- 1Department of Sustainable Development, Environmental Sciences and Engineering SEED, KTH Royal Institute of Technology, Stockholm, Sweden
- 2Department of Industrial Economics and Management INDEK, KTH Royal Institute of Technology, Stockholm, Sweden
Ambitious fossil-free targets imposed on the aviation industry worldwide demand a large volumetric supply of sustainable aviation fuel (SAF) to meet. Sweden's commitment to a 30% volume SAF blending target by 2030 attracts interest in local production. However, the sustainability of local production is largely unknown. Addressing this gap, we aim to explore potential SAF technology pathways and assess their environmental performances in Sweden. To do so, we utilize a socio-technical system (STS) approach for pathways selection and prospective life cycle assessment (LCA) for environmental impact assessment. As a result, we identify two lignocellulosic-based and two electrofuel-based pathways and evaluate their global warming potential, mineral depletion potential, ionizing radiation, land use, freshwater ecotoxicity and human toxicity impact in comparison to jet fuel. Our findings show that the well-to-wake global warming potential (100 years) of 30% SAF is on average 20% lower than that of jet fuel, with non-carbon dioxide species emitted in flight being the major contributors, prompting the need for urgent research efforts to mitigate their potential impacts. Under the assumption that no burdens are allocated to waste material used as feedstock, lignocellulosic-based 100% SAF has a well-to-pump climate impact (100 years) ranging from 0.6 to 1.5 g CO2−eq/MJ compared to jet fuel's 10.5 g CO2−eq/MJ. In contrast, the well-to-pump climate impact (100 years) of electrofuel-based 100% SAF (ranging from 7.8 to 8.2 g CO2−eq/MJ) is only marginally lower than that of jet fuel, mainly attributed to emissions from steel and concrete produced for wind turbine manufacturing. In general, the use of electricity generated by wind power could shift the potential environmental burden associated with jet fuel from global warming to mineral depletion, land use, freshwater ecotoxicity and human toxicity. The STS approach underscores the need to prioritize changes in systems underpinning SAF production, in turn supporting policy and investment decision making.
Introduction
Aviation is one of the fastest-growing GHG emitters (EU, 2021c). Thus, reducing aviation-induced climate impact is in the utmost interest of the European Union (EU) (EU, 2021c) as well as the international aviation community (ICAO, 2019). Sustainable aviation fuels (SAF) is a common name for advanced biofuels or renewable fuels of non-biological origin, i.e., electrofuel produced from renewable electricity, that can function as alternatives to jet fuel (EU, 2018; Pavlenko and Searle, 2021). Ambitious targets for fossil-free aviation have boosted interest in SAF worldwide. In their recent suggestion for a level playing field for sustainable air transport, the EU proposed to oversee that every liter of jet fuel supplied to the EU airports from 2030 must be blended with at least 2% of SAF (EU, 2021a). The foundation of the proposal was built upon the belief that large-scale production and supply of SAF can be achieved in the EU at competitive prices (EU, 2021a). For that matter, the proposal articulated its support for the use of existing SAF production capacity and the development of new additional SAF facilities in the EU (EU, 2021a).
Sweden, an EU member state, imposed a 30% volume SAF blending target by 2030 (Regeringskansliet, 2020), a mandate that was far more ambitious than the EU proposal. An initial estimate (Soone, 2020) projected that an overall SAF production in the EU in 2030 would meet ~6% of the anticipated jet fuel volume. In other words, to meet its national target, it may be necessary for Sweden to look beyond SAF import and plan for local production. Depending on the feedstock types and modeling choices, previous studies suggested that SAF could have a life cycle emission of between −222 g CO2−eq/MJ and 698 g CO2−eq/MJ (Kolosz et al., 2020; Prussi et al., 2021), compared to the average life cycle jet fuel baseline of 89 g CO2−eq/MJ (Prussi et al., 2021). The question is whether Sweden can deliver SAF with superior life cycle environmental performance as opposed to jet fuel in 2030? To address this question, it is essential to investigate what technologies are relevant for SAF production in the Swedish context, and how to assess their potential environmental impacts in future times.
Typically, a SAF technology pathway consists of three stages: feedstock supply, feedstock conversion and fuel synthesis. The selection of SAF technology pathways can be constrained by multiple factors, including feedstock types, aviation fuel production standards, technological limitations, technology readiness level (TRL), cost etc. In O'Malley et al.'s (2021) recent study of SAF production in Europe, they emphasized not only how feedstock could dictate the technology pathway, but also the importance of understanding its interdependencies with existing markets in which feedstock displacement effects could undermine the climate mitigation potential of SAF. They exemplified such a concept by elaborating that the potential consequence of diverting all wheat residue to SAF production could be the substitution of wheat resources formerly used for livestock fodder and bedding (O'Malley et al., 2021). To understand why certain feedstock is better suited for future SAF production in Sweden, and which interdependencies can occur among feedstock, market environment, institutions and other industrial sectors, we adopt a socio-technical system (STS) perspective to identify potential technology pathways. STS can be defined as assemblages of (networks of) technologies, actors (e.g., individuals, firms, and other types of organizations) and institutions (e.g., norms, regulations, and standards), embedded in material, political, economic, geographical, and socio-cultural aspects (Markard et al., 2012). In this paper, we conceptualize the Swedish aviation sector as a STS and SAF supply as a sub-segment of the STS and attempt to explore the relevant SAF technology pathways with some resemblance to flat ontology (Garud and Gehman, 2012). Under a flat ontological assumption, we consider technologies, actors, and institutions as mutually constitutive agents, flatting the distinction between human and non-human agents, i.e., assuming there are no hierarchical differences among agents and all entities are equally important (Garud and Gehman, 2012).
Technologies that are in early development stages but are fast-growing and gaining importance, such as those enabling SAF feedstock production, feedstock conversion and fuel synthesis (Mawhood et al., 2016; Morgan et al., 2019; Wei et al., 2019; EU, 2021a), are defined as emerging technologies (Rotolo et al., 2015; Cucurachi et al., 2018). To study the potential environmental impact of the projected future of emerging technologies and their large-scale implementation, Cucurachi et al. (2018) suggested the use of prospective life cycle assessment (LCA), a combination of futures scenarios and LCA. LCA is a quantitative decision support tool that is used to systematically assess the environmental impact of a product or service over its entire life cycle. It quantifies the emissions to the environment into different impact categories (Hauschild et al., 2018). In Cucurachi et al.'s (2018) opinion, prospective LCA is a tool that not only puts claims of environmental sustainability to the test but also allows the test of policy measure options, as well as supports technology improvement and investment decisions.
The study aims to explore potential SAF technology pathways and quantify, from the perspective of the fuel user, the potential environmental impacts that can be attributed to different SAF by utilizing a STS approach for pathways selection and prospective LCA for environmental impact assessment. The study compares the potential life cycle environmental impacts of the 30% SAF (mandate imposed by the Swedish government) and 100% SAF, produced from different pathways, to jet fuel in the year 2030, as well as identifies hotspots in SAF life cycles. The implications of the study could serve as a source of information to support future policy and investment decision-making.
Methods and Materials
Prospective LCA is an assessment method that studies emerging technologies and analyzes their potential future life cycle environmental impacts (Cucurachi et al., 2018). Arvidsson et al. (2018) explained that the primary features that differentiate a prospective LCA from its retrospective counterpart are the inclusion of future time consideration in the choice of emerging technology pathway, the projection of the background systems and upscaling of the foreground systems. Background systems are the parts of a product system that cannot be directly influenced by the developer of new technology, while foreground systems are the emerging technologies that can be directly manipulated by the technology developer (Arvidsson et al., 2018). They explained that background systems should be relevant to the time horizon of the study such that temporal inconsistency between foreground and background could be avoided. Cucurachi et al. (2018), in addition, indicated that a comparative LCA conducted for future times would only be fair if an up-scaled emerging technology is putting up against its evolved incumbent counterpart. Therefore, technology up-scaling in the foreground system is crucial to the performance of prospective LCA.
