- 1National Renewable Energy Laboratory, Golden, CO, United States
- 2Renewable and Sustainable Energy Institute, University of Colorado, Boulder, CO, United States
- 3Institute of Energy: Sustainability, Environment and Equity, Stony Brook University, Stony Brook, NY, United States
- 4Department of Chemistry, Stony Brook University, Stony Brook, NY, United States
- 5Department of Materials Science and Chemical Engineering, Stony Brook University, Stony Brook, NY, United States
- 6Interdisciplinary Science Department, Brookhaven National Laboratory, Upton, NY, United States
The transition to a decarbonized economy will drive dramatically higher demand for energy storage, along with technological diversification. To avoid mistakes of the past, the supply chain implications and environmental and social justice (ESJ) impacts of new battery technologies should be considered early during technological development. We propose herein a systematic framework for analyzing these impacts for new stationary battery technologies and illustrate the framework with a case study. The goal is to promote future development of technologies with secure supply chains and favorable ESJ profiles to avoid expensive corrective actions after substantial resources have been invested. This framework should be a useful tool for public and private researchers and sponsors who want to ensure that supply chain and ESJ concerns are considered and integrated as part of decision making throughout the research and development process.
1. Introduction
The transition to a decarbonized economy is expected to drive dramatically higher demand for energy storage (National Academies of Sciences, 2021). The International Energy Agency (IEA, 2022a) projects that—under current policies—global storage demand will grow from 71 to 5,200 GWh between 2020 and 2050, while U.S. storage demand will grow from 15 to 1,500 GWh; in a net-zero case, the demands are about three times higher. About 90% of this energy storage demand is needed to satisfy the lithium-ion battery (LIB) market, which is currently dominated by electric vehicles (EVs) (IEA, 2022a). However, the smaller stationary energy storage market also relies mostly on LIBs (Sandia National Laboratories, 2023).
Developing new battery types is crucial for meeting future demands, particularly for stationary applications. The operating conditions and performance requirements of stationary applications are much more diverse than those of EVs. It is unlikely that any one storage solution could optimally satisfy stationary and mobile needs, so purpose-driven storage designs are required (Trahey, 2020). In addition, safety issues related to LIBs may be of concern for stationary applications (Huang and Li, 2022), and current LIBs are generally not cost-effective for long-duration energy storage (10 h or more) (Tuttman and Litzelman, 2020; Wood Mackenzie, 2022). Further, the pressure on LIB supply chains and prices due to soaring EV demand should motivate the search for alternative stationary storage technologies.
Supply chain issues for LIBs have been studied extensively and have identified supply chain concerns for a range of materials used in commercial battery technologies, including lithium, cobalt, nickel, vanadium, and manganese (White House, 2021; IEA, 2022b; Mann, 2022; MIT, 2022). Concerns include the concentration of critical materials in a few locations, foreign control of refining and developing cathode precursors and natural graphite anodes, and instability of the primary cobalt supplier. These supply chain studies occurred predominantly after the LIB technologies were already in the market.
The social and environmental impacts of existing battery technologies were also studied after deployment. Life cycle assessments (LCAs) have identified the contribution of battery production steps to environmental impacts (e.g., Liu et al., 2015; Peters et al., 2017; Arshad et al., 2022; Das, 2023). Studies have shown high fatality rates and child labor associated with cobalt mining in the Democratic Republic of the Congo, health impacts from the production of intermediate compounds such as cobalt and nickel sulfates, and harmful emissions of lithium and lead into ecosystems and communities (e.g., Shanika et al., 2013; Ericson et al., 2016; Bolan et al., 2021; Murdock et al., 2021; Arvidsson et al., 2022). Owen et al. (2023) found that over 70% of mining activities for the top 17 energy transition minerals were among Indigenous and/or low-income community areas.
Circular economy strategies have also lagged battery deployment. Not until 1995, for example, did the U.S. begin implementing lead acid battery (LAB) recycling requirements (Turner, 2022). The LAB recycling rate is now 99% in the U.S. and other Western countries (ILA, 2021). Rates are much lower in developing countries, which have commensurate environmental and health problems (e.g., WHO, 2017). LIB recycling is in its infancy but has shown promise in reducing life cycle impacts compared with virgin production (DOE, 2023).
To avoid mistakes of the past, the supply chain implications and the environmental and social impacts of new battery technologies should be considered early during technological development. We propose a systematic framework to analyze the impacts of new stationary battery technologies. Supplementary Figure S1 illustrates the interconnected analyses needed in the proposed framework. The goal is to promote the development of technologies with secure supply chains and favorable environmental and social justice (ESJ) profiles and to avoid expensive corrective actions after substantial resources have been invested. Although many other characteristics are important for technological success, identifying a diverse slate of potentially abundant materials with limited negative impacts is a critical first step toward satisfying future energy storage system needs.
