems for periods in the order of years (58). It is an enabling technology with many medical applications, such as bone marrow transplantation, blood transfusion, artificial insemination, and in vitro fertilization (59).
When it comes to applying traditional cryopreservation to vital organs, there are a variety of challenges that the field has struggled with for decades. When large (up to 2–3 L of volume in the case of the liver) and three-dimensional structures are cooled below freezing point, ice can immediately form (60). This ice directly damages cells, as intracellular formation can rupture cells and extracellular formation can cause severe mechanical stress (61). The standard approaches to mitigate cryoinjury include the following: (1) the use of cryoprotectants (e.g., DMSO, glycerol, sorbitol, 2,3-butanediol) that modulate the thermodynamic properties of the sample (freezing point depression, altering the type of ice that forms, dehydration of cells before freezing, etc.) (59); and (2) careful tuning of cooling and rewarming rates to allow some spatiotemporal control of ice formation (62). Cooling too fast can cause insufficient dehydration of cells and increases the risk of lethal ice formation (63), whereas cooling too slowly means an extended period of ischemic injury. Similarly, for the re-warming stage, rapid thawing at 37°C–40°C is considered crucial (64) to prevent ice reformation and other mechanisms of damage to the cells. However, there have been few, if any, successes in scaling up traditional cryopreservation—which we define as any method that involves allowing ice formation in the range of −80°C to −196°C.
Recent research efforts have taken very different paths, either avoiding ice altogether or aiming to achieve temporal control using novel nature-inspired techniques. For the purposes of clarity in presentation, we will delineate the approaches into two categories (Figure 2): high subzero, meaning the range of −4°C to −30°C and covering supercooling, isochoric, and partial freezing; and low subzero storage, meaning vitrification at −196°C, particularly involving nanowarming. Table 2 depicts key developments in this new field. Table 3, in turn, provides an extended summary of all key extended preservation studies, including both subzero preservation and machine perfusion. It should be noted that the technological readiness level of the approaches described here are at a much earlier stage compared to machine perfusion overall, with only one of them having been scaled to human livers already. In a few cases, the initial development efforts have focused on kidneys, but we have opted to include them in this discussion as efforts in translation to livers have already been described.
4.1. High subzero storage
4.1.1. Supercooling
Ice nucleation is a stochastic event; under atmospheric pressure, the probability of ice nucleation becomes nonzero at 0°C and increases as the temperature is reduced. This means it is possible for biological systems to stay below the freezing point, which is called supercooling. Contrary to expectations, supercooling just below the freezing point can be remarkably stable. Indeed, supercooling is employed as a preservation technique by multiple animals in nature, including one crocodile. Still, the poster child is the arctic ground squirrel (65), which is known to have core temperatures of −8°C in a supercooled state. Given that every 10°C drop in temperature roughly halves the metabolic rates, a doubling of preservation time is relatively easy by reducing the storage temperature from ice-cold (2°C–8°C effective in the organ depending on the point of measurement) to a relatively easy to attain −5°C, while avoiding the complications and injuries induced by phase change (66).
The basic idea of utilizing supercooling in the transplantation field goes back at least to the 1960s (67). However, it appears to have been abandoned in the transplantation field since the advent of SCS. Recent developments, however, demonstrated that supercooling improved the preservation of primary hepatocytes (68), which was then scaled to rat livers with this success (69): The investigators demonstrated that SCS allowed only 24 h of storage of rat livers with transplant success, with 100% survival at 30 days. At 2 days, success fell to only 50%; at 3 days or longer, there was no survival. Machine perfusion alone was shown to double preservation to 2 days with excellent success but failed to push beyond (0% survival at 3 days) (12). The tandem use of subzero storage at −6°C, machine perfusion (SNMP), and two select preservative agents 3-O-methyl glucose (3-OMG) (70) and polyethylene glycol (PEG) have allowed transplantations with success at 3 days, with 4 days supercooled storage possible but a reduction in survival (69). The technique was then scaled up to whole human livers in a preclinical study, with success demonstrated when five human livers were preserved ice-free for 27 h at −4°C and followed by SNMP (71, 72). Given that organs only rejected for transplant are available for research, viability was tested using a matched non-inferiority trial comparing the state of the livers before and after as assessed during SNMP, followed by a simulated transplant step including reperfusion with reconstituted whole blood. The key changes in the human protocol are additional cryoprotective agents (CPAs) of glycerol and trehalose to enable stable, non-frozen storage and a multi-temperature perfusion protocol to enable homogenous loading of CPAs and their safe removal before rewarming (72).
As supercooling is not an asymptotically stable state by thermodynamic standards, it is often assumed that there is a significant risk of ice formation in the organ over the durations of storage practical for transplantation. In an elegant study, Huang et al. demonstrated that the risk of freezing is effectively zero so long as the heterogenous nucleation is prevented by eliminating air/liquid interfaces that induce ice formation (73). In this work, the air/water interface was eliminated using an oil sealant such that a large volume of water (100 ml) can be kept at −16°C for up to 100 days without freezing, and, similarly, red blood cells in 30-ml suspensions can be readily preserved at −13°C for up to 365 days. The same approach has also succeeded in preserving adipose-derived stem cells at −13°C and −16°C (74). This approach, dubbed deep supercooling, comes close to eliminating heterogeneous nucleation and leaves only homogenous nucleation as a possible risk for ice formation. Notably, the homogenous freezing temperature of water, i.e., the point where it becomes thermodynamically feasible for H2O molecules to form crystals in the absence of a catalyst such as an air–liquid interface, is −20°C (75), which therefore is the actual thermodynamic limit of supercooled storage.
Of the subzero approaches, supercooling is the most advanced in terms of technological readiness, with success in preclinical human studies already demonstrated. The key advantage it possesses is the absence of a need to deal with the consequences of phase change, be it glass or ice. Similarly, the CPAs used are very benign and are required in low concentrations compared to many alternatives. In terms of equipment, it is equivalent to a standard perfusion pump plus one chiller, which is technically not difficult to combine. All these advantages make it of higher value from a translational perspective, but avoiding phase change also limits the attainable preservation duration.