The remainder of this section is as follows. Highlighting the future time aspect of a prospective LCA, Section Selecting SAF Technology Pathways develops a four-step method to select the SAF technology pathways. Then, we clarify methods adopted for background system projection and upscaling of foreground systems in Sections Projection of Background Systems and Upscaling of Foreground Systems. Lastly, Sections Scope of LCA Model and Data Inventory explain the scope of the LCA model and how data inventory is accomplished.
Selecting SAF Technology Pathways
Selection of SAF technology pathway involves choice making. Choices on feedstock types, technologies for feedstock production, feedstock conversion and fuel synthesis are in turn demarcated by constraints. Here, we propose four steps (Figure 1) to select SAF technology pathways.
Our assumption is that feedstock types and availability (Step 1 in Figure 1) are dependent on the market environment, institutions and cross-sectorial development. Hence, we borrow some ideas from sustainability transitions literature (Markard et al., 2012) and conceptualize feedstock supply as a sub-segment in the STS of the aviation sector. Inspired by a flat ontological assumption, we consider technologies, actors, and institutions as mutually constitutive agents, flatting the distinction between human and non-human agents (Garud and Gehman, 2012). We also assume that a technological shift in one industrial sector affects the development in other sectors, inducing co-evolution and inter-dependence between industrial sectors (Bergek et al., 2015). Figure 2 illustrates our application of STS approach with a flat ontology, in which climate policy is seen as the key agent for novel technological development in different sectors. Assuming feedstock A has been identified as the primary resource to support the development of sector X, to assess A's availability, we study the interactions between A and the existing markets (Figure 2A). Next, we analyze the implications of existing and upcoming relevant national and regional policies on the availability of A (Figure 2B). Finally, we consider the potential impacts that other climate policy-driven sectors or markets have on A's availability (Figure 2C). The assessment would lead to a conclusion that either supply of A is sufficient to satisfy demand or an alternative (feedstock B) is required to supplement feedstock A (Figure 2D). The assessment process iterates for each feedstock alternative identified. As demonstrated in Section System Boundary, the STS approach helps identify feedstock types that could potentially contribute to SAF production in Sweden.
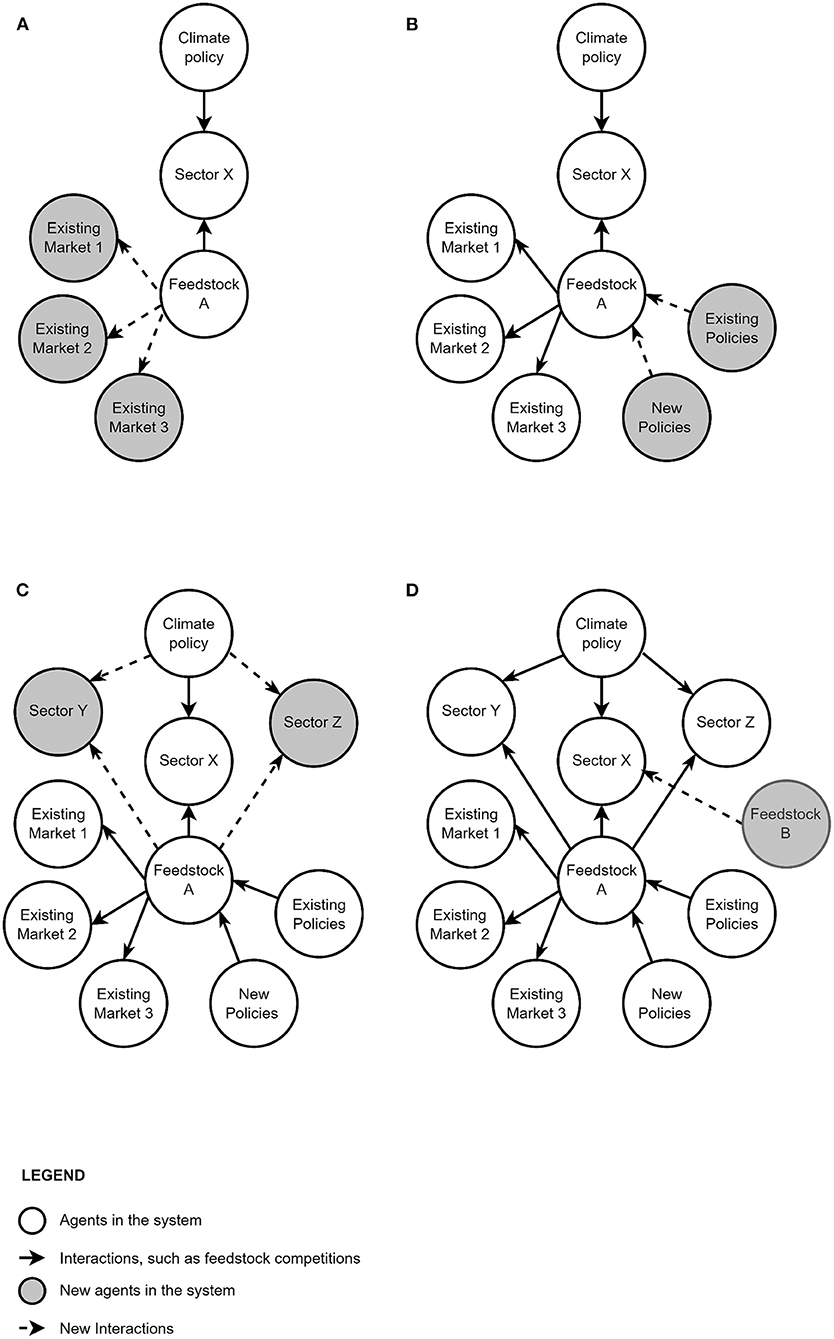
Figure 2. An application of a STS approach to assess feedstock types and availability. (A) Interactions between feedstock and existing markets; (B) Interactions between feedstock and policies; (C) Interactions between feedstock and other emerging sectors; (D) Inclusion of new feedstock alternative.
To select the feedstock production technology in Step 2 (Figure 1), feedstock types, technological limitations, TRL, cost and experiences from other sectors are taken into account. Steps 3 and 4 (Figure 1) are interdependent. The feedstock is converted to an intermediate product to feed the fuel synthesis processes, but it is the choice of fuel synthesis processes in Step 4 that dictates the feedstock conversion process. Thus, feedstock conversion technology choice (Step 3) is reliant on the fuel synthesis process, technological limitation, TRL and cost whereas fuel synthesis process determination (Step 4) is limited by the approved fuel production standard (ASTM, 2021), feedstock compatibility and cost. Implementation of steps 1–4 is demonstrated in Section System Boundary.
Projection of Background Systems
Steubing and de Koning (2021) advised that the background systems chosen for a specific future time horizon should reflect the wider technological and economic development at that particular point in time, including energy generation, material supply, manufacturing techniques and waste management etc. Mendoza Beltran et al. (2020) and Steubing and de Koning (2021) proposed that such future background can be represented by merging overarching future scenarios derived from the integrated assessment model (IAM) (Moss et al., 2010) with the Ecoinvent database (Wernet et al., 2016). To capture the wider aspect of local, regional and global social development in future times, we adopt a background system developed by Steubing and de Koning (2021). Their background system consists of a database made up of the Ecoinvent database and data that are common to all IAM scenarios, and a scenario difference file that portrays the specific IAM scenarios at targeted reference years. The integration of such future-oriented database and scenario difference files is shown in Figure 3. Detailed background systems used by the study are shown in Section Background Systems.
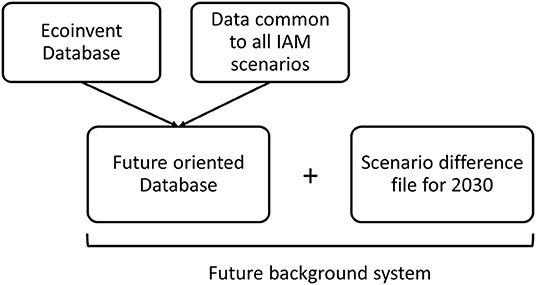
Figure 3. A simplified representation of a future background system for the year 2030. Adapted from Steubing and de Koning (2021).