2. Framework for early-stage consideration of supply chains and ESJ
In our framework—which brings together decades of experience conducting technoeconomic, life cycle ESJ, and supply chain analyses—supply chain and ESJ considerations are analyzed in concert with the development of new battery technologies at early technology readiness levels (TRLs) (Figure 1; Supplementary Figure S2). At all stages of research, sensitivity analyses are regularly conducted to understand factors that dominate impacts, informing potential shifts in research. At TRL 1, the battery development team identifies promising future battery materials (FBMs), the ESJ analysis focuses on impacts from mining only, and the supply chain analysis focuses on identifying FBM locations, reserves, and resources. Thus, the researchers can assess tradeoffs between FBM technical benefits and their supply chain and ESJ impacts at an early stage, along with potential alternatives.
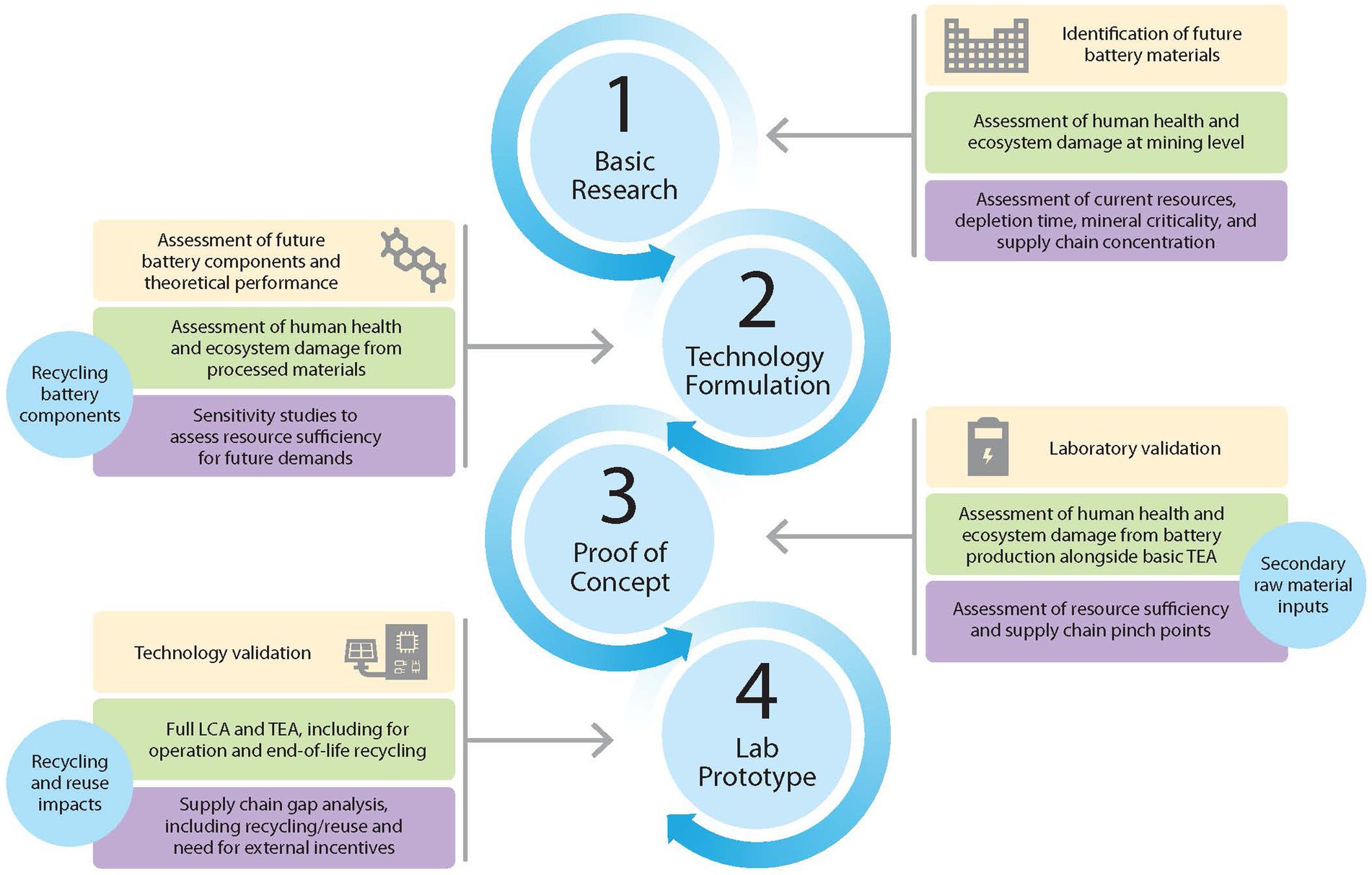
Figure 1. Framework for concurrent supply chain and ESJ assessments at early TRL stages during future battery development.