4.1.2. Partial freezing
While the homogenous nucleation temperature thus limits supercooling, many organisms in nature can handle ice formation and use it as a method of survival over harsh winters. The classic example of this approach is the wood frog, Rana Sylvatica (76, 77), which is known to survive over 200 days in a frozen state in lab conditions. A key aspect is that the ice does not occur randomly, but its formation is carefully controlled both spatially and temporally; the result is that the animal's blood is completely frozen, but the parenchyma remains in a non-frozen subzero state similar to supercooling. In a study using a microfluidic model of capillaries, a similar spatiotemporal control was first shown to be possible using Snomax, a well-known ice-inducer that is used to create artificial snow for ski resorts (78). Leveraging these findings, the same group demonstrated the successful partial freezing of rodent livers for 5 days at temperatures as low as −15°C (79). The study further demonstrated that the main limitation was the freezing-thawing process, and it may be possible to extend the storage duration further to reach that achieved by Rana Sylvatica. Extrapolating from the numbers observed in nature, partial freezing has the potential to further push the limits of high subzero preservation from weeks to months. However, the phase change still causes some injury to the organs, as suggested by elevated ALT levels, and further improvements are needed to scale to human organs.
4.1.3. Isochoric preservation
Isochoric (constant volume) preservation is a novel approach aiming to utilize pressure as an additional variable to optimize preservation conditions. The process involves placing the biological sample suspended in preservation media within a pressure chamber and inducing ice formation at the periphery of the chamber (80). Since the molar volume of ice is larger than liquid water, the ice expands and increases the pressure of the liquid trapped in the chamber, which depresses the freezing point for the remaining liquid (specimen) and allows storage at lower temperatures in a non-frozen state without the need of additional, potentially toxic, CPAs (81). Initial studies with rat hearts have shown preservation under isochoric conditions at −8°C (78 MPa) in a UW solution for 1 h without additional CPAs (82). And in cardiac micro physiological systems, the use of isochoric conditions has been shown to increase the stability of supercooling by preventing the system to access air-water interfaces and suppressing density fluctuations (83). While there is much work to be done in terms of scaling towards transplantation, and success has not yet been shown in models of liver transplantation, the fundamental idea of controlling pressure and temperature makes intuitive and thermodynamic sense and can enable entirely new applications and technologies.
4.2. Low subzero storage
4.2.1. Vitrification
Under cryogenic conditions (defined as temperatures <−153°C), metabolic activity effectively ceases, and the storage of biologic material is theoretically indefinite (84). The application of this ultralow temperature storage of biomaterials was conceptualized by Boyle in the 17th century when trying to preserve human bodies and animals (85). Still, it was not successfully achieved until the 1940s when Polge, Smith, and Parkes demonstrated that the addition of CPAs, such as glycerol, could protect cells at ultralow temperatures and enable the successful cryopreservation and recovery of spermatozoa (86). Unfortunately, those conventional cryopreservation techniques failed with larger specimens, such as tissues and organs, due to the destructive formation of ice crystals that kills cells (87) and disrupts the complex architecture of whole organs (88, 89).
Vitrification, or rapid cooling to a glass-like state, offers a promising alternative to conventional cryopreservation (88). The biomaterial is cooled at a rate that is too fast for the phase transition from liquid to crystalline ice to occur. Basel Luyet is generally credited with conceptualizing this approach in the 1930s (90), but the first successful demonstration occurred with erythrocytes in the 1960s (91). In the vitrified state, the biomaterial is an amorphous solid and can be cryogenically stored without any destructive ice formation. The first major biological and physical success of vitrification and rewarming was achieved with mouse embryos in the 1980s (92), thereby opening up the field of assisted reproductive medicine. The extension of vitrification to organs was shown by Fahy and colleagues in the mid-1980s, when they showed that rabbit kidneys that had been perfused with sufficiently concentrated CPA solutions could be cooled to an ice-free vitrified state. Vitrification of other organs, including rat kidneys (93), hearts (94, 95), livers (96), porcine blood vessels (97), and ovaries (98), has also been demonstrated more recently.
However, rewarming vitrified organs while preserving viability and function remains challenging. To rewarm them, the rate of warming must be fast enough to avoid ice formation during warming. That rate, the critical warming rate (CWR), is always greater than the critical cooling rate (CCR; the rate to achieve vitrification), which is already incredibly high for physiologic solutions (>107°C/min) which cannot be reached for any but the smallest size specimens (99). Fortunately, CPA cocktails have been developed that reduce the CWR to achievable values (100, 101). However, these CPAs can be toxic to cells and tissues. Thus, there is a critical balance between achieving sufficient CPA concentration in tissue and avoiding the toxicity of those agents. The equilibration of CPA in the tissue or organ increases the CPA exposure time, which can be toxic to cells (102). Combining multiple CPAs with similar thermodynamic effects but different underlying toxicity mechanisms, or lowering the CPA exposure temperature, are options commonly pursued to reduce the toxicity.
Further, the rewarming of organs must be uniform. Boundary heating, such as convection during immersion in a water bath, fails in organs and other larger biomaterials. This is because the temperature gradient from the warmed surface to the relatively cooler core creates significant thermal stresses that can fracture the organ system. Thus, successful rewarming of vitrified organs must be fast (to avoid ice) and uniform (to prevent cracking or fracture)—two challenges that are difficult to achieve in combination.
4.2.2. Nanowarming
Several approaches to rewarming vitrified organs have been attempted, including convective warming (103), dielectric heating (104), and even microwave heating (105–107). However, these have yet to achieve sufficiently rapid and uniform heating to restore full function in samples greater than several milliliters in size. To overcome these limits, a new electromagnetic approach called “nanowarming” was developed (108) and deployed on an 80-ml system (97). It was then generalized to the kidneys (and later other organs) that could be perfused with CPA and biocompatible iron oxide nanoparticles (109) and then heated (93). The CPA diffuses throughout the tissue, but the nanoparticles remain intravascular. The organs are then rapidly cooled to a vitrified state and stored at an ultralow temperature (i.e., −150°C) until needed. To rewarm them, the organs are transferred to a radiofrequency (RF) coil that induces heat generation through hysteresis losses from oscillating magnetic dipoles in the nanoparticles. Rewarming is both rapid and uniform.
Since RF waves penetrate biomaterials efficiently, and the nanoparticles are distributed throughout the vasculature, the process of nanowarming is essentially independent of system size and can scale from rodent to human organs. The physical success of this approach has been demonstrated for the blood vessels, heart, kidney, and liver in animal models (93–97). However, future work will be needed to minimize CPA toxicity for improved organ function and scaling various aspects of the protocols to human organ size.