Upscaling of Foreground Systems
Upscaling of emerging technologies with varying maturity levels would require a tap into the plausible future scenarios of their industrial-scale implementation (Cucurachi et al., 2018). To scale up the technology, Tsoy et al. (2020) proposed utilizing technological experts to hypothesize the projected future technology scenarios. Once defined, the scenarios could be translated into LCA flow charts by LCA experts and thereafter the unit process data including energy, material and elementary flows be estimated to the desired scales (Tsoy et al., 2020). There are several ways to go about data estimation, for example, Cossutta et al. (2017) and Mazzoni et al. (2019) employed process simulations to estimate large-scale data flows of their emerging technologies. While Piccinno et al. (2018) proposed the use of manual calculations to scale up their laboratory-based process, Villares et al. (2016) and Schulze et al. (2018) utilized the proxy method for the same purpose. Based on their literature review, Tsoy et al. (2020) found that the accuracy of data estimation methods decreases from process simulation to manual calculations, to proxy data and data omission. In this study, future technology scenarios are projected through reviewing literature and institutional reports that focus on technology optimization and the future development of the targeted technology. Data estimation is conducted primarily through collecting secondary data from the relevant process simulations. In cases of lack of simulation data, manual calculation and proxy data estimation are utilized. This is done following Tsoy et al.'s (2020) upscaling framework, to minimize potential data uncertainty as far as possible. Details of technology upscaling for the current study are presented in Section Foreground Systems.
Scope of LCA Model
Functional Unit
The LCA is designed to compare fuels that could be used in the same aircraft type, i.e., with the same combustion technology, thereby circumventing the potential complications introduced by differences in technological efficiencies (Elgowainy et al., 2012). For this reason, adopting energy in fuel as the reference value of the functional unit can be considered reliable (Elgowainy et al., 2012). Therefore, the functional unit chosen is 1 MJ of fuel consumed by a twin-engine single aisled aircraft in a typical short-haul flight consisting of takeoff, climb, cruise, descend, approach and landing phases. Single aisled aircraft is used as the reference as it is the most popular aircraft type deployed globally (O'Hare, 2022).
System Boundary
The life cycle of aviation fuel covered by this study consists of two stages, namely well-to-pump and pump-to-wake. Well-to-pump stage constitutes feedstock extraction and transportation, feedstock treatment, fuel synthesis and the subsequent transportation to the fuel pump. The combustion of fuel during aircraft operation is termed the pump-to-wake life cycle stage (Elgowainy et al., 2012).
The system boundary of the well-to-wake life cycle of aviation fuels in 2030 is presented in Figure 4, which summarizes the results of applying the four-step technology pathway selection process outlined in Section Selecting SAF Technology Pathways. Hydrogen and carbon dioxide (CO2) storage are not included as the syngas production system assumed just-in-time supply. Fuel storage and fuel blending are also excluded as these activities are assumed to have negligible contributions to the life cycles. Black liquor and forest residue feedstock in the study are treated as waste material, in agreement with Annex V of EU (2018), that could be collected from their respective sources with no burden. The selection of the SAF technology pathways is explained in the four steps that follow.
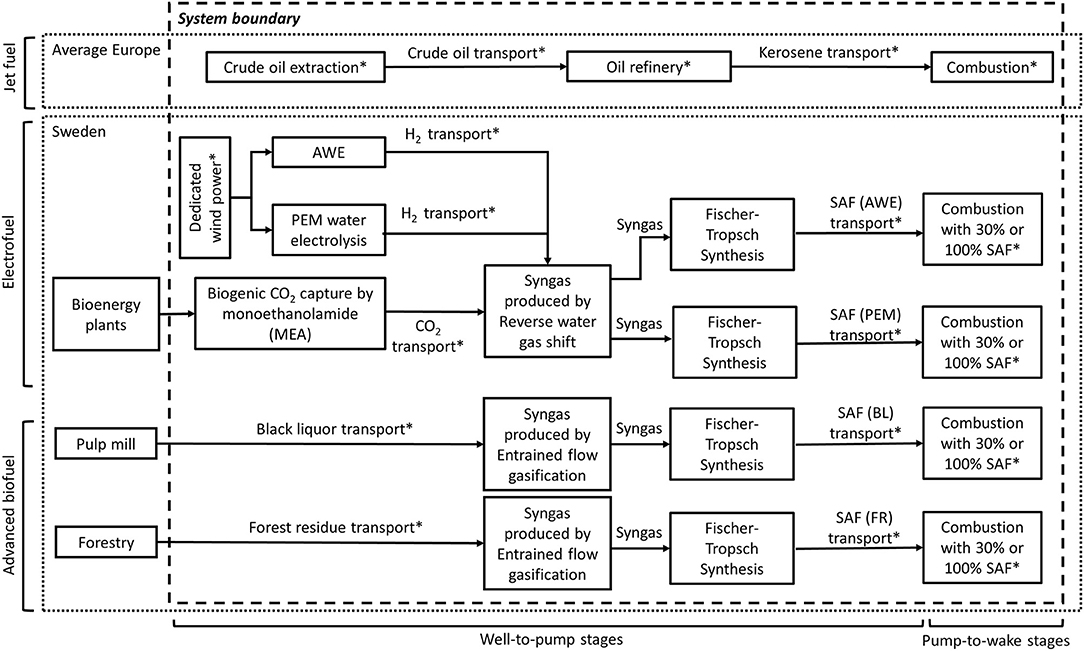
Figure 4. Possible technology pathways for SAF and jet fuel in a scenario for Sweden in 2030. Except for processes marked with * and energy inputs that are not shown in the figure, all processes within the system boundary belong to the foreground system. AWE, alkaline water electrolysis; PEM, polymer electrolyte membrane; BL, black liquor; FR, forest residue.
SAF Technology Pathway Selection Step 1: Feedstock Types and Availability
Local SAF production is seen supported by some political parties (Kulanovic and Nordensvärd, 2021) and some actors in the aviation sector (Christley et al., in progress) in Sweden. It has been suggested by researchers that rich resources like forest residue, biogenic CO2 and renewable electricity could drive the Swedish SAF (lignocellulosic-based advanced biofuel and electrofuel) production (Hansson et al., 2017; Trinh et al., 2021). We adopt a STS approach, as proposed in Section Selecting SAF Technology Pathways, to analyze the availability of each of these potential feedstock options qualitatively, based on their interactions with the market environment, policies and other industrial sectors to understand their potential in contributing to the demand in 2030. Such analysis is elaborated in Figures 5A–D and the paragraphs that follow. The results show that lignocellulosic-based feedstock (forest residue and black liquor) and electrofuel-based feedstock (renewable electricity and biogenic CO2) may all play a part in contributing to the future Swedish SAF production.
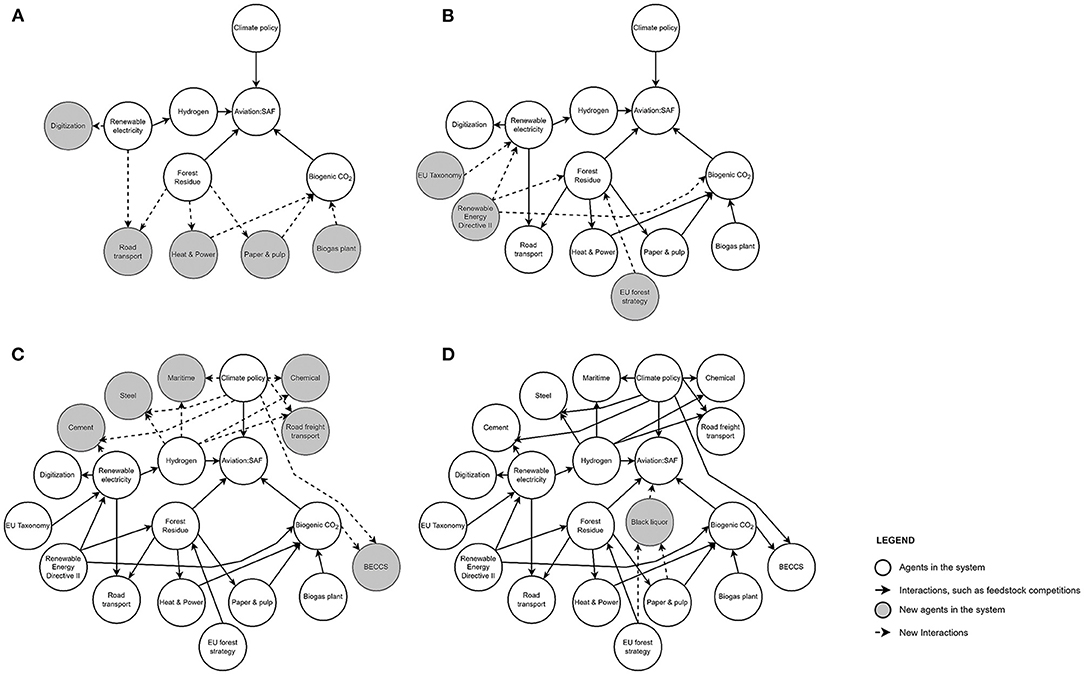
Figure 5. Application of STS approach to assess forest residue, biogenic CO2 and renewable electricity as SAF feedstock types and their availability in Sweden. (A) Interactions between feedstock and existing markets/sectors; (B) Interactions between feedstock and policies; (C) Interactions between feedstock and other emerging markets/sectors; (D) Inclusion of new feedstock alternative.