At TRL 2, the development team identifies potential battery components (e.g., cathodes) and compiles preliminary technical information, such as specific energy density. The supply chain analysis focuses on the sufficiency of reserves based on reported technical performance. The ESJ analyses are based on demands from the supply chain, including the refining stage. Toxicity is evaluated by comparing it with standard toxicity lists. The circular economy is assessed by determining if processes exist to recover and recycle the components and/or FBMs.
At TRL 3, other components are identified (e.g., electrolyte, separator), preliminary performance characteristics (e.g., operating range) are demonstrated, and battery lifetime is estimated. A preliminary techno-economic analysis (TEA) provides information about raw materials and manufacturing processes required, which are used to identify social and socioeconomic risks related to the future product’s supply chain. Locations of recycling facilities can be included, as well as the identification of potential recycling processes and secondary uses for materials and components. Information about where it is most economic to extract and process materials based on the TEA and supply chain information can be used to perform LCA to identify potential positive (e.g., job growth) and negative (e.g., environmental degradation) impacts on relevant communities.
At TRL 4, a lab prototype is used to demonstrate system performance under various conditions. Analyses of expected battery lifetime, other performance characteristics, and suitable applications are refined, and the bill of materials is developed. With this information, TEA and LCA are refined to include recycling and/or reuse impacts.
The following subsections discuss our initial proposed supply chain and ESJ analyses. Section 3 illustrates the framework with a case study covering TRL 1 and TRL 2.
2.1. Supply chain
Supply chain analyses encompass each part of the battery technology life cycle. The upstream sector includes material extraction and upgrading of minerals into refined chemical precursors for the batteries. The midstream sector covers development of subcomponents such as the cathode, anode, separator, and electrolyte. The downstream sector encompasses manufacturing of battery cells and packaging into packs, racks, and other systems. Finally, end-of-life steps include battery reuse or recycling into new batteries. Here we describe several supply chain assessments.
2.1.1. Specific energy density
Specific energy density characterizes the battery energy storage capacity by mass (kWh/kg) and, along with battery chemistry, can be used to calculate the amount of each element needed to meet a specific energy storage demand. These data can be estimated from literature values, typically as per kilogram of battery, per kilogram of active materials, or a generic “per kilogram.” In per-battery or per-kilogram terms, other information—such as bill of materials, typical concentrations of catholyte in flow batteries, and/or economic analyses of batteries—can be used to derive the amount of FBM used. Finally, the specific energy density can be converted to kWh/kg of reactants using the battery chemistry. See Supplementary Figure S3 for more information.
2.1.2. Reserves, resources, and production
Global and regional reserves, resources, and production are used to assess the availability of mineral resources (USGS, 2022). In general, reserves estimate the amount of minerals that can be economically extracted currently, and resources estimate the amount that can be extracted in the future with different technology and economic circumstances. The material required is compared to the global or domestic reserve or resource to determine if there is enough material to sustain potential future battery production. The current global production is also compared to the global reserve to determine the time that the reserve will last under current extraction rates. Finally, the supply growth rate required to meet the new demand can be compared to the current growth rate and/or demand.
2.1.3. Market concentration
Market concentration indicates the level of competition in the market. One measure of market concentration is the Herfindahl–Hirschman Index (HHI) (Hirschman, 2018), which can be used to evaluate the competitiveness of the mineral market supply. Lower competition in a market incurs a higher supply risk, while a more competitive market has higher diversity in materials sourcing, increasing the market’s resilience. HHI is a function of the market share of each firm, in this case country (n):
HHI results are classified into three market types: unconcentrated (HHI < 1,500), moderately concentrated (1,500 < HHI < 2,500), and highly concentrated (HHI > 2,500) (Calkins, 1983).
2.1.4. Critical minerals
The U.S. Energy Act of 2020 defines a critical mineral as a non-fuel mineral or mineral material essential to economic or national security that has a supply chain vulnerable to disruption (U.S. Congress, 2021). The full methodology for determining critical minerals is outlined in Nassar and Fortier (2021).
2.2. Environmental and social justice
Our ESJ framework leverages guidance from the literature to identify ESJ hot spots at early TRL stages and provide a high-level quantitative analysis of the potential impacts of technology deployment (Huijbregts et al., 2016; EPA, 2023). At TRL 1, we use two ESJ metrics based on LCA of FBM mining and extraction of primary ores only: disability-adjusted life years (DALYs) to measure human health impacts (years lost or impacted from diseases or accidents) and the ReCiPe method to measure terrestrial ecosystem damage (yearly loss of species) (Huijbregts et al., 2016). Supplementary Figures S4–S6 provide results on the contributing geo-specific and prospective impacts. At TRL 2, we identify whether potential chemistries for the anode and cathode are hazardous or toxic based on the Toxic Substances Control Act (TSCA) inventory (EPA, 2023). As research progresses to the next TRL stages, ESJ analyses build on analytical results from previous TRLs and become more targeted, with increased detail to identify potentially impactful materials or activities based on a full suite of environmental and social impacts.