5. Discussion
In many aspects, transplantation is a story of moving from one extreme to another. The starkest contrast is the extremes found between death due to organ failure and a decade or more of life a transplant can provide. The immunosuppression required enables transplant but increases the risk of cancer and infection. The preservation methods have oscillated between warm and cold and warm again, and as this review of recent developments indicates, the next-generation techniques are evidently returning to cold.
Prolonged ex vivo machine liver perfusion has been explored clinically, with one major study showing success with up to 3 days (52), and prior clinical experience also suggests using it to optimize scheduling. A storage time of 3 days itself is a major improvement, which could transform the current clinical practice by making transplant an elective surgery. As to the type of perfusion modality, it is difficult to suggest the best approach, since there are very few detailed comparative studies. Each temperature has its advantages and challenges; NMP keeps the livers fully functional and allows the most straightforward way for viability assessment before transplant. The low temperature of HMP provides low metabolic rates with decreasing cellular metabolic need. SNMP, as well as COR, offer a balance between these two approaches, and there appears to be some evidence suggesting that a gentler approach may be the ideal option. Rigorous studies comparing these alternatives, ideally in a clinically relevant large animal model, could answer many key questions and inform the field. On the other hand, it is unclear who would fund such a comparative study. The end result may be that each transplant center will have its preferred protocol, likely based on the cost aspects, and the comparison will have to wait until there are enough data to perform a retrospective study.
For the subzero approaches in development, the literature indicates there is a variety of techniques in development, all with promising results, with different strengths and weaknesses in terms of potential storage duration vs. complexity. The likely near-term trajectory is that these techniques will be developed towards clinical testing in order of technological readiness levels. It is possible to envision a future where there are multiple preservation techniques employed based on need: for ideal organs with a recipient in close proximity, SCS can be perfectly adequate; for organs that need repair, some form of perfusion would allow ex vivo treatment, which would further allow allocation of organs in an expanded area such as within the continental USA. For international exchanges and allocation and short-term banking in the order of months, for instance to provide back-up livers to be used in case of Primary Nonfunction (PNF) and acute liver injury, high subzero organ banking would be the solution. For very long-term banking, which would allow off-the-shelf readiness for organs, low subzero approaches would be the real solution. Isochoric approaches could either boost one of these or be completely enabling by, for instance, allowing a specific approach to be practical without the need for using toxic levels of CPA. Whether these options can simultaneously be viable commercially will depend on cost, practicality, and efficacy, which are yet to be determined.
For the clinical translation of the novel techniques described here, the economics of the technology are a key aspect of widespread adoption. Cold storage is simple and cheap compared to the cost of the graft, not to mention the total cost of the transplant procedure, including postoperative patient care. The latter component, the cost of patient care, can more than double the cost of the graft and surgical procedures, since it involves intensive care, extending significantly depending on the outcomes. When these costs are included, machine perfusion is cost-effective considering the significantly decreased rates of transplant complications, reduced length of hospital stay, and consequent benefits to the transplant waiting list (110). It can be readily envisioned that similar benefits will also be true for new techniques for organ preservation. It is too early to make a cost comparison between various subzero approaches. However, the financial structure of how those costs are paid and how risk is balanced between the organ procurement organization, transplant centers, and insurers must be determined. These structures are also likely to vary between countries with different laws, healthcare systems, and ethical and cultural views on organ transplantation. Technology, as it often does, will likely push a closer alignment of such structures globally.
The most extreme aspect of new biopreservation technologies will be their impact on our unique system for allocating scarce but lifesaving resources. The existing organ availability and allocation system is predicated upon an organ's time-limited viability when outside the body. However, as technology modifies that barrier, the system must change. Significantly prolonging ex vivo viability would permit greater flexibility with candidate identification for a transplant and better candidate preparation, permitting safer transplant procedures. There will also be “new” variables entered into the assessment of organ quality. Will medical judgment continue as the final tool to assess organ quality? Introducing complex physical and chemical organ manipulation will likely exceed clinical expertise. Oversight, required to assure public trust, will be challenged with expanded definitions for organ quality and safety. Questions about allocating organs equitably and ethically will become more prominent, and more complicated because it is rapidly becoming practical to share donor organs across countries, if not globally. The legal and ethical questions raised by the prospect of sharing organs without borders can be daunting to the extreme for some, but new allocation systems enabled by exciting new technology carry the promise of increasing access to this unique, lifesaving treatment, and a second transplant revolution.
It should be obvious that this work has its limitations. While extending the review to organs beyond the liver would have been preferrable in terms of completeness, we realized the scope grew beyond tractability, and we therefore refrained from discussing other organs with very few exceptions where translational technology transfer was obvious and provided insights. We similarly limited the scope of the review to isolated organs: NRP (111) and ECMO (112) are technologies that provide options to retain multiple organs in situ in the donor body, in a viable state for procurement at an ideal point in time. These techniques are exciting new options that would also enable addressing the unmet need for transplants and deserve their own separate review and discussion.
6. Conclusions
Recent studies have demonstrated that a variety of new strategies in preservation promises to enable longer storage times and recover marginal donor organs. Machine perfusion has the potential to be the clinical standard for transplant and is evolving rapidly before our eyes. Tomorrow will belong to next-generation preservation techniques, which may be advanced perfusion or subzero or isochoric, or something else that is brewing on a lab bench. What is clear is that transplantation is seeing the convergence of several new groundbreaking technologies, and the future will be very different from what is the current clinical standard across the world.
Author contributions
All authors contributed to the concept and design, critical review for intellectual content, and interpreting the conclusions. OSO and B-EN collected the data and visual display items presented in the tables and figures. All authors contributed to the article and approved the submitted version.
Funding
This material is partially based upon work supported by the National Science Foundation (Grant No. EEC 1941543). Support from the US National Institutes of Health is gratefully acknowledged for the following awards: R01DK114506, R01DK096075, R21GM143659, R01EB028782, R01DK117425, R01HL135046, R01DK131209, and R01DK132211.
Conflict of interest
Some authors declare competing interests. KU, JB, and MT have patent applications relevant to this study. KU and MT have a financial interest in and serve on the Scientific Advisory Board for Sylvatica Biotech Inc., a company focused on developing high subzero organ preservation technology. Competing interests for MGH investigators are managed by MGH and MGB in accordance with their conflict-of-interest policies. The remaining authors declare that the research was conducted in the absence of any commercial or financial relationships that could be construed as a potential conflict of interest. KU declares that they were an editorial board member of Frontiers, at the time of submission. This had no impact on the peer review process and the final decision.