In the shadow of bioeconomy development, the extensive use of forest residue in future SAF production is debatable. For instance, Börjesson et al.'s (2017) estimation of additional forest logging residue to support the growth of paper and pulp, power and heat and road transport sectors in 2030 is in direct feedstock competition with SAF production. The limitation of forest residue supply makes black liquor, a by-product of pulp mill (IEA, 2007) that is qualified by the EU (2018) as an advanced biofuel feedstock, an interesting alternative lignocellulosic feedstock for SAF production (IEA, 2007; Jafri et al., 2020). Besides, the appreciable amount of chemical pulp mills in Sweden estimated by Pettersson et al. (2015) might ensure an uninterrupted supply of black liquor. However, taking the EU forest strategy that proliferates the function of the forest as a carbon sink (EU, 2021b) into account, the supply of any lignocellulosic material could be restricted and unlikely to meet the demand in 2030 on its own.
Non-fossil CO2 can be one of the ingredients for electrofuel production in Europe (EU, 2018). In Sweden, the biogenic CO2 feedstock could be recovered from bioenergy point sources such as paper and pulp mills or biogas plants etc. (Hansson et al., 2017). In their study of biogenic CO2 point sources in Sweden, Hansson et al. (2017) projected that the sum of all recoverable biogenic CO2 could produce sufficient electrofuel to cover the entire Swedish transportation demand. However, the deployment of bioenergy carbon capture and storage (BECCS) may limit CO2 supply. The potential development of BECCS credits, an acceptable avenue to compensate for hard-to-abate emissions in the EU (Zetterberg et al., 2021), could constrain the supply of CO2 in the future. In other words, potential biogenic CO2 competition should be taken into account when electrofuel production is concerned.
Green hydrogen, another ingredient for electrofuel production, is produced from renewable electricity (EU, 2018). Although the EU Taxonomy Complementary Delegated Act has classified certain nuclear activities as sustainable (EU, 2022), whether the projected 2030 Swedish electricity production grid, which composes of ~30% of nuclear power (Energimyndigheten, 2021a), is qualified for green hydrogen production is still debatable. It is worth mentioning that the additional electricity demand from hydrogen production is not currently included in the grid expansion planned for 2030, which is mainly targeting to support road electrification and societal digitization (Energimyndigheten, 2021a). Moreover, renewable electricity competition from actors undergoing fossil-free transitions including steel (Pei et al., 2020), heavy road transport, maritime (Energimyndigheten, 2021b), cement (Energimyndigheten, 2021a) and chemical (Fossil Free Sweden, 2021) are also anticipated. To elude grid power competitions and at the same time ensure 100% renewable electricity delivery, a dedicated renewable electricity supply might be a viable option for hydrogen production in Sweden.
SAF Technology Pathway Selection Step 2: Feedstock Production Technology
The dependency of feedstock production technology on feedstock types, technological limitations, TRL, cost and development of other sectors are explained in this section.
The proposed Swedish national hydrogen strategy (Energimyndigheten, 2021b) has identified alkaline water electrolysis (AWE) (TRL 8), polymer electrolyte membrane (PEM) (TRL 7) and solid oxide electrolyzer cell (SOEC) (TRL 5) as the relevant hydrogen production technologies. Given that AWE and PEM have similar merits in technical performance and cost (see Table 1), they are both included in the study. In contrast, SOEC is rejected mainly due to its technological limitations.
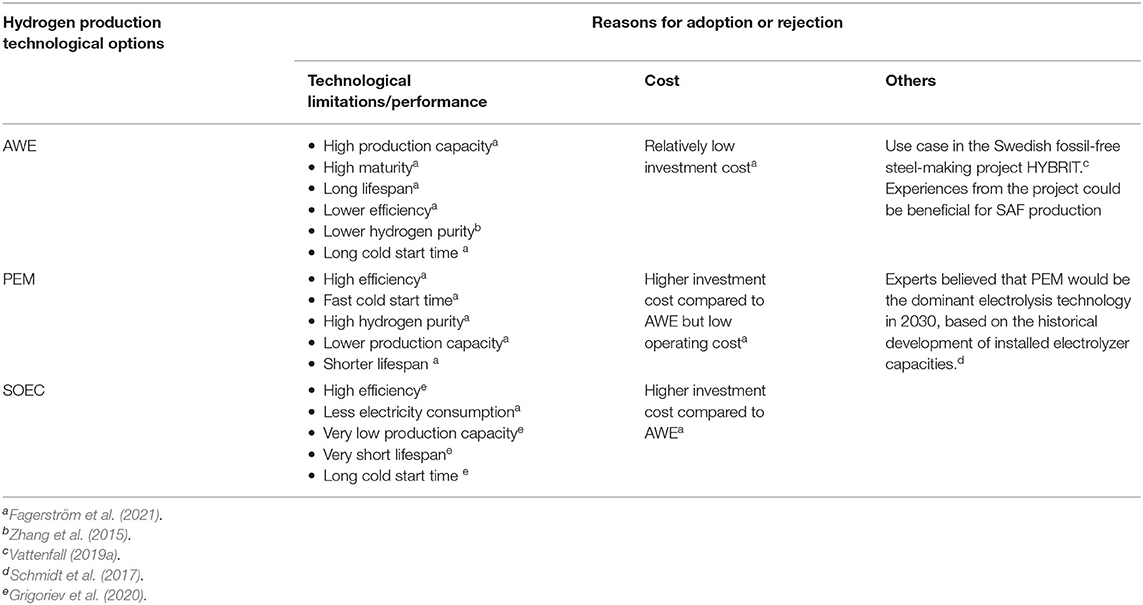
Table 1. Technological limitations/performance, cost, and development of other sectors that affect the selection of hydrogen production technology.
Non-fossil CO2 can be captured from the air or bioenergy point sources. Direct air capture (DAC), despite its site independency, is rejected as a technological choice mainly due to its low concentration yields. Monoethanolamide (MEA) or hot potassium carbonate (HPC), with TRL of 9 and 7, respectively, are relevant representatives of point source carbon capture technologies (Fagerström et al., 2021). MEA is however chosen for the study, given that HPC's cost-effectiveness is tied to specific applications, which poses a limitation to its use (see Table 2 for details).