2.3. Circular economy
The circular economy maintains a circular flow of resources by regenerating, retaining, or adding to their value while contributing to sustainable development (ISO, 2023). Designing for circularity includes product performance, durability, repairability, recyclability, and avoiding the use of hazardous substances (UNECE, 2023). In this way, circular economy strategies can help improve both supply chain and ESJ considerations.
The circular economy strategies evaluated and the depth of their evaluation vary by TRL. At TRLs 1 and 2, an evaluation could focus on product manufacturing, use, and material recovery to consider if the product is needed, the presence of hazardous or toxic substances, and material recyclability. As the research progresses, circular economy strategies for longevity come into play. Material flow analysis and LCAs are two methods used to quantify the impacts of circular economy strategies, beginning at TRLs 3 and 4.
3. Applying the framework—A case study
A case study illustrates how our proposed framework could be used to analyze supply chain and ESJ factors during early stationary battery development. Potential elements are identified at TRL 1, which informs selection of potential cathode and anode materials to be evaluated at TRL 2.
3.1. Evaluation at TRL 1: basic research
At TRL 1, we screen 27 promising FBMs using supply chain and ESJ parameters; Supplementary Table S1 includes a complete list of battery types and chemistries considered. We use a stoplight diagram to compare numerous disparate parameters (Figure 2). Multiple parameters are aggregated into three categories: combined global supply chain indicator (world reserves, years to economic depletion, and HHI), combined domestic supply chain indicator (U.S. reserves and percent reliance on net imports), and combined ESJ indicator (DALY and terrestrial ecosystem damage). The subcomponents of each indicator are averaged—each having an equal weight—and color-coded. In Figure 2, values denoting relatively minor or desirable impacts are at the blue end of the color spectrum, values denoting relatively major or undesirable impacts are at the red end, and values in between are in light blue, yellow, and orange. For ESJ impacts, we compare only mining and extraction of the primary ores for the selected elements, owing to the lack of data and uncertainty in battery chemistry.
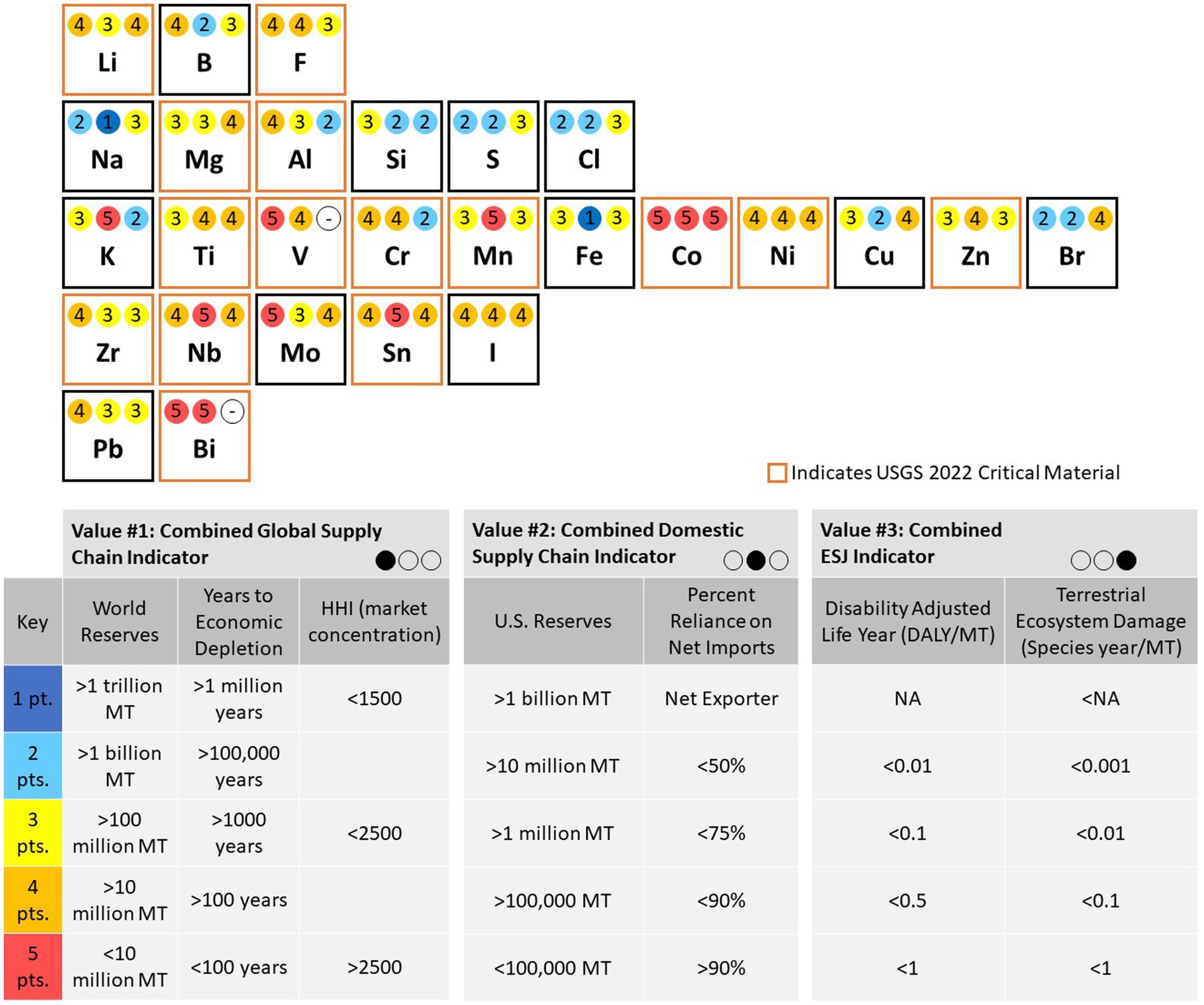
Figure 2. Stoplight diagram of FBMs. The environmental impacts of bismuth and vanadium extraction are unavailable in the ecoinvent 3.8 database (ecoinvent, 2023) used for this analysis because these elements are minor byproducts from extraction of other materials.