Publisher's note
All claims expressed in this article are solely those of the authors and do not necessarily represent those of their affiliated organizations, or those of the publisher, the editors and the reviewers. Any product that may be evaluated in this article, or claim that may be made by its manufacturer, is not guaranteed or endorsed by the publisher.
Abbreviations
ALP, alkaline phosphatase; ALT, alanine aminotransferase; AST, aspartate aminotransferase; ATP, adenosine triphosphate; cfDNA, cell-free deoxyribonucleic acid; COR, controlled rewarming; CPA, cryoprotective agents; DBD, donor after brain death; DCD, donor after cardiac death; DHOPE, dual hypothermic oxygenated machine perfusion; DMSO, dimethyl sulfoxide; EAD, early allograft dysfunction; ECD, extended criteria donor; HA, hyaluronic acid; HMGB-1; high motility group box-1; HMP, hypothermic machine perfusion; HOPE, hypothermic oxygenated machine perfusion; ICG, indocyanine green; IL-6,8; interleukin-6,8; INR, international normalized ratio; IRI, ischemia-reperfusion injury; LDH, lactate dehydrogenase; MPa, megapascal; MP, machine perfusion; NMP, normothermic machine perfusion; PEG, polyethylene glycol; PVP, polyvinyipyrrolidone; ROS, reactive oxygen species; SCS, static cold storage; TNFα; tumor necrosis factorα, UW, University of Wisconsin; WI, warm ischemia; 3-OMG, 3-
References
1. Giwa S, Lewis JK, Alvarez L, Langer R, Roth AE, Church GM, et al. The promise of organ and tissue preservation to transform medicine. Nat Biotechnol. (2017) 35(6):530–42. doi: 10.1038/nbt.3889
2. A fairer and more equitable, cost-effective, and transparent system of donor organ procurement, allocation, and distribution. Available at: https://www.nationalacademies.org/our-work/a-fairer-and-more-equitable-cost-effective-and-transparent-system-of-donor-organ-procurement-allocation-and-distribution.
3. OPTN/SRTR 2018 annual data report: introduction. Am J Transplant. (2020) 20(Suppl s1):11–9. doi: 10.1111/ajt.15671
4. Tan SY, Merchant J. Joseph Murray (1919–2012): first transplant surgeon. Singapore Med J. (2019) 60(4):162–3. doi: 10.11622/smedj.2019032
5. Starzl TE, Groth CG, Brettschneider L, Penn I, Fulginiti VA, Moon JB, et al. Orthotopic homotransplantation of the human liver. Ann Surg. (1968) 168(3):392–415. doi: 10.1097/00000658-196809000-00009
6. Southard JH, Belzer FO. Organ preservation. Annu Rev Med. (1995) 46:235–47. doi: 10.1146/annurev.med.46.1.235
7. Belzer FO, Ashby BS, Gulyassy PF, Powell M. Successful seventeen-hour preservation and transplantation of human-cadaver kidney. N Engl J Med. (1968) 278(11):608–10. doi: 10.1056/NEJM196803142781108
8. Collins GM, Bravo-Shugarman M, Terasaki PI. Kidney preservation for transportation. Initial perfusion and 30 h’ ice storage. Lancet. (1969) 2(7632):1219–22. doi: 10.1016/S0140-6736(69)90753-3
9. Wahlberg JA, Southard JH, Belzer FO. Development of a cold storage solution for pancreas preservation. Cryobiology. (1986) 23(6):477–82. doi: 10.1016/0011-2240(86)90056-8
10. Salehi S, Tran K, Grayson WL. Advances in perfusion systems for solid organ preservation. Yale J Biol Med. (2018) 91(3):301–12.30258317
11. Weissenbacher A, Vrakas G, Nasralla D, Ceresa CDL. The future of organ perfusion and re-conditioning. Transpl Int. (2019) 32(6):586–97. doi: 10.1111/tri.13441
12. Bruinsma BG, Berendsen TA, Izamis ML, Yarmush ML, Uygun K. Determination and extension of the limits to static cold storage using subnormothermic machine perfusion. Int J Artif Organs. (2013) 36(11):775–80. doi: 10.5301/ijao.5000250
13. Dubbeld J, Hoekstra H, Farid W, Ringers J, Porte RJ, Metselaar HJ, et al. Similar liver transplantation survival with selected cardiac death donors and brain death donors. Br J Surg. (2010) 97(5):744–53. doi: 10.1002/bjs.7043
14. Fernandez AR, Sanchez-Tarjuelo R, Cravedi P, Ochando J, Lopez-Hoyos M. Review: ischemia reperfusion injury – a translational perspective in organ transplantation. Int J Mol Sci. (2020) 21(22). doi: 10.3390/ijms21228549
15. Perico N, Cattaneo D, Sayegh MH, Remuzzi G. Delayed graft function in kidney transplantation. Lancet. (2004) 364(9447):1814–27. doi: 10.1016/S0140-6736(04)17406-0
16. Raigani S, De Vries RJ, Carroll C, Chen YW, Chang DC, Shroff SG. Viability testing of discarded livers with normothermic machine perfusion: alleviating the organ shortage outweighs the cost. Clin Transplant. (2020) 34(11):e14069. doi: 10.1111/ctr.14069
17. Resch T, Cardini B, Oberhuber RA, Weissenbacher A, Dumfarth J, Krapf C, et al. Transplanting marginal organs in the era of modern machine perfusion and advanced organ monitoring. Front Immunol. (2020) 11:631. doi: 10.3389/fimmu.2020.00631
18. Bruinsma BG, Avruch JH, Sridharan GV, Weeder PD, Jacobs L, Crisalli K, et al. Peritransplant energy changes and their correlation to outcome after human liver transplantation. Transplantation. (2017) 101(7):1637–44. doi: 10.1097/TP.0000000000001699
19. Agius T, Songeon J, Klauser A, Allagnat F, Longchamp G, Ruttimann R, et al. Subnormothermic ex vivo porcine kidney perfusion improves energy metabolism: analysis using (31)P magnetic resonance spectroscopic imaging. Transplant Direct. (2022) 8(10):e1354. doi: 10.1097/TXD.0000000000001354