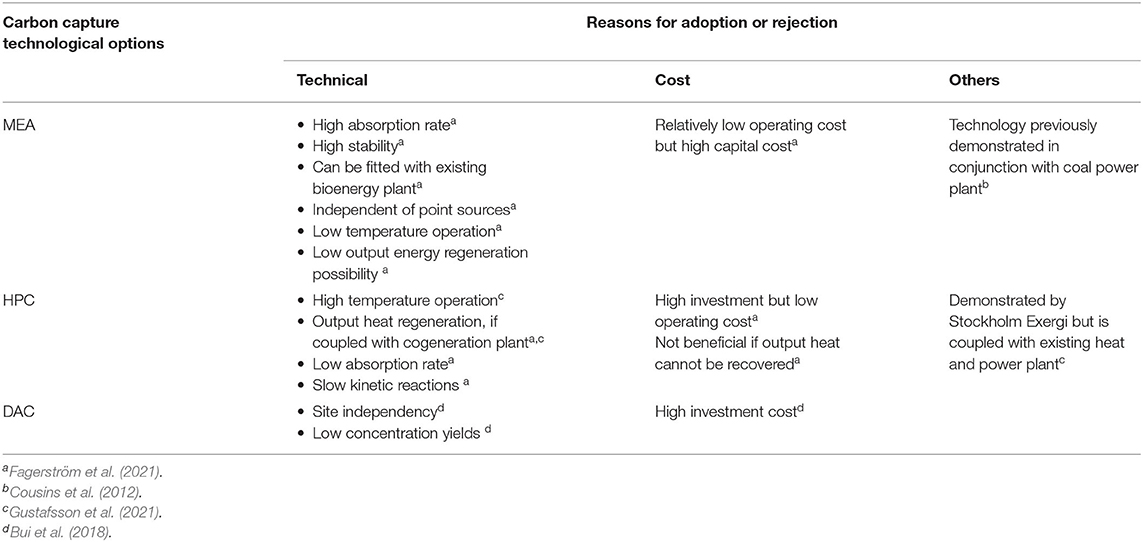
Table 2. Technological limitations/performance, cost, and development of other sectors that affect the selection of carbon capture technology.
SAF Technology Pathway Selection Step 3: Feedstock Conversion Technology
Fischer-Tropsch (FT) synthesis, as mapped in Step 4 (Section SAF Technology Pathway Selection Step 4: Fuel Synthesis Process), is a catalyzed chemical reaction used to convert syngas, a unique mixture of carbon monoxide and hydrogen (Foit et al., 2017), to various liquid hydrocarbons (Ail and Dasappa, 2016). To produce syngas, hydrogen and CO2 would be subjected to a reverse water gas shift (rWGS) process (Schmidt et al., 2018), whereas lignocellulosic feedstock would need to go through gasification (Ail and Dasappa, 2016; Jafri et al., 2016).
rWGS, with low technical maturity (EU, 2021a), is the sole technology available to convert CO2 to syngas, which is also the reason for incorporating the technology into the system boundary.
Black liquor can only be gasified efficiently with entrained flow gasification technology (Jafri et al., 2016). A pilot demonstration of the technology in northern Sweden earlier (Jafri et al., 2016) is a valuable reference for SAF production. Forest residue gasification, on the other hand, is technically mature and can be done at high efficiency either by fluidized bed or entrained flow gasification technology (Molino et al., 2018). Given that syngas yielded from entrained flow gasification contains less char and tar (Molino et al., 2018), and the use of technology common to black liquor gasification might be economically beneficial, entrained flow gasification of forest residue is selected.
SAF Technology Pathway Selection Step 4: Fuel Synthesis Process
Mapping feedstock with the approved aviation fuel production standard stipulated by the ASTM D7566 (ASTM, 2021) results in Fischer-Tropsch (FT) and alcohol-to-jet (AtJ) being identified as the eligible processes. Whist lignocellulosic-based SAF or advanced biofuel can be synthesized with FT and AtJ, electrofuel is only approved to be produced from FT for the time being (EU, 2021a). From the point of commonality, hence cost-saving perspective, FT is chosen as the fuel synthesis process in the study.
Assumptions, Cut-Off, and Allocation
The study adopted a cut-off allocation approach, by which the burden of treating waste material is borne by the primary user of the material, while recycled materials are available burden-free (Ekvall et al., 2020). In our case, forest residue is assumed as waste material with no burden. By the same token, biogenic CO2 is considered a burden-free carbon source, where the flue gas is assumed treated by the bioenergy plants before release, and black liquor is assumed as waste material rather than a co-product. Therefore, bioenergy plants, pulp mills and forestry are considered external to the system boundary (Figure 4). This cut-off approach is valid as long as the waste flow remains as waste and does not assume any economic values. While this approach is consistent with the Ecoinvent cutoff database (Ecoinvent, 2022), which is utilized by the study, as well as how the EU (2018) quantifies the emissions of waste material, it does not consider the avoided burdens or functions of those waste streams in the case when kerosene is used instead of SAF. This choice deviates from the ISO 14044 recommendations where system expansion is prioritized over allocation (ISO 14044, 2006). On the other hand, it is in line with the aim of the study which is to quantify, from the perspective of the fuel user, what impacts can be attributed to different SAF.
Non-waste by-products of energy processes are allocated with burden based on their energy content. Allocation applies to entrained flow gasification of black liquor and FT fuel synthesis in this study. Details of these processes are presented in Supplementary Material. It is worth mentioning that there are other ways such as mass, economic values, exergy and system expansion to allocate burden. Mass allocation is not used for the reason that mass cannot be allocated to the electricity by-product yielded from the FT fuel synthesis process. Similarly, economic allocation is not employed given that the economic values of green liquor (a by-product of black liquor gasification) and the future prices of electricity are largely unknown. Exergy, owing to a lack of data associated with green liquor, is also not used for allocation. Finally, system expansion is not considered because it is uncertain which system(s) the green liquor will be substituting in future times.
Geographically, feedstock conversion and fuel synthesis processes are assumed to be located close to the existing oil refineries on the Swedish west coast, typically Gothenburg and Lysekil (Figure 6), taking advantage of the know-how from the industries. According to the prediction of Energimyndigheten (2021b) and Stenkvist (2021), northern Sweden will be the most likely location for future wind power and major hydrogen-dependent projects. Therefore, it might be reasonable to assume a centralized supply of green hydrogen from the same region in the future. Biogenic CO2 could be captured from high concentration point sources distributed in the SE3 region as described by Hansson et al. (2017). Forest residue is presumably transported from sustainably managed and productive forests in southern Sweden (SE4 region) while black liquor could be extracted from pulp mills located in central and southern Sweden (Pettersson et al., 2015).
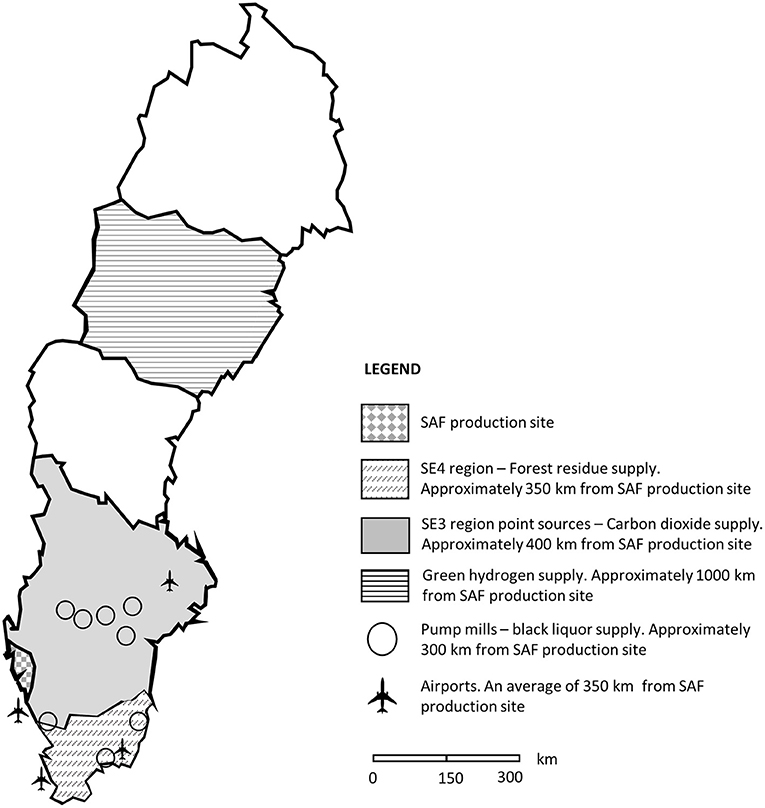
Figure 6. Map of Sweden with locations of potential SAF production sites, potential feedstock supply sites and location of average Swedish airports.