Now, promising (blue end of the spectrum) and unpromising (red end) elements can be identified. Elements such as silicon, sulfur, chlorine, and sodium appear promising. Elements such as tin, cobalt, niobium, and bismuth appear particularly unpromising. However, it is unlikely any element would be summarily dismissed based on this assessment alone, because potential technical benefits and specific applications have not been considered. Instead, the analysis at TRL 1 provides a starting point for incorporating supply chain and ESJ considerations into future battery research and impetus to mitigate inordinately negative impacts or explore alternatives. The analyses at subsequent TRLs build upon this foundation.
3.2. Evaluation at TRL 2: technology formulation
At TRL 2, researchers begin to identify specific components (e.g., cathodes) of potential batteries, so analysts can begin evaluating impacts based on component manufacturing processes, performance, toxicity, and so forth. To demonstrate the framework at TRL 2, we analyze the components of a battery with relatively favorable elements (no red designations) from Figure 2: a BrCl battery with an aluminum anode. Supplementary Figure S7 shows the analysis boundaries between TRL 1 and TRL 2. Information on the production process of BrCl cathodes was unavailable, so we analyzed chlorine and bromine purification/refining separately. Data on detailed production processes at TRL 3 would improve these estimates but are beyond the scope of this simplified case study.
Supplementary Table S2 lists the TRL 2 analysis results, and Supplementary Tables S3–S12 show the data and calculations. The supply chain analysis for BrCl-Al shows that the U.S. demand for aluminum and bromine would be very high relative to U.S. reserves, but global demand for all materials would be very low relative to global reserves. These preliminary results are only indicative, as the numbers in the table assume both a maximum theoretical specific energy density—775 Wh/kg (Cao et al., 2020)—and that BrCl-Al batteries would meet the entire grid storage demand. In TRL 3, these calculations would be refined to capture impacts more accurately by matching the expected technical performance in other areas (e.g., lifetime, response) to suitable applications (e.g., peaking). Sensitivity analyses are critical for mitigating the impact of uncertainties in the assumed input data.
Our ESJ analysis suggests that two-thirds of the estimated human health and ecosystem impacts from BrCl-Al batteries relate to bromine production. Aluminum production accounts for a quarter of those impacts, and chlorine production accounts for the remaining 7–8%. Bromine and chlorine are also active on the TSCA inventory. The relative impacts of aluminum and chlorine illustrate the insights provided by analyses at multiple TRLs. For the extraction activities at TRL 1, the human health and ecosystem impacts of chlorine and aluminum are somewhat similar (Figure 2). However, once the BrCl-Al battery chemistry is defined and the production processes analyzed at TRL 2, aluminum exhibits less favorable impacts. This is because aluminum produces more impact per unit, contributing only 5% of the mass but 25% of the impact.
The absolute values of human health and ecosystem impacts require additional context. All industrial activities entail such negative impacts, and clean energy technologies also can produce positive health and ecosystem impacts by offsetting impacts from more polluting alternatives. As a frame of reference, unregulated LAB recycling was associated with 127,248–1,612,476 DALYs in 2013 (Ericson et al., 2016). In addition, the distribution of impacts must be considered. ESJ issues are magnified if health and ecosystem impacts fall inordinately on a few areas. In any case, impacts must be evaluated in comparison to alternative technologies to identify the least impactful solution.
Finally, the circular economy status of each component must be considered. Aluminum already has a robust recycling industry, and in 2020 the global recycling efficiency rate was over 75%. Aluminum recycling requires only 5% of the energy used to produce primary aluminum (Aluminium Recycling, 2023). In contrast, no recycling processes exist for bromine or chlorine.