20. Karangwa SA, Dutkowski P, Fontes P, Friend PJ, Guarrera JV, Markmann JF, et al. Machine perfusion of donor livers for transplantation: a proposal for standardized nomenclature and reporting guidelines. Am J Transplant. (2016) 16(10):2932–42. doi: 10.1111/ajt.13843
21. Moers C, Smits JM, Maathuis MH, Treckmann J, van Gelder F, Napieralski BP, et al. Machine perfusion or cold storage in deceased-donor kidney transplantation. N Engl J Med. (2009) 360(1):7–19. doi: 10.1056/NEJMoa0802289
22. Guarrera JV, Henry SD, Samstein B, Odeh-Ramadan R, Kinkhabwala M, Goldstein MJ, et al. Hypothermic machine preservation in human liver transplantation: the first clinical series. Am J Transplant. (2010) 10(2):372–81. doi: 10.1111/j.1600-6143.2009.02932.x
23. van Rijn R, Karimian N, Matton APM, Burlage LC, Westerkamp AC, van den Berg AP, et al. Dual hypothermic oxygenated machine perfusion in liver transplants donated after circulatory death. Br J Surg. (2017) 104(7):907–17. doi: 10.1002/bjs.10515
24. Schlegel A, Porte R, Dutkowski P. Protective mechanisms and current clinical evidence of hypothermic oxygenated machine perfusion (HOPE) in preventing post-transplant cholangiopathy. J Hepatol. (2022) 76(6):1330–47. doi: 10.1016/j.jhep.2022.01.024
25. van Rijn R, van Leeuwen OB, Matton APM, Burlage LC, Wiersema-Buist J, van den Heuvel MC, et al. Hypothermic oxygenated machine perfusion reduces bile duct reperfusion injury after transplantation of donation after circulatory death livers. Liver Transpl. (2018) 24(5):655–64. doi: 10.1002/lt.25023
26. Burlage LC, Karimian N, Westerkamp AC, Visser N, Matton APM, van Rijn R, et al. Oxygenated hypothermic machine perfusion after static cold storage improves endothelial function of extended criteria donor livers. HPB. (2017) 19(6):538–46. doi: 10.1016/j.hpb.2017.02.439
27. Schlegel A, Muller X, Kalisvaart M, Muellhaupt B, Perera M, Isaac JR, et al. Outcomes of DCD liver transplantation using organs treated by hypothermic oxygenated perfusion before implantation. J Hepatol. (2019) 70(1):50–7. doi: 10.1016/j.jhep.2018.10.005
28. Mueller M, Kalisvaart M, O'Rourke J, Shetty S, Parente A, Muller X, et al. Hypothermic oxygenated liver perfusion (HOPE) prevents tumor recurrence in liver transplantation from donation after circulatory death. Ann Surg. (2020) 272(5):759–65. doi: 10.1097/SLA.0000000000004258
29. van Rijn R, Schurink IJ, de Vries Y, van den Berg AP, Cortes Cerisuelo M, Darwish Murad S, et al. Hypothermic machine perfusion in liver transplantation: a randomized trial. N Engl J Med. (2021) 384(15):1391–401. doi: 10.1056/NEJMoa2031532
30. Bruggenwirth IMA, van Leeuwen OB, de Vries Y, Bodewes SB, Adelmeijer J, Wiersema-Buist J, et al. Extended hypothermic oxygenated machine perfusion enables ex situ preservation of porcine livers for up to 24 h. JHEP Rep. (2020) 2(2):100092. doi: 10.1016/j.jhepr.2020.100092
31. Bruinsma BG, Sridharan GV, Weeder PD, Avruch JH, Saeidi N, Ozer S, et al. Metabolic profiling during ex vivo machine perfusion of the human liver. Sci Rep. (2016) 6:22415. doi: 10.1038/srep22415
32. Bruinsma BG, Yeh H, Ozer S, Martins PN, Farmer A, Wu W, et al. Subnormothermic machine perfusion for ex vivo preservation and recovery of the human liver for transplantation. Am J Transplant. (2014) 14(6):1400–9. doi: 10.1111/ajt.12727
33. Bruinsma BG, Avruch JH, Weeder PD, Sridharan GV, Uygun BE, Karimian NG, et al. Functional human liver preservation and recovery by means of subnormothermic machine perfusion. J Vis Exp. (2015) 98. doi: 10.3791/52777
34. Tolboom H, Izamis ML, Sharma N, Milwid JM, Uygun B, Berthiaume F, et al. Subnormothermic machine perfusion at both 20 degrees C and 30 degrees C recovers ischemic rat livers for successful transplantation. J Surg Res. (2012) 175(1):149–56. doi: 10.1016/j.jss.2011.03.003
35. Fontes P, Lopez R, van der Plaats A, Vodovotz Y, Minervini M, Scott V, et al. Liver preservation with machine perfusion and a newly developed cell-free oxygen carrier solution under subnormothermic conditions. Am J Transplant. (2015) 15(2):381–94. doi: 10.1111/ajt.12991
36. Spetzler VN, Goldaracena N, Echiverri J, Kaths JM, Louis KS, Adeyi OA, et al. Subnormothermic ex vivo liver perfusion is a safe alternative to cold static storage for preserving standard criteria grafts. Liver Transpl. (2016) 22(1):111–9. doi: 10.1002/lt.24340
37. Hoyer DP, Paul A, Luer S, Reis H, Efferz P, Minor T. End-ischemic reconditioning of liver allografts: controlling the rewarming. Liver Transpl. (2016) 22(9):1223–30. doi: 10.1002/lt.24515
38. Berendsen TA, Bruinsma BG, Lee J, D'Andrea V, Liu Q, Izamis ML, et al. A simplified subnormothermic machine perfusion system restores ischemically damaged liver grafts in a rat model of orthotopic liver transplantation. Transplant Res. (2012) 1(1):6. doi: 10.1186/2047-1440-1-6
39. Karimian N, Raigani S, Huang V, Nagpal S, Hafiz EOA, Beijert I, et al. Subnormothermic machine perfusion of steatotic livers results in increased energy charge at the cost of anti-oxidant capacity compared to normothermic perfusion. Metabolites. (2019) 9(11). doi: 10.3390/metabo9110246
40. Zhang A, Carroll C, Raigani S, Karimian N, Huang V, Nagpal S, et al. Therapeutic implications of amino acid metabolism in non-steatotic discarded human livers during normothermic versus subnormothermic machine perfusion. Hepatology. (2020) 72:854A–854A.