Impact Categories
The current study assesses the potential climate impact, GWP100 and GWP20, of the future Swedish-produced SAF and conventional jet fuel. Global warming potential in the time horizon of 100 years (GWP100) is chosen as the assessment metric, for the reason that it is the most common metric adopted in climate policy application and LCA assessment (Tanaka et al., 2010). The 20 years time horizon is selected to investigate the reportedly elevated impact of short-lived aviation non-CO2 emissions in the use phase of the life cycle (Lee et al., 2021). To assess the potential well-to-wake (excluding non-CO2 emissions) climate impact at different time scales, ReCiPe 2016 Midpoint (I) and (H) methods (Huijbregts et al., 2016) are used to evaluate 20 and 100 years time horizons, respectively. Concerning global warming potential induced by the non-CO2 emissions from aviation, including NOX, water vapor, soot and sulfate, Lee et al. (2021) investigated the exogenous relationships between aviation emissions and earth-atmosphere radiation budget. As such, non-CO2 contributed potential climate impact in the use phase of the life cycle is quantified with the characterization factor proposed by Lee et al. (2021). The potential impact attributed to aviation-induced contrail cirrus is not included in the study due to the complexity and uncertainty involved in the formations of these species (Lee et al., 2021).
As emphasized by Algunaibet and Guillén-Gosálbez (2019), focusing solely on climate change when performing environmental studies of energy systems may undermine other impact categories, which could lead to suboptimal decision making, resulting in potential burden shifting. Previous studies conducted by Kleijn et al. (2011) and de Koning et al. (2018) found that low carbon technology is oftentimes metal intensive, while Yellishetty et al. (2012) stated that human toxicity and ecotoxicity are impact categories relevant to the minerals and metals sector. The construction of dedicated wind power plants and the additional demand for electricity to support the SAF production (Figures 4, 5) could entail urban land use and higher capacity of the Swedish electricity grid. As such, to ensure that potential burden shifting is captured by the study, mineral depletion potential (MDP), freshwater ecotoxicity, human toxicity, land use and ionizing radiation in the well-to-pump life stages are also assessed, using the mineral depletion, freshwater ecotoxicity, human toxicity, ionizing radiation, agricultural and urban land occupation metrics calculated with ReCiPe Midpoint (H) method (Huijbregts et al., 2016). Further justification of the choice of impact categories can be found in Supplementary Figure S9.
LCA Modeling
The Activity Browser, an open-source LCA software (Steubing et al., 2020), is used to model and analyze the results of the well-to-pump life stages of jet fuel and the various types of SAF. The potential climate impacts of the pump-to-wake stage of the life cycles, on the other hand, are calculated manually.
Data Inventory
Background Systems
The background database and the scenario difference file adopted in this study are generated from Brightway, an open-source LCA framework (Mutel, 2017; Steubing and de Koning, 2021). The database is a combination of Ecoinvent version 3.7.1 and common IAM data, while the scenario difference file portrays the IAM Shared Socio-economic pathway “Middle of the Road” below 2°C scenario (O'Neill et al., 2014) at 2030.
The background database is designed to represent the generic regional development (Steubing and de Koning, 2021), in which sectorial progress in any specific country is not reflected. To portray energy development in Sweden, we add Swedish electricity production mix data to supply the foreground systems. To project the Swedish electricity production mix in the year 2030, the scenario “Electrification” proposed by Energimyndigheten (2021a) is adopted since the high degree of sectoral and societal electrification portrayed by this scenario agrees with the future anticipated by the energy sector (NEPP, 2020). The assumptions made by the “Electrification” scenario and the dataset used to construct the 2030 electricity production mix are explained in the Supplementary Material. Additionally, to align with the cut-off allocation approach described in Section Assumptions, Cut-Off, and Allocation, where forest residue is available burden-free, the burden allocated to wood chips in the dataset of electricity generated by the combined heat and power plants (CHP) is removed.
As indicated in Figure 4, jet fuel is a part of the background systems. Dataset for kerosene production in average Europe is used to represent the conventional jet fuel in the study. Besides kerosene production, the dataset includes the necessary transport of kerosene from the production plant to an average European location, which is assumed to sufficiently cover the distance to travel to any average Swedish airport. It is also noted that infrastructure and the associated land use are accounted for by the dataset; in other words, the foreground systems should reflect the same scope as kerosene, to ensure the final comparison with jet fuel is fair.
To represent local transportation, a freight train driven by electricity is assumed as the default transport mode for any distances >300 km traveled in Sweden (Schenker, 2020). Distances <300 km and transportation of SAF are covered by EURO 6 class lorry. Cryogenic tanks are not used for the transport of hydrogen or carbon dioxide, instead, regular freight trains or Euro 6 lorry are assumed.
Data for the pump-to-wake life cycles including aircraft type, the average distance traveled, passenger capacity, fuel consumptions, emission types and quantities at different phases of flight are derived from the dataset for various aircraft averages published by the U.S. Volpe National Transportation Systems Center (Elgowainy et al., 2012).
Foreground Systems
Technologies included in the SAF technology pathways (Figure 4) make up the foreground systems. Both AWE and PEM are hydrogen production technologies that use electric energy to split water. While AWE utilizes liquid alkaline electrolytes (Koj et al., 2017), PEM employs a thin solid polymer electrolyte membrane as an electrolyte (Bareiß et al., 2019). MEA is a post-combustion carbon capture technology that employs amine solvent to absorb carbon dioxide in the flue gas stream exited from CO2 point sources (Iribarren et al., 2013; Kuparinen et al., 2019). While rWGS reaction produces syngas by treating CO2 with hydrogen (González-Castaño et al., 2021), entrained flow gasification converts lignocellulosic material, including black liquor and forest residue, into syngas (Jafri et al., 2016; Molino et al., 2018). FT synthesis is a catalyzed chemical reaction in which synthesis gas is converted into liquid hydrocarbons of various forms via the use of a reactor with cobalt or iron catalyst (Ail and Dasappa, 2016).
Future technology scenarios are formulated based on data obtained from literature and institutional reports review, focusing on optimization and future development of technology. Performed per Tsoy et al.'s (2020) upscaling framework, data estimation of MEA carbon capture, rWGS syngas forming, gasification of black liquor and FT fuel synthesis are conducted through process simulation data collection. In the case of AWE and PEM hydrogen production, where process simulation data are unavailable, manual calculations are employed. Data for forest residue gasification is estimated based on proxy, given that a relevant simulated process is identified. Descriptions of projected scenarios, detailed LCA flow charts, data estimation and dataset used in LCA modeling for all the technologies included in the four SAF technology pathways (Figure 4) are presented in Supplementary Material.
Results
Results of the prospective LCA of the 30% SAF, 100% SAF and jet fuel GWP, ionizing radiation, MDP, land use and toxicity impacts are presented in Sections Potential Well-to-Wake Climate Impact and Well-to-Pump Life Cycles. Sensitivity analysis of uncertain modeling choices is performed and presented in Section Sensitivity Analysis.
Potential Well-to-Wake Climate Impact
From the perspective of the entire life cycle, the performances of 30% SAF fuels in the GWP20 (between 260 and 263 g CO2−eq/MJ) and GWP100 (between 94 and 97 g CO2−eq/MJ) categories are not drastically different from that of jet fuel (290 g CO2−eq/MJ in 20 years and 120 g CO2−eq/MJ in 100 years), where a majority of the impacts are contributed by fuel combustion (Figure 7). This can be explained partly by the burden from the 70% volume of jet fuel, but also by the contribution of non-CO2 emissions. In the case when non-CO2 impacts are excluded, the GWP20 and GWP100 of the 30% SAF fuels are calculated to be between 59 and 62 g CO2−eq/MJ, and between 58 and 61 g CO2−eq/MJ, respectively. The corresponding values for jet fuel are 85 g CO2−eq/MJ in 20 years and 84 g CO2−eq/MJ in 100 years. The non-CO2 impacts, as seen in Figure 7, are comparable regardless of fuel types, indicating that the use of SAF is not free from non-CO2 emissions. These impacts are particularly pronounced in the 20 years horizon (Figure 7), due to the net photochemical effects of aviation NOX, including increase and reduction of ozone, methane and stratospheric water vapor, which is ~5.4 times higher than a 100 years consideration (Lee et al., 2021). Otherwise, all of the 100% SAF types are having minimal impacts in the combustion stage (excluding non-CO2) when only CO2, methane and nitrous oxide emissions are included, for the reason that biogenic CO2 emissions are discounted by the ReCiPe 2016 Midpoint (H) and (I) calculation methods.