As a comparison with the BrCl-Al results, Supplementary Table S2 also lists the TRL 2 analysis results for another battery chemistry with generally favorable aspects in Figure 2: an SiF4 battery with an aluminum anode. Supplementary Tables S13–S19 show the data and calculations. This analysis provides detail on the potential supply chain issues for fluorine and aluminum. In addition, it shows the importance of accounting for the ESJ impacts of material production steps at TRL 2. For example, extraction-related ESJ impacts are greater for bromine than for silicon (Figure 2), but production-related ESJ impacts are greater for silicon than for bromine (Supplementary Table S2). This example shows how the analysis framework can be used to compare battery chemistries at an early stage. However, significant uncertainties remain at this stage, as discussed in Section 4, and the preferability of one battery type over another cannot be determined definitively. Rather, such a comparison adds another dimension to the iterative evaluation of potential battery chemistries that continues to be refined as research progresses along TRLs. Note that, for this initial sketch of an analysis framework, we only developed a scoring system for TRL 1 (Figure 2, the stoplight diagram). Scoring systems could also be developed for other TRLs to facilitate comparison of alternatives.
4. Discussion
It is important to develop novel battery chemistries that can meet growing energy storage demand in a wide variety of locations and applications—and to do so in a sustainable, just, and environmentally responsible manner while ensuring secure supply chains. Potential issues can be mitigated by aligning technology development with supply chain and ESJ concerns as early as possible. In addition, early identification of problematic technologies can provide financial benefits by preventing investments in ultimately unacceptable technologies or spurring early changes so the technologies can become acceptable.
We outline a framework for scientists developing novel grid storage batteries at the lab scale. The framework is meant to help researchers develop new batteries more effectively through early identification and mitigation of potential supply chain and ESJ issues. It applies equally well to government-sponsored and industrial research and is useful for public and private funders who want to ensure supply chain and ESJ concerns are integrated with the development process, rather than an afterthought. For example, the framework could be incorporated into initiatives like the Justice40 Initiative, which is driving the inclusion of ESJ criteria into federally funded research opportunities in the United States, as well as public and private efforts to achieve United Nations Sustainable Development Goals (United Nations, 2023).
Our framework employs a staged approach, which helps account for the uncertainty surrounding data on battery performance, manufacturing process data, and material requirements. Table 1 shows data availability and uncertainties for TRL stages 1–4. For example, at TRL 1, material requirements, battery specific energy (Supplementary Figure S3), and process inputs for manufacturing cathodes and anodes can be uncertain. Therefore, the high-level and streamlined analysis conducted at this early research stage provides only broad directions related to the supply chain and ESJ. As more precise data become available at further TRLs, the analysis becomes more detailed. Sensitivity analysis across a range of input values mitigates uncertainty by clarifying the relative importance of different factors and the areas that require greater attention or refining. In our simplified and partial case study, analysis at TRL 1 (extraction) suggests that bromine, chlorine, and aluminum have relatively favorable supply chain and ESJ impacts. However, the picture becomes more complex at TRL 2 (refining and processing). As the potential chemistries are further developed at TRL 3 and TRL 4, the analyses would be refined, and the tradeoffs quantified and compared with greater confidence.
At early TRLs, critical technology performance data are preliminary or even theoretical. The supply chain and ESJ analyses are only as good as the available data, and comprehensive data are difficult to obtain at early TRLs. This data challenge can be addressed by analyzing the sensitivity of supply chains and ESJ impacts to uncertain battery chemistry parameters. The results may be directional rather than comprehensive during early research. There is also a need to analyze tradeoffs among technology options at various developmental stages, capturing the drawbacks and benefits of a new technology compared with incumbent technologies and other novel options. We hope our proposed framework stimulates further interest in and elaboration of analyses that integrate supply chain and ESJ considerations at the early stages of research.
Data availability statement
The data analyzed in this study is subject to the following licenses/restrictions: EcoInvent is a subscription-based dataset. NREL has a subscription to EcoInvent. Requests to access these datasets should be directed to https://ecoinvent.org/.
Author contributions
VP: Conceptualization, Data curation, Formal analysis, Methodology, Validation, Writing – original draft. JP: Data curation, Formal analysis, Writing – original draft. TG: Data curation, Formal analysis, Methodology, Writing – original draft. SA: Data curation, Formal analysis, Writing – original draft. JZ: Writing – original draft. AC: Conceptualization, Supervision, Writing – review & editing. ET: Supervision, Writing – review & editing. AM: Supervision, Writing – review & editing. KT: Writing – review & editing. AB: Funding acquisition, Writing – review & editing. MM: Conceptualization, Methodology, Project administration, Supervision, Writing – review & editing.
