- 1Multidisciplinary Graduate Program in Environmental Sciences, Autonomous University of San Luis Potosí, San Luis Potosí, Mexico
- 2Interdisciplinary Professional Unit of Engineering, Instituto Politécnico Nacional, Environmental Engineering, Zacatecas, Mexico
- 3Graduate Studies and Research Center, Faculty of Engineering, Autonomous University of San Luis Potosí, San Luis Potosí, Mexico
This work aimed to evaluate the devilfish bone char as an alternative material to be used as a bioanode in a microbial fuel cell (MFC) applied in bioenergy production from the pantoprazole biodegradation. The devilfish is an invasive species in Mexico and other countries, so its control and eradication are based on the integral use of its biomass. In addition, the bones are unusable waste and could be used as a raw material to manufacture the bioanode. Two MFCs with an anaerobic anode single chamber of 125 mL were operated independently using the fabricated bioanode and the carbon felt (conventional anode). Both MFCs were fed with 1 g/L of pantoprazole as substrate with a chemical oxygen demand (COD) of 210 ± 1.3 mg/L and 1.2 g of soil as inoculum. The two MFCs were characterized electrochemically, and the COD removal percentage was measured as an indicator of the pantoprazole biodegradation. The results showed that the MFC operated with the bioanode had a better performance than the conventional anode, since it reached a maximum power density of 1.22 mW/m2, being 156% higher than carbon felt. These values were consistent with the COD removal percentages reached in 10 days with values of 47.17 ± 0.23 and 43.34 ± 0.41%, respectively. Therefore, these findings provide a low-cost alternative carbonaceous material for the sustainable construction of MFC applied to produce bioenergy from the biodegradation of pharmaceutical products.
Introduction
Microbial fuel cells (MFCs) are bioelectrochemical processes that aim to produce bioenergy (electricity) by using the electrons derived from biochemical reactions catalyzed by bacteria (Hamouda and El-Naggar, 2021). Generally, the MFC contains an anode and a cathode, separated by a proton exchange membrane. The anode is kept under anaerobic conditions (without oxygen) and the cathode under aerobic conditions (with oxygen from the air). In the anode chamber, bacteria adhere to the anode, generate electrons by substrate oxidation, and transfer the electrons to the anode (Yaqoob et al., 2021). The electrons are transferred to the cathode across the external circuit. The protons are transferred across the proton exchange membrane. The electrons and protons are spent in the cathode chamber, reducing oxygen to water (Ahmed et al., 2022). The surface characteristics of the anode directly affect the microbial adhesion and, therefore, the electrical performance of MFC. Increased specific surface area and more positive charge on the electrode surface, which interact with negatively charged bacteria, aid the microbial adhesion and electron transfer on the anode surface (Yaqoob et al., 2021). Thus, a material with these characteristics should be selected to improve the performance of bioenergy production in the MFC.
Carbon felt, carbon cloth, and carbon brushes are preferred materials that are used as electrode materials (Isaacs-Páez et al., 2020). However, they tend to be more expensive in the MFC, so alternative materials based on biomass are of interest. Animal waste (bone) has been transformed by calcination into bone chars. These materials have been explored as electrode materials for bioanodes in MFC, being selected due to their high surface area and surface roughness. Both properties facilitate the bacterial colonization of the anode and increase the possibility of its attachment to it (Isaacs-Páez et al., 2020; Aguilera Flores et al., 2021a,b). Bioanodes from devilfish bone char have been explored to produce bioenergy from the biodegradation of ibuprofen and carbamazepine in MFC (Aguilera Flores et al., 2021a,b). In these works, devilfish bone char bioanodes showed a better MFC performance than when used carbon felt. MFC with the bioanodes showed higher bioenergy production and higher substrate biodegradation. These findings show that these materials must continue to be studied as low-cost alternative materials to produce bioenergy from the biodegradation of pharmaceutical products using MFC technology.