41. Furukori M, Matsuno N, Meng LT, Shonaka T, Nishikawa Y, Imai K, et al. Subnormothermic machine perfusion preservation with rewarming for donation after cardiac death liver grafts in pigs. Transplant Proc. (2016) 48(4):1239–43. doi: 10.1016/j.transproceed.2015.12.076
42. Reddy SP, Brockmann J, Friend PJ. Normothermic perfusion: a mini-review. Transplantation. (2009) 87(5):631–2. doi: 10.1097/TP.0b013e3181995e83
43. Vogel T, Brockmann JG, Coussios C, Friend PJ. The role of normothermic extracorporeal perfusion in minimizing ischemia reperfusion injury. Transplant Rev. (2012) 26(2):156–62. doi: 10.1016/j.trre.2011.02.004
44. Markmann JF, Abouljoud MS, Ghobrial NM, Bhati CS, Pelletier SJ, Lu AD, et al. Impact of portable normothermic blood-based machine perfusion on outcomes of liver transplant: the OCS liver PROTECT randomized clinical trial. JAMA Surg. (2022) 157(3):189–98. doi: 10.1001/jamasurg.2021.6781
45. Mergental H, Stephenson BTF, Laing RW, Kirkham AJ, Neil DAH, Wallace LL, et al. Development of clinical criteria for functional assessment to predict primary nonfunction of high-risk livers using normothermic machine perfusion. Liver Transpl. (2018) 24(10):1453–69. doi: 10.1002/lt.25291
46. Mergental H, Perera MT, Laing RW, Muiesan P, Isaac JR, Smith A, et al. Transplantation of declined liver allografts following normothermic ex-situ evaluation. Am J Transplant. (2016) 16(11):3235–45. doi: 10.1111/ajt.13875
47. Watson CJE, Kosmoliaptsis V, Randle LV, Gimson AE, Brais R, Klinck JR, et al. Normothermic perfusion in the assessment and preservation of declined livers before transplantation: hyperoxia and vasoplegia-important lessons from the first 12 cases. Transplantation. (2017) 101(5):1084–98. doi: 10.1097/TP.0000000000001661
48. Nasralla D, Coussios CC, Mergental H, Akhtar MZ, Butler AJ, Ceresa CDL, et al. A randomized trial of normothermic preservation in liver transplantation. Nature. (2018) 557(7703):50–6. doi: 10.1038/s41586-018-0047-9
49. Watson CJE, Kosmoliaptsis V, Pley C, Randle L, Fear C, Crick K, et al. Observations on the ex situ perfusion of livers for transplantation. Am J Transplant. (2018) 18(8):2005–20. doi: 10.1111/ajt.14687
50. Vogel T, Brockmann JG, Quaglia A, Morovat A, Jassem W, Heaton ND, et al. The 24-hour normothermic machine perfusion of discarded human liver grafts. Liver Transpl. (2017) 23(2):207–20. doi: 10.1002/lt.24672
51. Eshmuminov D, Becker D, Bautista Borrego L, Hefti M, Schuler MJ, Hagedorn C, et al. An integrated perfusion machine preserves injured human livers for 1 week. Nat Biotechnol. (2020) 38(2):189–98. doi: 10.1038/s41587-019-0374-x
52. Clavien PA, Dutkowski P, Mueller M, Eshmuminov D, Bautista Borrego L, Weber A, et al. Transplantation of a human liver following 3 days of ex situ normothermic preservation. Nat Biotechnol. (2022) 40(11):1610–6. doi: 10.1038/s41587-022-01354-7
53. Minor T, Efferz P, Fox M, Wohlschlaeger J, Luer B. Controlled oxygenated rewarming of cold stored liver grafts by thermally graduated machine perfusion prior to reperfusion. Am J Transplant. (2013) 13(6):1450–60. doi: 10.1111/ajt.12235
54. Hoyer DP, Benko T, Manka P, von Horn C, Treckmann JW, Paul A, et al. Long-term outcomes after controlled oxygenated rewarming of human livers before transplantation. Transplant Direct. (2020) 6(4):e542. doi: 10.1097/TXD.0000000000000987
55. Boteon YL, Laing RW, Schlegel A, Wallace L, Smith A, Attard J, et al. Combined hypothermic and normothermic machine perfusion improves functional recovery of extended criteria donor livers. Liver Transpl. (2018) 24(12):1699–715. doi: 10.1002/lt.25315
56. de Vries Y, Matton APM, Nijsten MWN, Werner MJM, van den Berg AP, de Boer MT, et al. Pretransplant sequential hypo- and normothermic machine perfusion of suboptimal livers donated after circulatory death using a hemoglobin-based oxygen carrier perfusion solution. Am J Transplant. (2019) 19(4):1202–11. doi: 10.1111/ajt.15228
57. van Leeuwen OB, de Vries Y, Fujiyoshi M, Nijsten MWN, Ubbink R, Pelgrim GJ, et al. Transplantation of high-risk donor livers after ex situ resuscitation and assessment using combined hypo- and normothermic machine perfusion: a prospective clinical trial. Ann Surg. (2019) 270(5):906–14. doi: 10.1097/SLA.0000000000003540
58. Pegg DE. Principles of cryopreservation. Methods Mol Biol. (2007) 368:39–57. doi: 10.1007/978-1-59745-362-2_3
59. Jang TH, Park SC, Yang JH, Kim JY, Seok JH, Park US, et al. Cryopreservation and its clinical applications. Integr Med Res. (2017) 6(1):12–8. doi: 10.1016/j.imr.2016.12.001
60. Fahy GM, Wowk B, Wu J. Cryopreservation of complex systems: the missing link in the regenerative medicine supply chain. Rejuvenation Res. (2006) 9(2):279–91. doi: 10.1089/rej.2006.9.279
61. Fowler A, Toner M. Cryo-injury and biopreservation. Ann N Y Acad Sci. (2005) 1066:119–35. doi: 10.1196/annals.1363.010
62. Whaley D, Damyar K, Witek RP, Mendoza A, Alexander M, Lakey JR. Cryopreservation: an overview of principles and cell-specific considerations. Cell Transplant. (2021) 30:963689721999617. doi: 10.1177/0963689721999617
63. Mazur P. The role of intracellular freezing in the death of cells cooled at supraoptimal rates. Cryobiology. (1977) 14(3):251–72. doi: 10.1016/0011-2240(77)90175-4
64. Fuller BJ, Petrenko AY, Rodriguez JV, Somov AY, Balaban CL, Guibert EE. Biopreservation of hepatocytes: current concepts on hypothermic preservation, cryopreservation, and vitrification. Cryo Lett. (2013) 34(4):432–52.
65. Jabr F. What the supercool arctic ground squirrel teaches us about the brain’s resilience (2012). Available at: https://www.scientificamerican.com/article/arctic-ground-squirrel-brain/.