Funding
The author(s) declare financial support was received for the research, authorship, and/or publication of this article. This work was authored in part by the National Renewable Energy Laboratory, operated by Alliance for Sustainable Energy, LLC, for the U.S. Department of Energy (DOE) under Contract No. DE-AC36-08GO28308.
Acknowledgments
ET, AM, and KT acknowledge support from the Institute of Energy: Sustainability, Environment and Equity at Stony Brook University. ET also acknowledges support as the William and Jane Knapp Chair in Energy and the Environment. The authors also acknowledge the support received from Rebecca Martineau, Michael Deneen, and Fred Zietz from the National Renewable Energy Laboratory Communications team.
Conflict of interest
The authors declare that the research was conducted in the absence of any commercial or financial relationships that could be construed as a potential conflict of interest.
Publisher’s note
All claims expressed in this article are solely those of the authors and do not necessarily represent those of their affiliated organizations, or those of the publisher, the editors and the reviewers. Any product that may be evaluated in this article, or claim that may be made by its manufacturer, is not guaranteed or endorsed by the publisher.
Author disclaimer
The views expressed in the article do not necessarily represent the views of the DOE or the U.S. Government. The U.S. Government retains and the publisher, by accepting the article for publication, acknowledges that the U.S. Government retains a nonexclusive, paid-up, irrevocable, worldwide license to publish or reproduce the published form of this work, or allow others to do so, for U.S. Government purposes.
Supplementary material
The Supplementary material for this article can be found online at: https://www.frontiersin.org/articles/10.3389/frsus.2023.1287423/full#supplementary-material
References
Aluminium Recycling. (2023). Aluminum for future generations – sustainability. Available at: https://recycling.world-aluminium.org/fr/reperes/developpement-durable [Accessed April 23, 2023].
Arshad, F., Lin, J., Manurkar, N., Fan, E., Ahmad, A., Tariq, M.-U.-N., et al. (2022). Life cycle assessment of lithium-ion batteries: a critical review. Resour. Conserv. Recycl. 180:106164. doi: 10.1016/j.resconrec.2022.106164
Arvidsson, R., Chordia, M., and Nordelöf, A. (2022). Quantifying the life-cycle health impacts of a cobalt-containing lithium-ion battery. Int. J. Life Cycle Assess. 27, 1106–1118. doi: 10.1007/s11367-022-02084-3
Bolan, N., Hoang, S., Tanveer, M., Wang, L., Bolan, S., Sooriyakumar, P., et al. (2021). From mine to mind and mobiles – lithium contamination and its risk management. Environ. Pollut. 290:118067. doi: 10.1016/j.envpol.2021.118067
Calkins, S. (1983). The new merger guidelines and the Herfindahl-Hirschman index. Cal. L. Rev. 71:402. doi: 10.2307/3480160
Cao, W. J., Zhang, J., and Hong, L. (2020). Batteries with high theoretical energy densities. Energy Storage Mater. 26, 46–55. doi: 10.1016/j.ensm.2019.12.024
Das, J. (2023). Batteries and flow batteries-life cycle assessment in Indian conditions. Clean Techn. Environ. Policy 25, 1163–1177. doi: 10.1007/s10098-022-02431-w
DOE. (2023). Sustainable manufacturing and the circular economy. Washington, DC: U.S. Department of Energy.
ecoinvent. (2023). Ecoinvent database. Available at: https://ecoinvent.org/the-ecoinvent-database [Accessed 2023].
EPA. (2023). TSCA chemical substance inventory. Available at: https://www.epa.gov/tsca-inventory. [Accessed April 2023].
Ericson, B., Landrigan, P., Taylor, M., Frostad, J., Caravanos, J., Keith, J., et al. (2016). The global burden of lead toxicity attributable to informal used lead-acid battery sites. Ann. Global Health. 82, 686–699. doi: 10.1016/j.aogh.2016.10.015
Hirschman, A. (2018). National Power and the structure of foreign trade, 1st. Oakland, CA: University of California Press. Available at: https://www.ucpress.edu/book/9780520301337/national-power-and-the-structure-of-foreign-trade.
Huang, Y., and Li, J. (2022). Key challenges for grid-scale lithium-ion battery energy storage. Adv. Energy Mater. 12. doi: 10.1002/aenm.202202197
Huijbregts, M., Steinmann, Z., Elshout, P., Stam, G., Verones, F., Vieira, M., et al. (2016). ReCiPe2016: a harmonised life cycle impact assessment method at midpoint and endpoint level. Int. J. Life Cycle Assess. 22, 138–147. doi: 10.1007/s11367-016-1246-y
IEA. (2022a). Grid-scale storage. Available at: https://www.iea.org/reports/grid-scale-storage.
IEA. (2022b). The role of critical minerals in clean energy transitions. Paris: International Energy Agency
ILA. (2021). Lead batteries: The recycling success story. [https://ila-lead.org/lead-batteries-the-unsung-heroes-of-recycling-2877926].