In this context, this work aimed to evaluate the performance of a bioanode fabricated from devilfish bone char in pantoprazole biodegradation and bioenergy production. Pantoprazole is one of the twenty-selling drugs globally. It is a proton pump inhibitor used in intensive care unit (ICU) patients to reduce the risk of stress ulcers. In veterinary medicine, it has been recognized as the most potent suppressor of gastric acid (Cox et al., 2022). Pantoprazole and other drugs have been listed as emerging pollutants in the water because they are partially or inefficiently removed by the conventional technologies used in wastewater treatment plants. These pollutants are characterized by being detected at concentrations between 1 ng/L and 1 μg/L, their toxicological significance is difficult to assess, and accepted concentration limits for drinking water, wastewater, and treated water have not yet been stipulated internationally (Gogoi et al., 2018). When these drugs enter the human body, they could disturb hormonal, enzymatic, and genetic activities (Hube and Wu, 2021; Neha et al., 2021; Zhong et al., 2022). In humans, multiple adverse effects are possible from the consumption of pantoprazole, including hematologic and electrolyte abnormalities, anaphylaxis, and edema. Hypomagnesemia is observed in cattle, goats, and sheep when administered pantoprazole (Smith et al., 2020). Hence, the presence of pantoprazole in water could cause health risks. In addition, it should be noted that the authors have not found reports on the use of MFC technology applied to the biodegradation of pantoprazole, so its study shows an opportunity area.
Materials and methods
Raw material
The non-live devilfish samples were provided by fishers from Usumacinta River, Tabasco, Mexico. Approximately 300 g of devilfish bones were obtained. The bones were boiled for 1 h in approximately 1.7 L of a solution of deionized water and concentrated hydrogen peroxide with a volume ratio of 6:1, respectively. This step was performed to remove the organic matter. Then, the bones free of organic matter were put in an oven at 70 °C for 24 h to remove the moisture. Later, devilfish bones were calcined in an air atmosphere with a flow rate of 15 mL/min at 600 °C and a heating rate of 10 °C/min for 2 h to obtain a bone char. Finally, the bone char was sieved to an average particle diameter of 0.79 mm and was stored in refrigeration until use.
Characterization of devilfish bone char and carbon felt
The specific area of the devilfish bone char and the carbon felt was determined by nitrogen adsorption using Micromeritics Mod. ChemiSoft TPx equipment in the relative pressure range from 0.05 to 0.2 using the BET method. Both materials were used as electrode materials in the MFC. The surface morphology of both materials was characterized by environmental scanning electron microscopy (ESEM) before and after the bacterial colonization and biofilm formation during the MFC operation, using an FEI Quanta 250 FEG microscope.
Preparation of the bioanode and carbon felt
Devilfish bone char was used to fabricate the bioanode. A mixture of 0.5 g of devilfish bone char, 800 mL of Nafion®, and 200 mL of ethanol was made to obtain an ink. This ink was adhered on both sides of a stainless-steel mesh with a square geometric area of 18.58 cm2 (Figure 1A). A total area of 37.16 cm2 was considered the electrode projected area. The same projected area was used for carbon felt. Carbon felt was thermally treated with a solution of concentrated HNO3 and 30% H2O2 of 1:1 v/v, respectively (Zhao et al., 2018). Carbon felt was used as reference material for this study since it is a conventional anode commonly used in MFC.
MFC operation
Two cubic-shaped single-chamber MFCs were constructed with a total cell volume of 125 mL, maintaining the anodic chamber under anaerobic conditions (Figure 1B). Carbon Cloth Electrode (with 0.5 mg/cm2 60% Platinum) was used as cathode with similar geometry to the anodes, Nafion® as proton exchange membrane, and the devilfish bone char bioanode and carbon felt were used as anodes of independent form in each cell. Stainless steel current collectors were connected by direct contact on both electrodes. The external circuit was connected to a constant external resistance of 100 Ω. Each cell was fed with 1 g/L of reagent-grade pantoprazole (substrate), 1.5 g of activated soil (inoculum), and a potassium phosphate buffer solution to maintain a neutral pH and fill the cell volume. The soil was used as inoculum because it contains a bacterial species diversity (microbial consortium). Some authors have reported its use as inoculum in MFC since it is easily accessible (Kumar et al., 2016). The soil was heated at 40 °C in approximately 125 mL of the mentioned buffer solution. The heating allowed the activation of the microorganisms present in the soil sample. Finally, the cells were operated on for 10 days at room temperature. This time was determined because the cells showed a lower potential on this day (day 10) on the day the operation began (day 0). This potential fall is associated with cell death or a substrate deficit state where the microorganisms consumed a maximum substrate amount. Therefore, adding inoculum or substrate (pantoprazole) is required to maintain or increase the potential (Lóránt et al., 2019). This study used a batch system where no extra feeding is used from the beginning to the end of the process. Hence, the highest percentage of COD removal achieved in MFC could be associated with the biodegradation of pantoprazole since this compound was used as the unique carbon source (feed) for the microorganisms.