66. Bruinsma BG, Uygun K. Subzero organ preservation: the dawn of a new ice age? Curr Opin Organ Transplant. (2017) 22(3):281–6. doi: 10.1097/MOT.0000000000000403
67. Rudolf LE, Mandel S. Supercooling, intermittent perfusion, and high pressure oxygen in whole organ preservation. Transplantation. (1967) 5(4):1159–66. doi: 10.1097/00007890-196707001-00053
68. Usta OB, Kim Y, Ozer S, Bruinsma BG, Lee J, Demir E, et al. Supercooling as a viable non-freezing cell preservation method of rat hepatocytes. PLoS One. (2013) 8(7):e69334. doi: 10.1371/journal.pone.0069334
69. Berendsen TA, Bruinsma BG, Puts CF, Saeidi N, Usta OB, Uygun BR, et al. Supercooling enables long-term transplantation survival following 4 days of liver preservation. Nat Med. (2014) 20(7):790–3. doi: 10.1038/nm.3588
70. Sugimachi K, Roach KL, Rhoads DB, Tompkins RG, Toner M. Nonmetabolizable glucose compounds impart cryotolerance to primary rat hepatocytes. Tissue Eng. (2006) 12(3):579–88. doi: 10.1089/ten.2006.12.579
71. de Vries RJ, Tessier SN, Banik PD, Nagpal S, Cronin SEJ, Ozer S, et al. Supercooling extends preservation time of human livers. Nat Biotechnol. (2019) 37(10):1131–6. doi: 10.1038/s41587-019-0223-y
72. de Vries RJ, Tessier SN, Banik PD, Nagpal S, Cronin SEJ, Ozer S, et al. Subzero non-frozen preservation of human livers in the supercooled state. Nat Protoc. (2020) 15(6):2024–40. doi: 10.1038/s41596-020-0319-3
73. Huang H, Yarmush ML, Usta OB. Long-term deep-supercooling of large-volume water and red cell suspensions via surface sealing with immiscible liquids. Nat Commun. (2018) 9(1):3201. doi: 10.1038/s41467-018-05636-0
74. Huang H, Rey-Bedon C, Yarmush ML, Usta OB. Deep-supercooling for extended preservation of adipose-derived stem cells. Cryobiology. (2020) 92:67–75. doi: 10.1016/j.cryobiol.2019.11.004
75. Sanz E, Vega C, Espinosa JR, Caballero-Bernal R, Abascal JL, Valeriani C. Homogeneous ice nucleation at moderate supercooling from molecular simulation. J Am Chem Soc. (2013) 135(40):15008–17. doi: 10.1021/ja4028814
76. Storey KB. Life in a frozen state: adaptive strategies for natural freeze tolerance in amphibians and reptiles. Am J Physiol. (1990) 258(3 Pt 2):R559–68. doi: 10.1152/ajpregu.1990.258.3.R559
77. Wolanczyk JP, Storey KB, Baust JG. Ice nucleating activity in the blood of the freeze-tolerant frog, Rana sylvatica. Cryobiology. (1990) 27(3):328–35. doi: 10.1016/0011-2240(90)90032-Y
78. Tessier SN, Weng L, Moyo WD, Au SH, Wong KHK, Angpraseuth C, et al. Effect of ice nucleation and cryoprotectants during high subzero-preservation in endothelialized microchannels. ACS Biomater Sci Eng. (2018) 4(8):3006–15. doi: 10.1021/acsbiomaterials.8b00648
79. Tessier SN, de Vries RJ, Pendexter CA, Cronin SEJ, Ozer S, Hafiz EOA, et al. Partial freezing of rat livers extends preservation time by 5-fold. Nat Commun. (2022) 13(1):4008.35840553
80. Ukpai G, Nastase G, Serban A, Rubinsky B. Pressure in isochoric systems containing aqueous solutions at subzero centigrade temperatures. PLoS One. (2017) 12(8):e0183353. doi: 10.1371/journal.pone.0183353
81. Preciado JA, Rubinsky B. Isochoric preservation: a novel characterization method. Cryobiology. (2010) 60(1):23–9. doi: 10.1016/j.cryobiol.2009.06.010
82. Wan L, Powell-Palm MJ, Lee C, Gupta A, Weegman BP, Clemens MG, et al. Preservation of rat hearts in subfreezing temperature isochoric conditions to −8 degrees C and 78 MPa. Biochem Biophys Res Commun. (2018) 496(3):852–7. doi: 10.1016/j.bbrc.2018.01.140
83. Powell-Palm MJ, Charwat V, Charrez B, Siemons B, Healy KE, Rubinsky B. Isochoric supercooled preservation and revival of human cardiac microtissues. Commun Biol. (2021) 4(1):1118. doi: 10.1038/s42003-021-02650-9
84. Fahy GM, MacFarlane DR, Angell CA, Meryman HT. Vitrification as an approach to cryopreservation. Cryobiology. (1984) 21(4):407–26. doi: 10.1016/0011-2240(84)90079-8