ISO. (2023). ISO/DIS 59004: Circular economy – Terminology, principles and guidance for implementation. Available at: https://www.iso.org/standard/80648.html [Accessed 2023].
Liu, W., Sang, J., Chen, L., Tian, J., Zhang, H., and Palma, G. (2015). Life cycle assessment of lead-acid batteries used in electric bicycles in China. J. Clean. Prod. 108, 1149–1156. doi: 10.1016/j.jclepro.2015.07.026
Mann, M.V. (2022). Grid energy storage, supply chain deep dive assessment, U.S. Department of Energy response to executive order 14017. Washington, DC: U.S. Department of Energy.
Murdock, B., Toghill, K., and Tapia-Ruiz, N. (2021). A perspective on the sustainability of cathode materials used in lithium-ion batteries. Adv. Energy Mater. 11. doi: 10.1002/aenm.202102028
Nassar, N.T., and Fortier, S.M. (2021). Methodology and technical input for the 2021 review and revision of the U.S. critical minerals list. Reston, VA: U.S. Geological Survey.
National Academies of Sciences. (2021). Accelerating Decarbonization of the U.S. energy system. Washington, DC: National Academies Press.
Owen, J., Kemp, K., Lechner, A., Harris, J., Zhang, R., and Lebre, E. (2023). Energy transition minerals and their intersection with land-connected peoples. Nat. Sustainability. 6, 203–211. doi: 10.1038/s41893-022-00994-6
Peters, J., Maumann, M., Zimmermann, B., Braun, J., and Weil, M. (2017). The environmental impact of Li-ion batteries and the role of key parameters–a review. Renew. Sust. Energ. Rev. 67, 491–506. doi: 10.1016/j.rser.2016.08.039
Sandia National Laboratories. (2023). DOE global energy storage database. Available at: https://sandia.gov/ess-ssl/gesdb/public.
Shanika, A., Smith, J., and Segal, B. (2013). Application of life-cycle assessment to nanoscale technology: Lithium-ion batteries for electric vehicles. Washington, DC: U.S. Environmental Protection Agency.
Trahey, L. F. (2020). Energy storage emerging: a perspective from the joint Center for Energy Storage Research. PNAS 117, 12550–12557. doi: 10.1073/pnas.1821672117
Turner, J. (2022). Charged: A history of batteries and lessons for a clean energy future. Seattle: University of Washington Press.
Tuttman, M., and Litzelman, S. (2020). Why long-duration energy storage matters. Available at: https://arpa-e.energy.gov/news-and-media/blog-posts/why-long-duration-energy-storage-matters.
U.S. Congress. (2021). Division Z - energy act of 2020. Part 1* - public law 116-260-Dec. 27, 2020, consolidated appropriations act, 2021. Washington, DC: U.S. GPO
UNECE. (2023). Designing and planning for circularity. Available at: https://unece.org/circular-economy/designing-and-planning-circularity
United Nations. (2023). United Nations development of economic and social affairs, sustainable development - the 17 goals. Available at: https://sdgs.un.org/goals
White House. (2021). Building resilient supply chains, revitalizing American manufacturing, and fostering broad-based growth, 100-day reviews under executive order 14017. Washington DC: White House. Available at: https://www.whitehouse.gov/wp-content/uploads/2021/06/100-day-supply-chain-review-report.pdf.
WHO. (2017). Recycling used Lead-acid batteries: Health considerations. Geneva: WHO. Available at: https://apps.who.int/iris/handle/10665/259447.
Wood Mackenzie. (2022). The need for long duration energy storage (LDES) technologies. Available at: https://www.woodmac.com/news/opinion/the-need-for-long-duration-energy-storage-ldes-technologies.
Keywords: supply chain, environment, social justice, stationary battery, materials, analysis framework
Citation: Putsche VL, Pattany J, Ghosh T, Atnoorkar S, Zuboy J, Carpenter A, Takeuchi ES, Marschilok AC, Takeuchi KJ, Burrell A and Mann MK (2023) A framework for integrating supply chain, environmental, and social justice factors during early stationary battery research. Front. Sustain. 4:1287423. doi: 10.3389/frsus.2023.1287423
Edited by:
Sharfuddin Khan, University of Regina, CanadaReviewed by:
Abhijeet Parvatker, Sphera Solutions Inc., United StatesCopyright © 2023 Putsche, Pattany, Ghosh, Atnoorkar, Zuboy, Carpenter, Takeuchi, Marschilok, Takeuchi, Burrell and Mann. This is an open-access article distributed under the terms of the Creative Commons Attribution License (CC BY). The use, distribution or reproduction in other forums is permitted, provided the original author(s) and the copyright owner(s) are credited and that the original publication in this journal is cited, in accordance with accepted academic practice. No use, distribution or reproduction is permitted which does not comply with these terms.
*Correspondence: Margaret K. Mann, TWFyZ2FyZXQuTWFubkBucmVsLmdvdg==