MFC electrochemical characterization
The MFC electrochemical characterization was performed under the electrochemical techniques: linear sweep voltammetry and electrochemical impedance spectroscopy. The first was carried out with a scan rate of 0.001 V/s and a potential range from 0.65 to 0.05 V and from 0.60 to 0.05 V for the MFC operated with the devilfish bone char bioanode and the carbon felt, respectively. These values (0.65 and 0.60 V) corresponded to the maximum potential achieved in the cells during the operation days. The second one was conducted in the frequency range of 10 kHz to 0.1 Hz with a potential amplitude of 10 mV and was conducted under the open-circuit condition. The Nyquist plot was fitted with a modified Randles equivalent circuit using the EIS Spectrum Analyzer program to get the impedance parameters.
COD removal
The COD was measured before and after MFC operation using a GENESYSTM 10S Vis Spectrophotometer and based on the test method stipulated by the Mexican Standard to determine the COD in natural water, wastewater, and treated water (Mexican Standard, 2001). The percentage of COD removal was calculated by Equation (1).
where CODiand CODf are the COD estimated at the beginning and end of the MFC operation in mg/L.
Results and discussion
Electrode materials properties and micrographs
Table 1 shows the specific area of the devilfish bone char and the carbon felt used in this work as electrode materials. Likewise, data reported for some materials used as anodes in MFC are shown.
It can be observed that the specific area of the devilfish bone char calcined in this work (Table 1) is within the range reported in works previously. Likewise, devilfish bone char has a specific area from 1.3 to 1.5 times higher than cattle bone char (Table 1). The properties of the bone char (as a specific area) depend on various factors, such as heating rate, calcination temperature, residence time, and purge gas, among others (Alkurdi et al., 2019). In this work, the calcination process was performed using an air atmosphere, and the study of Aguilera Flores et al. (2021a) used nitrogen as a gas purge. Hence, the nitrogen increased the bone char surface area, being 1.14 times higher.
On the contrary, the calcination process in this study was performed under specific conditions without searching for the optimal conditions. Now, a specific area of carbon felt between 0.6 and 3.2 m2/g has been reported (Kaur et al., 2022). The value shown in Table 1 is 1.3 times higher than the upper value reported by Kaur et al. (2022). This result is associated with the treatment applied to carbon felt described in Section Preparation of the bioanode