85. Boyle R, Hobbes T, Merret C. New experiments and observations touching cold. London: John Crook (1665).
86. Polge C, Smith AU, Parkes AS. Revival of spermatozoa after vitrification and dehydration at low temperatures. Nature. (1949) 164(4172):666. doi: 10.1038/164666a0
87. Mazur P. Freezing of living cells: mechanisms and implications. Am J Physiol. (1984) 247(3 Pt 1):C125–42. doi: 10.1152/ajpcell.1984.247.3.C125
88. Pegg DE. The relevance of ice crystal formation for the cryopreservation of tissues and organs. Cryobiology. (2010) 60(3 Suppl):S36–44. doi: 10.1016/j.cryobiol.2010.02.003
89. Meryman HT. Mechanics of freezing in living cells and tissues. Science. (1956) 124(3221):515–21. doi: 10.1126/science.124.3221.515
91. Rapatz G, Luyet B. Electron microscope study of erythrocytes in rapidly cooled suspensions containing various concentrations of glycerol. Biodynamica. (1968) 10(210):193–210.5725456
92. Rall WF, Fahy GM. Ice-free cryopreservation of mouse embryos at −196 degrees C by vitrification. Nature. (1985) 313(6003):573–5. doi: 10.1038/313573a0
93. Sharma A, Rao JS, Han Z, Gangwar L, Namsrai B, Gao Z, et al. Vitrification and nanowarming of kidneys. Adv Sci. (2021) 8(19):e2101691. doi: 10.1002/advs.202101691
94. Gao Z, Namsrai B, Han Z, Joshi P, Rao JS, Ravikumar V, et al. Vitrification and rewarming of magnetic nanoparticle-loaded rat hearts. Adv Mater Technol. (2022) 7(3). doi: 10.1002/admt.202100873
95. Chiu-Lam A, Staples E, Pepine CJ, Rinaldi C. Perfusion, cryopreservation, and nanowarming of whole hearts using colloidally stable magnetic cryopreservation agent solutions. Sci Adv. (2021) 7(2). doi: 10.1126/sciadv.abe3005
96. Sharma A, Lee CY, Namsrai BE, Han Z, Tobolt D, Rao JS, et al. Cryopreservation of whole rat livers by vitrification and nanowarming. Ann Biomed Eng. (2022):51. doi: 10.1007/s10439-022-03064-2
97. Manuchehrabadi N, Gao Z, Zhang J, Ring HL, Shao Q, Liu F, et al. Improved tissue cryopreservation using inductive heating of magnetic nanoparticles. Sci Transl Med. (2017) 9(379). doi: 10.1126/scitranslmed.aah4586
98. Hossay C, Donnez J, Dolmans MM. Whole ovary cryopreservation and transplantation: a systematic review of challenges and research developments in animal experiments and humans. J Clin Med. (2020) 9(10). doi: 10.3390/jcm9103196
99. Wowk B. Thermodynamic aspects of vitrification. Cryobiology. (2010) 60(1):11–22. doi: 10.1016/j.cryobiol.2009.05.007
100. Karow AM Jr. Cryoprotectants – a new class of drugs. J Pharm Pharmacol. (1969) 21(4):209–23. doi: 10.1111/j.2042-7158.1969.tb08235.x
101. Elliott GD, Wang S, Fuller BJ. Cryoprotectants: a review of the actions and applications of cryoprotective solutes that modulate cell recovery from ultra-low temperatures. Cryobiology. (2017) 76:74–91. doi: 10.1016/j.cryobiol.2017.04.004
102. Fahy GM. Cryoprotectant toxicity neutralization. Cryobiology. (2010) 60(3 Suppl):S45–53. doi: 10.1016/j.cryobiol.2009.05.005
103. Fahy GM, Wowk B, Pagotan R, Chang A, Phan J, Thomson B, et al. Physical and biological aspects of renal vitrification. Organogenesis. (2009) 5(3):167–75. doi: 10.4161/org.5.3.9974
104. Wusteman M, Robinson M, Pegg D. Vitrification of large tissues with dielectric warming: biological problems and some approaches to their solution. Cryobiology. (2004) 48(2):179–89. doi: 10.1016/j.cryobiol.2004.01.002
105. Burdette EC, Karow AM, Jeske AH. Design, development, and performance of an electromagnetic illumination system for thawing cryopreserved kidneys of rabbits and dogs. Cryobiology. (1978) 15(2):152–67. doi: 10.1016/0011-2240(78)90020-2
106. Jackson TH, Ungan A, Critser JK, Gao D. Novel microwave technology for cryopreservation of biomaterials by suppression of apparent ice formation. Cryobiology. (1997) 34(4):363–72. doi: 10.1006/cryo.1997.2016
107. Ecker HA, Burdette EC, Cain FL. Simultaneous microwave and HF thawing of cryogenically preserved canine kidneys. (1976).
108. Etheridge ML, Xu Y, Rott L, Choi J, Glasmacher B, Bischof JC. RF heating of magnetic nanoparticles improves the thawing of cryopreserved biomaterials. Technology. (2014) 2(3):229–42. doi: 10.1142/S2339547814500204
109. Gao Z, Ring HL, Sharma A, Namsrai B, Tran N, Finger EB, et al. Preparation of scalable silica-coated iron oxide nanoparticles for nanowarming. Adv Sci. (2020) 7(4):1901624. doi: 10.1002/advs.201901624
110. Boteon YL, Hessheimer AJ, Bruggenwirth IMA, Boteon A, Padilla M, de Meijer VE, et al. The economic impact of machine perfusion technology in liver transplantation. Artif Organs. (2022) 46(2):191–200. doi: 10.1111/aor.14131
111. Watson CJE, Hunt F, Messer S, Currie I, Large S, Sutherland A, et al. In situ normothermic perfusion of livers in controlled circulatory death donation may prevent ischemic cholangiopathy and improve graft survival. Am J Transplant. (2019) 19(6):1745–58. doi: 10.1111/ajt.15241
112. Barrou B, Billault C, Nicolas-Robin A. The use of extracorporeal membranous oxygenation in donors after cardiac death. Curr Opin Organ Transplant. (2013) 18(2):148–53. doi: 10.1097/MOT.0b013e32835e29f5
113. Kidney transplantation: past, present, and future. Available at: https://web.stanford.edu/dept/HPST/transplant/html/history.html.
114. The official Dr. Thomas E. Starzl web site. Available at: https://www.starzl.pitt.edu/about/starzl.html.
115. Belzer F. Organ preservation: a personal perspective. Available at: https://web.stanford.edu/dept/HPST/transplant/html/belzer.html.
Keywords: organ transplantation, liver preservation, machine perfusion, subzero preservation, nanowarming, supercooling, isochoric preservation, partial freezing
Citation: Ozgur OS, Namsrai B-E, Pruett TL, Bischof JC, Toner M, Finger EB and Uygun K (2023) Current practice and novel approaches in organ preservation. Front. Transplant. 2:1156845. doi: 10.3389/frtra.2023.1156845
Received: 1 February 2023; Accepted: 16 May 2023;
Published: 8 June 2023.
Edited by:
Hirofumi Hirao, Kyoto University, JapanReviewed by:
Philipp Dominique Kron, University Hospital Zürich, SwitzerlandTheresa Hautz, Innsbruck Medical University, Austria
© 2023 Ozgur, Namsrai, Pruett, Bischof, Toner, Finger and Uygun. This is an open-access article distributed under the terms of the Creative Commons Attribution License (CC BY). The use, distribution or reproduction in other forums is permitted, provided the original author(s) and the copyright owner(s) are credited and that the original publication in this journal is cited, in accordance with accepted academic practice. No use, distribution or reproduction is permitted which does not comply with these terms.
*Correspondence: Korkut Uygun a3V5Z3VuQG1naC5oYXJ2YXJkLmVkdQ==