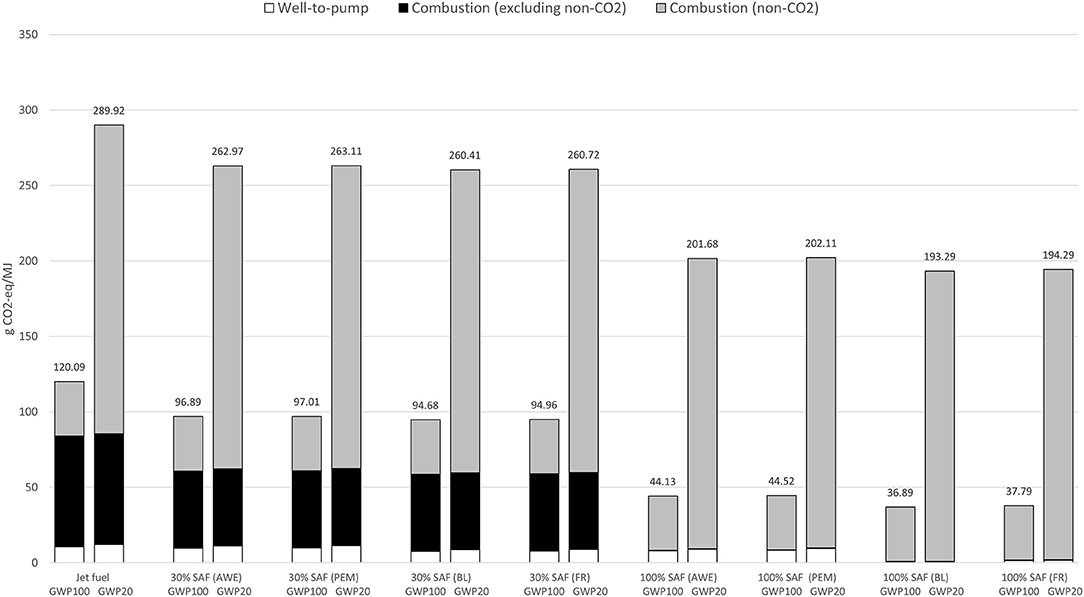
Figure 7. Well-to-wake potential climate impacts (100 years horizon and 20 years horizon) per 1MJ of jet fuel, 30% SAF and 100% SAF in 2030.
Even though the well-to-pump phases are not the dominating contributors to potential climate impact, they are not negligible. Specifically, the contributions from the production of electrofuel, SAF (AWE) and SAF (PEM) in Figure 7, are comparable to jet fuel production. In contrast, the GWP20 and GWP100 of producing SAF from black liquor (BL) and forest residue (FR) are notably lower. Well-to-pump contributions to climate impact are further explained in Section Well-to-Pump Life Cycles.
Well-to-Pump Life Cycles
As illustrated in Figure 8, the average GWP100 of electrofuel production, SAF (AWE) or SAF (PEM) is not significantly lower than that of jet fuel production. Analysis of 100% SAF (AWE) and 100% SAF (PEM) shows that both cases could attribute over 68% of their GWP to hydrogen production, of which more than 90% is from electricity generation from dedicated wind power, owing to the production of steel and concrete (Supplementary Figures S-10, S-11). About 22% of the GWP is contributed by the biogenic CO2 capture process, where the use of electricity from the Swedish production mix is identified as the main contributor. Although a majority of the Swedish electricity in 2030 would be generated from renewable sources, they are not completely emission-free when the share of wind power and hydropower original construction, as well as feedstock to biogas plants and blast furnaces for iron purification (with electricity as a by-product), are taken into account. Overall, the intensive electricity demand for hydrogen production is the main driver of the GWP of electrofuel production.
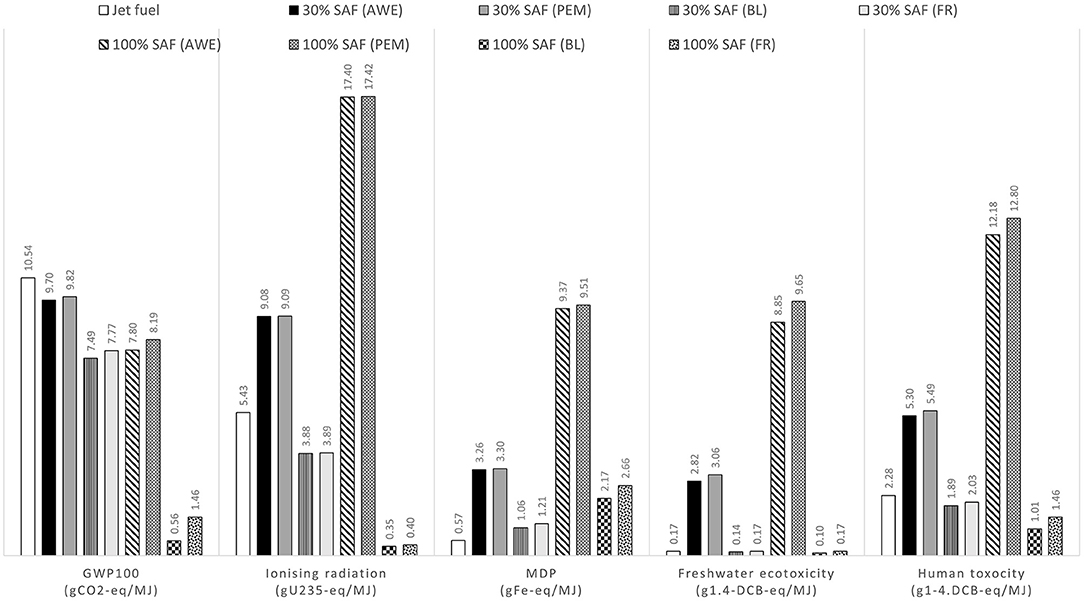
Figure 8. Well-to-pump potential climate impacts (100 years horizon), ionizing radiation, potential mineral depletion, freshwater ecotoxicity and human toxicity per 1MJ of jet fuel, 30% SAF and 100% SAF in 2030.
In comparison, the GWP of forest residue and black liquor originated 100% SAF is remarkably low (Figure 8), which can be largely explained by the assumption that both materials are considered as waste and therefore available as burden-free, per the cut-off allocation principle. By the same token, one could infer that the global warming potential of captured biogenic CO2 would be higher if the burden from bioenergy production would be partially allocated to the released biogenic CO2.
From Figure 8, it is clear that the larger the volume of SAF produced, the higher would the MDP be, regardless of technology pathways. Cobalt produced for the use as a catalyst in FT synthesis is identified as the common contributor to MDP for all the SAF production types. In addition, copper and steel used by wind turbines for renewable electricity generation contributed on average more than 50% of MDP from SAF (AWE) and SAF (PEM) productions.
The potential impact of ionizing radiation increases with the volume production of electrofuel, with 100% SAF (AWE) or SAF (PEM) on average having a 220% higher impact than that of jet fuel (Figure 8). This relatively higher impact of ionizing radiation could be attributed to the Swedish medium voltage electricity used to operate the biogenic CO2 capturing process, which might consist of 26% of nuclear power in 2030.
The treatment of scrap copper, used in wind turbines and wind turbine network connections, is the main reason for the freshwater ecotoxicity produced by SAF (AWE) or SAF (PEM) compared to jet fuel (Figure 8). The copper smelting process employed in making copper cathode, as a part of wind turbines and network connections, is accountable for the human toxicity associated with SAF (AWE) or SAF (PEM) production (Figure 8). The relative impacts of toxicity increase with the volume of electrofuel production.
Figure 9 shows a potentially higher land use by a larger volume (from 30% to 100%) of SAF (AWE) and SAF (PEM) in comparison to jet fuel. The onshore wind turbine is primarily responsible for the agricultural and urban land use (over 45% contribution on average) in producing electricity to support the production of SAF (AWE) or SAF (PEM).