and carbon felt. However, it is noted that the specific area of devilfish bone char is approximately 33 times higher than carbon felt, according to the results obtained in this study. Hence, it is speculated that devilfish boner char will perform better than carbon felt when used as bioanode in the MFC. This condition is attributed to the high specific area where more bacteria could interact with the material, aiding the bacterial adhesion and electron transfer on the anode surface (Yaqoob et al., 2021). Figure 2 shows the micrographs of the electrode materials obtained before and after the bacterial colonization of the electrode materials.
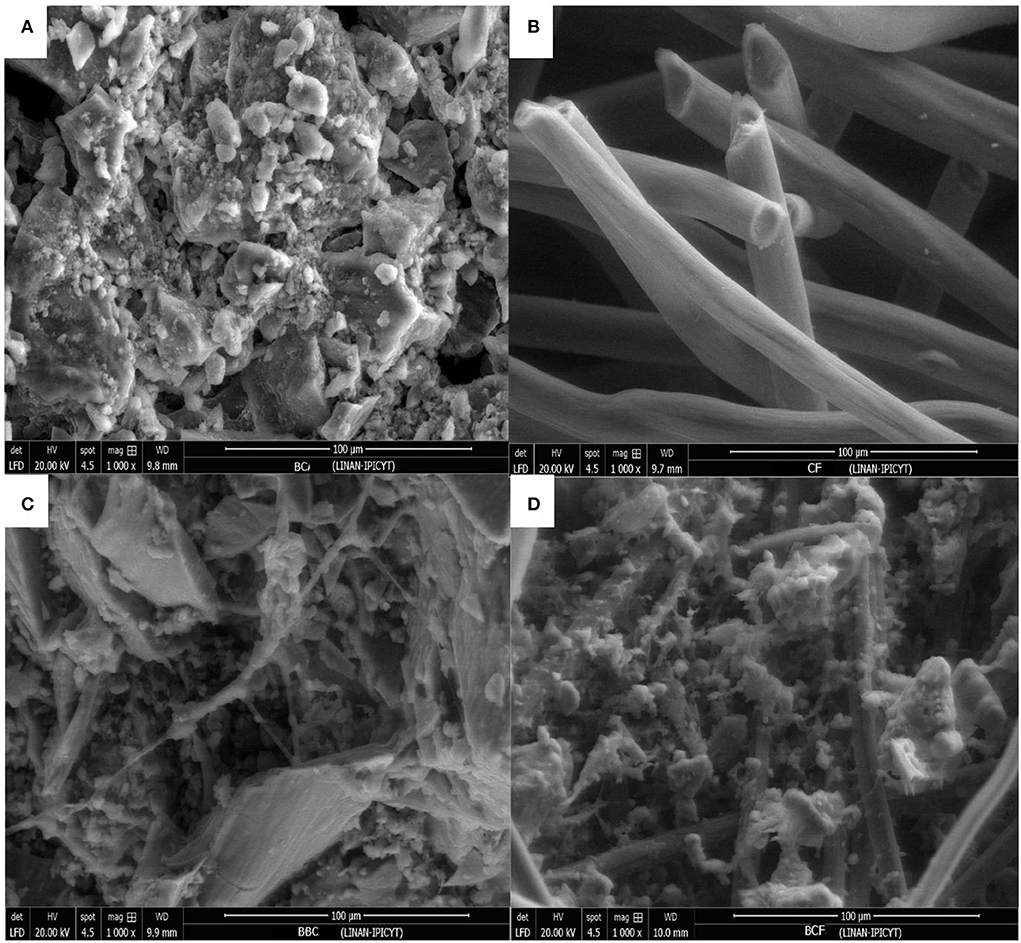
Figure 2. Scanning electron micrographs: (A) devilfish bone char, (B) carbon felt, (C) biofilm formed on char devilfish bone char, and (D) biofilm formed on carbon felt.
Figure 2A shows an irregular and rough structure, which characterizes the bone chars. Its roughness could provide more space for bacterial attachment (Isaacs-Páez et al., 2020). Figure 2B shows the filamentous structure of the carbon felt. Figures 2C,D show the bacterial colonization and biofilm formation on the electrodes. It can be noted that biofilm layers cover the materials.
Electrochemical measures
The output power densities (PD) of the cells are shown in Figure 3. The PD gradually increased till attaining the peak with the increase in current density and then decreased for both cells. The output maximum power densities of the cells with devilfish bone char and carbon felt were 1.22 and 0.78 mW/m2, respectively. MFC with devilfish bone char showed a value of 156% higher than MFC with carbon felt. These results are like those reported in other works where the devilfish bone char was used as bioanode in MFC to biodegrade other drugs. When the cells were fed with ibuprofen, MFC with the bioanode achieved an output maximum power density of 4.26 mW/m2 (175% higher carbon felt) (Aguilera Flores et al., 2021b), and with carbamazepine, 5.40 mW/m2 (210% higher than carbon felt) (Aguilera Flores et al., 2021a). Thus, the results prove the better performance obtained in the MFC when the devilfish bone char is used as bioanode, compared to carbon felt, since a higher power density (bioenergy) is obtained. It could be associated with the high specific area of the devilfish bone char where more electrochemically active bacteria could have the opportunity to adhere to the bioanode forming the biofilm.
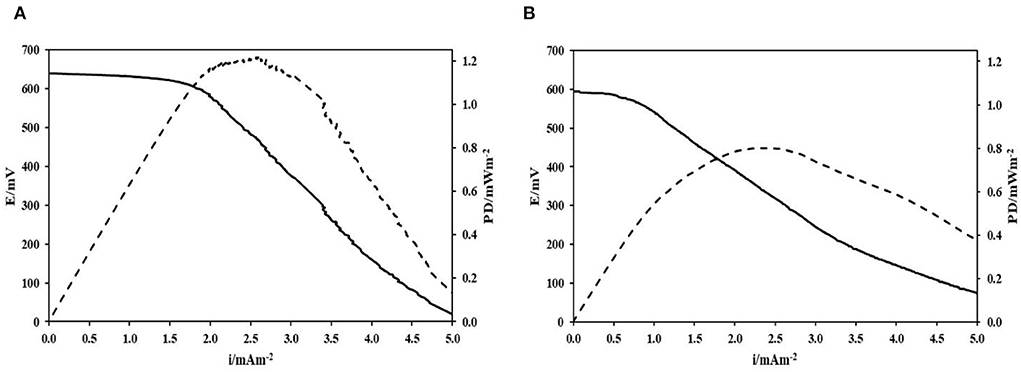
Figure 3. Power and polarization curves of the MFC: (A) devilfish bone char bioanode, (B) carbon felt (Dotted line, power curve; solid line, and polarization curve).
On the one hand, some authors reported a power density value of 0.119 mW/m2 in an MFC using PANi@CNTs/SS as bioanode to biodegrade 10 mg/L of ibuprofen at 40 h (Xu et al., 2018). This value is 10.25 times lower than obtained in this work when devilfish bone char is used as bioanode. On the other hand, other authors reported a value of 29.12 mW/m2 to treat pharmaceutical sewage using MFC at 5 h (Chang et al., 2018). This value is 23.86 times higher than this work. Multiple factors make these differences because each cell is operated with designs and configurations different (type and amount of inoculum and substrate, type of electrode materials, and cell volume, among others).
The Nyquist plots are shown in Figure 4. The experimental spectra fit into a modified Randles equivalent circuit to quantitatively estimate the impedance data. The equivalent circuit consisted of a solution resistance (Rs) with two circuits connected in series. The first one composes of the film capacitance (Cf) connected in parallel with the film resistance (Rf) and Warburg diffusion resistance (Zw). The second one comprises the double-layer capacitance (Cdl) connected in parallel with the charge transfer resistance (Rct). Impedance data settings of the MFC are shown in Table 2.
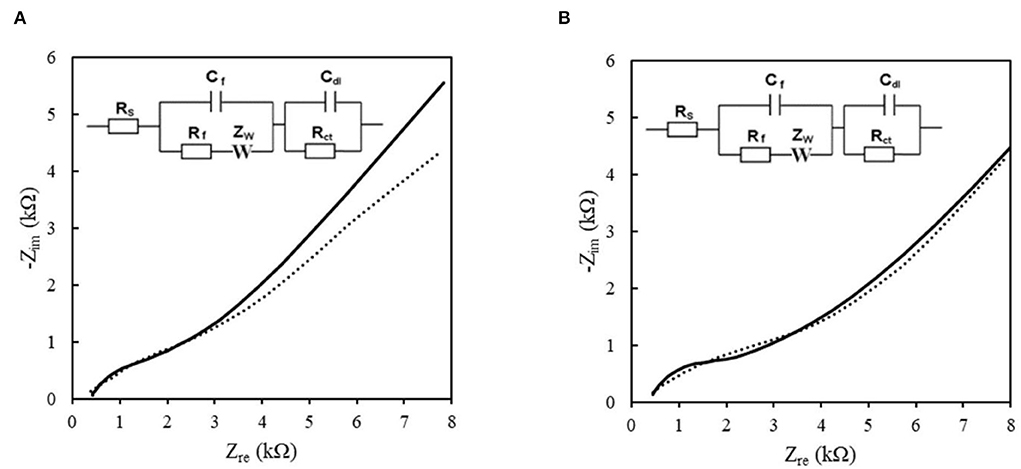
Figure 4. Nyquist plots of the MFC: (A) devilfish bone char bioanode, (B) carbon felt (Dotted line, experimental curve; solid line, and fitting curve).
Rs is associated with the electrical contacts and solution resistances. The values of Rs were very similar for both cells. These values could be considered higher than those reported by other authors (Aguilera Flores et al., 2021a,b). However, these resistances could be decreased by improving the cell architecture. The distance between the anode and the cathode could be reduced. Now, MFC with devilfish bone char bioanode had a value of Zw 1.25 times higher carbon felt, which means that the resistance offered by the system due to the diffusion of species to the interface is higher when used bioanode.
Nevertheless, MFC with carbon felt had Cdl 1.14 times higher value than devilfish bone char bioanode. These values reflect the electrochemical double-layer capacitance at the biofilm surface, where MFC with carbon felt stores more energy. Depending on film thickness and ion mobility, Rf and Cf are multilayer resistance and capacitance. Although MFC with devilfish bone char showed a higher value of Cf (2.58 times) and a lower value of Rf (1.60 times) than carbon felt, the Rct of the MFC with carbon felt is 1.67 times higher than devilfish bone char bioanode, so the last one is more conductive, transferring the electrons from anode to cathode more easily. This issue is proved with the maximum power densities obtained so that MFC with devilfish bone char showed a value of 156% higher than carbon felt.
COD removal efficiency
Table 3 shows the efficiencies of COD removal obtained in this work and other studies. Both cells had an initial value of 210 ± 1.3 mg/L, achieving, after 10 days, the COD removal efficiencies shown in Table 3. The COD removal efficiency using devilfish bone char bioanode was slightly higher (1.09 times) than carbon felt. The reduction in COD suggests that the bacteria used pantoprazole as a carbon source and produced bioenergy from its biochemical reactions (Hamouda and El-Naggar, 2021).
The chemical structure of pantoprazole, ibuprofen, and carbamazepine is C16H15N3F2O4S, C13H18O2, and C15H12N2O, respectively. Microorganisms perform biodegradation by breaking down these complex organic compounds into simpler molecules like carbon dioxide and water (Nyirenda et al., 2020). However, as already mentioned, the used inoculum type is essential since the microorganisms have an affinity for different substrates to be used as carbon sources.
Some authors reported COD removal efficiencies higher than this work (Liu et al., 2012; Sharma et al., 2021). These differences are associated with the used substrate amount and cell operation time. Liu et al. (2012) used an amount of substrate 6.72 times higher than this work. However, the MFC was operated on for 3 days. Sharma et al. (2021) operated the MFC for 200 days more than this study.
Nevertheless, they used an amount of substrate 500 times lower than this study (Table 3). Therefore, it is not easy to compare the performance of different MFC. The authors suggest that a comparison could be made by relating the fraction of electrical energy generated in the MFC to the biodegradation of a determined substrate (Luo et al., 2010).
An analysis of the final concentration of pantoprazole by HPLC resided outside the scope of this study. However, this work's bioenergy production and COD removal suggest pantoprazole biodegradation. The authors reported that ibuprofen and carbamazepine biodegradation efficiencies of 34 and 78% corresponded to 44 and 84% COD removal (Aguilera Flores et al., 2021a,b). Taking the values and analogy with the value of COD removal obtained in this work, a pantoprazole biodegradation efficiency in the range of 40–50% would be expected using devilfish bone char bioanode in the MFC. The three studies used activated soil as inoculum, having a microbial consortium. Therefore, the bacteria present in the soil mainly use carbon source carbamazepine, pantoprazole, and ibuprofen. Substrate, inoculum, and electrode types are essential factors determining MFC performance. Therefore, the study of testing other inoculum, substrates, and electrodes to improve MFC performance is still an area of opportunity. However, it is shown in this work that it is possible to achieve the bioenergy production from the pantoprazole biodegradation in the MFC operated and even demonstrates that low-cost alternative materials like devilfish bone char can be used as bioanodes.
Conclusion
Devilfish bone char showed morphological and textural properties suitable for bacterial colonization and biofilm formation on their surface. This material had a better performance when used as bioanode than carbon felt (conventional anode) in MFC. The cell with devilfish bone char bioanode showed a maximum power density of 1.22 mW/m2 (156% higher than carbon felt) and a COD removal percentage of 47.17 ± 0.23% (109% higher than carbon felt) in 10 days, using pantoprazole as substrate. Therefore, these findings confirm the use of devilfish bone char as low-cost carbonaceous materials employed as bioanodes for the sustainable construction of MFC applied to produce bioenergy from the biodegradation of pharmaceutical products.
Data availability statement
The raw data supporting the conclusions of this article will be made available by the authors, without undue reservation.
Author contributions
MA, VA, and NM contributed to the conception and design of the study. MA performed the formal analysis, investigation, and wrote the first draft of the manuscript. VA and NM supervised the investigation. All authors contributed to manuscript revision and read and approved the submitted version.
Funding
This work was supported by Consejo Nacional de Ciencia y Tecnología, CONACyT, Mexico, under grant Nos. CONACyT CB-286990-2016 and CONACyT PN-3947-2016.
Acknowledgments
MA would like to thank the Comité Técnico para el Otorgamiento de Becas, Estudio, Apoyos Económicos y Licencias con Goce de Sueldo (COTEBAL) of the Instituto Politécnico Nacional (IPN) for the paid leave granted in the years 2020, 2021, and 2022. We also thank Dra. Gladis Judith Labrada Delgado of the Potosino Institute of Scientific and Technological Research (IPICYT) and Dr. Sergio Miguel Durón Torres of the Autonomous University of Zacatecas (UAZ) for the technical support for carrying out the experimental tests of the project.
Conflict of interest
The authors declare that the research was conducted in the absence of any commercial or financial relationships that could be construed as a potential conflict of interest.
Publisher's note
All claims expressed in this article are solely those of the authors and do not necessarily represent those of their affiliated organizations, or those of the publisher, the editors and the reviewers. Any product that may be evaluated in this article, or claim that may be made by its manufacturer, is not guaranteed or endorsed by the publisher.
References
Aguilera Flores, M. M., Ávila Vázquez, V., Medellín Castillo, N. A., Cardona Benavides, A., Carranza Álvarez, C., and Ocampo Pérez, R. (2021a). Biodegradation of carbamazepine and production of bioenergy using a microbial fuel cell with bioelectrodes fabricated from devil fish bone chars. J. Environ. Chem. Eng. 9, 106692. doi: 10.1016/j.jece.2021.106692
Aguilera Flores, M. M., Ávila Vázquez, V., Medellín Castillo, N. A., Carranza Álvarez, C., Cardona Benavides, A., Ocampo Pérez, R., et al. (2021b). Ibuprofen degradation and energy generation in a microbial fuel cell using a bioanode fabricated from devil fish bone char. J. Environ. Sci. Health, Part A 56, 874–885. doi: 10.1080/10934529.2021.1934357
Ahmed, S. F., Mofijur, M., Islam, N., Parisa, T. A., Rafa, N., Bokhari, A., et al. (2022). Insights into the development of microbial fuel cells for generating biohydrogen, bioelectricity, and treating wastewater. Energy 254, 124163. doi: 10.1016/j.energy.2022.124163
Alkurdi, S. S. A., Al-Juboori, R. A., Bundschuh, J., and Hamawand, I. (2019). Bone char as a green sorbent for removing health threatening fluoride from drinking water. Environ. Int. 127, 704–719. doi: 10.1016/j.envint.2019.03.065
Chang, T. J., Chang, Y. H., Chao, W. L., Jane, W. N., and Chang, Y. T. (2018). Effect of hydraulic retention time on electricity generation using a solid plain-graphite plate microbial fuel cell anoxic/oxic process for treating pharmaceutical sewage. J. Environ. Sci. Health. Part A. 53, 1185–1197. doi: 10.1080/10934529.2018.1530338
Cox, S., Harvill, L., Bullock, S., Smith, J., and Bergman, J. (2022). Validation of a method for pantoprazole and its sulfone metabolite in goat plasma using high performance liquid chromatography. J. Chromatogr. Open 2, 100038. doi: 10.1016/j.jcoa.2022.100038
Gogoi, A., Mazumder, P., Tyagi, V. K., Tushara Chaminda, G. G., An, A. K., and Kumar, M. (2018). Occurrence and fate of emerging contaminants in water environment: a review. Groundw. Sustain. Dev. 6, 169–180. doi: 10.1016/j.gsd.2017.12.009
Hamouda, R. A., and El-Naggar, N. E.-A. (2021). “Cyanobacteria-based microbial cell factories for production of industrial products,” in Microbial Cell Factories Engineering for Production of Biomolecules, ed. V. Singh (Amsterdam, NL: Elsevier B.V.) 277–302. doi: 10.1016/B978-0-12-821477-0.00007-6
Hube, S., and Wu, B. (2021). Mitigation of emerging pollutants and pathogens in decentralized wastewater treatment processes: A review. Sci. Total Environ. 779, 146545. doi: 10.1016/j.scitotenv.2021.146545
Isaacs-Páez, E. D., Medellín-Castillo, N., Manríquez-Guerrero, F., and Cercado, B. (2020). Characterization of bone char and carbon xerogel as sustainable alternative bioelectrodes for bioelectrochemical systems. Waste Biomass Valorization 11, 4885–4894. doi: 10.1007/s12649-019-00817-4
Kaur, A., Jeong, K. I., Kim, S. S., and Lim, J. W. (2022). Optimization of thermal treatment of carbon felt electrode based on the mechanical properties for high-efficiency vanadium redox flow batteries. Compos. Struct. 290, 115546. doi: 10.1016/j.compstruct.2022.115546
Kumar, R., Singh, L., and Zularisam, A. W. (2016). Exoelectrogens: Recent advances in molecular drivers involved in extracellular electron transfer and strategies used to improve it for microbial fuel cell applications. Renew. Sustain. Energy Rev. 56, 1322–1336. doi: 10.1016/j.rser.2015.12.029
Liu, R., Gao, C., Zhao, Y.-G., Wang, A., Lu, S., Wang, M., et al. (2012). Biological treatment of steroidal drug industrial effluent and electricity generation in the microbial fuel cells. Bioresour. Technol. 123, 86–91. doi: 10.1016/j.biortech.2012.07.094
Lóránt, B., Gyalai-Korpos, M., Goryanin, I., and Tardy, G. M. (2019). Single chamber air-cathode microbial fuel cells as biosensors for determination of biodegradable organics. Biotechnol. Lett. 41, 555–563. doi: 10.1007/s10529-019-02668-4
Luo, Y., Zhang, R., Liu, G., Li, J., Li, M., and Zhang, C. (2010). Electricity generation from indole and microbial community analysis in the microbial fuel cell. J. Hazard. Mater. 176, 759–764. doi: 10.1016/j.jhazmat.2009.11.100
Mexican Standard. (2001). NMX-AA-030-SCFI-2001. Test method to determine the chemical oxygen demand in natural water, wastewater and treated water. Available online at: https://agua.org.mx/wp-content/uploads/2011/01/nmx-aa-030-scfi-2001.pdf (accessed January 12, 2022).
Neha, R., Adithya, S., Jayaraman, R. S., Gopinath, K. P., M, P., L, P., et al. (2021). Nano-adsorbents an effective candidate for removal of toxic pharmaceutical compounds from aqueous environment: A critical review on emerging trends. Chemosphere 272, 129852. doi: 10.1016/j.chemosphere.2021.129852
Nyirenda, J., Mwanza, A., and Lengwe, C. (2020). Assessing the biodegradability of common pharmaceutical products (PPs) on the Zambian market. Heliyon 6, e05286. doi: 10.1016/j.heliyon.2020.e05286
Sharma, P., Kumar, D., and Mutnuri, S. (2021). Probing the degradation of pharmaceuticals in urine using MFC and studying their removal efficiency by UPLC-MS/MS. J. Pharm. Anal. 11, 320–329. doi: 10.1016/j.jpha.2020.04.006
Smith, J. S., Kosusnik, A. R., and Mochel, J. P. (2020). A Retrospective Clinical Investigation of the Safety and Adverse Effects of Pantoprazole in Hospitalized Ruminants. Front. Vet. Sci. 7, 97. doi: 10.3389/fvets.2020.00097
Xu, H., Quan, X., Xiao, Z., and Chen, L. (2018). Effect of anodes decoration with metal and metal oxides nanoparticles on pharmaceutically active compounds removal and power generation in microbial fuel cells. Chem. Eng. J. 335, 539–547. doi: 10.1016/j.cej.2017.10.159
Yaqoob, A. A., Ibrahim, M. N. M., and Guerrero-Barajas, C. (2021). Modern trend of anodes in microbial fuel cells (MFCs): An overview. Environ. Technol. Innovation 23, 101579. doi: 10.1016/j.eti.2021.101579
Zhao, Y., Ma, Y., Li, T., Dong, Z., and Wang, Y. (2018). Modification of carbon felt anodes using double-oxidant HNO3/H2O2 for application in microbial fuel cells. RSC Adv. 8, 2059–2064. doi: 10.1039/C7RA12923H
Keywords: bioenergy, devilfish bone char bioanode, microbial fuel cells, pantoprazole, bioanode
Citation: Aguilera Flores MM, Avila Vázquez V and Medellín Castillo NA (2022) Devilfish bone char, an alternative material to be used as bioanode in microbial fuel cells. Front. Sustain. 3:940038. doi: 10.3389/frsus.2022.940038
Received: 09 May 2022; Accepted: 30 September 2022;
Published: 20 October 2022.
Edited by:
Laxman Singh, Siddharth University Kapilvastu, IndiaReviewed by:
Tirath Raj, Yonsei University, South KoreaJose Paixão Coelho, Lisbon Higher Institute of Engineering (ISEL), Portugal
Copyright © 2022 Aguilera Flores, Avila Vázquez and Medellín Castillo. This is an open-access article distributed under the terms of the Creative Commons Attribution License (CC BY). The use, distribution or reproduction in other forums is permitted, provided the original author(s) and the copyright owner(s) are credited and that the original publication in this journal is cited, in accordance with accepted academic practice. No use, distribution or reproduction is permitted which does not comply with these terms.
*Correspondence: Miguel Mauricio Aguilera Flores, bWFndWlsZXJhZkBpcG4ubXg=; bW1hZjFAaG90bWFpbC5jb20=; Verónica Avila Vázquez, dmF2aWxhQGlwbi5teA==