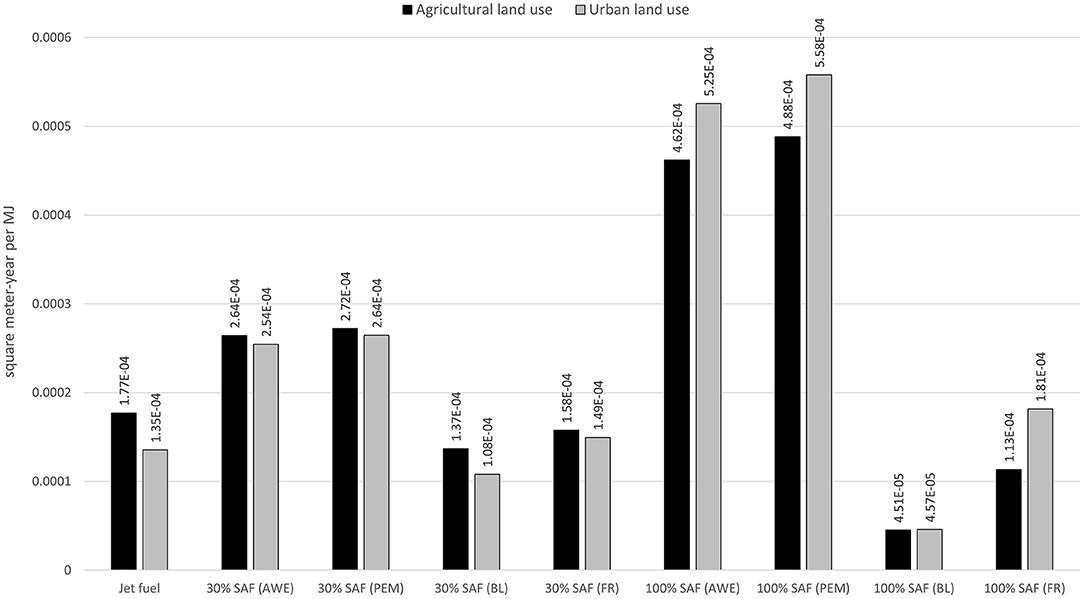
Figure 9. Well-to-pump agricultural and urban land use per 1 MJ of jet fuel, 30% SAF and 100% SAF in 2030.
Sensitivity Analysis
This section analyzes the two main uncertain choices identified based on the results in Sections Potential Well-to-Wake Climate Impact and Well-to-Pump Life Cycles, namely the allocation rule and political definition of renewable energy.
Using cut-off allocation, by which all lignocellulosic-based feedstock considered as waste products are available burden-free, produces SAF with low life cycle environmental impacts (see Figures 7–9). However, as clarified in Section SAF Technology Pathway Selection Step 1: Feedstock Types and Availability and Figure 5, market conditions and the introduction of new policy measures could lead to resource competition, a situation where feedstock like black liquor, forest residue, or biogenic CO2 would no longer be considered as waste and could no longer be taken for granted as burden-free. To understand how burden-borne feedstock, in general, would alter the GWP, MDP, ionizing radiation, land use and toxicity impacts of SAF production, a sensitivity analysis of 100% SAF (FR) and SAF (BL) produced from burden-borne forest residue and black liquor is performed. Assuming forest residue is a commodity, we assign environmental burden to the feedstock for SAF production as well as to the wood chips input to CHP that is removed in the background systems earlier (see Section Background Systems). As a co-product of the pulp mills, we allocate the burden to the black liquor based on its energy content, to be consistent with the rest of the LCA model (see Section Assumptions, Cut-Off, and Allocation). Detailed calculations of the allocation factor and dataset used can be found in Supplementary Material.
Renewable electricity generated from dedicated wind power and its high demand for hydrogen production appears to contribute to relatively high GWP, MDP, urban land use and toxicity impacts when electrofuel production is concerned. If nuclear power is accepted as an energy source for green hydrogen production (see Section SAF Technology Pathway Selection Step 1: Feedstock Types and Availability), the potential environmental impacts of electrofuel production might be different. Hence, a sensitivity analysis is conducted by replacing wind power generated electricity with Swedish electricity production mix in the production of green hydrogen for 100% SAF (PEM).
The results of the sensitivity analysis are presented in Table 3. When the burden is allocated to forest residue and black liquor, their GWP and potential agricultural and urban land use are substantially higher than when they are burden-free, indicating the potential impact of forestry, the source of forest residue and pulp, on climate change and land use in general. The effects of bearing burdens on ionizing radiation, MDP and the toxicity impacts observed in the burden-borne SAF (FR) and SAF (BL) can be explained by the electricity usage in the wood chip and pulp production processes. Compared to jet fuel, the potential land use impacts and freshwater ecotoxicity of the burden-borne SAF (FR) and SAF (BL) could be significantly higher.
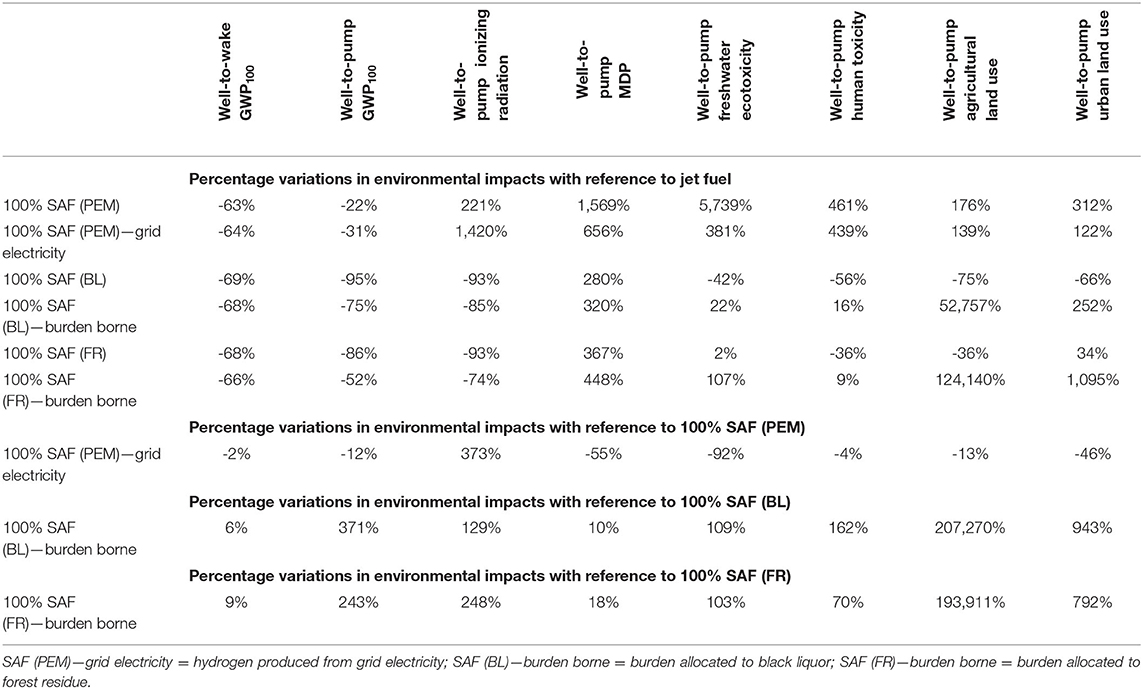
Table 3. Percentage variations in environmental impacts per 1MJ of 100% SAF (PEM)—grid electricity, 100% SAF (BL)—burden borne and 100% SAF (FR)—burden borne with references to jet fuel, SAF (PEM), SAF (BL) and SAF (FR).
Replacing dedicated wind power generated electricity with grid electricity results in an ~12% well-to-pump GWP, 54% MDP, 92% freshwater ecotoxicity, 4% human toxicity, 13% agricultural land use and 46% urban land use reduction in SAF (PEM) production (Table 3). Compared to a 100% dedicated wind power source, the projected share of wind power in the electricity production mix is significantly smaller, clarifying the reduction seen in the analysis. The GWP, human toxicity and agricultural land use reduction are comparatively lower for the reason that the electricity production grid consists of multiple contributing sources to these impact categories, whereas the larger reduction in MDP, freshwater ecotoxicity and urban land use can be attributed to the production grid's less dependency on wind power. On the other hand, the use of grid electricity is likely to induce higher ionizing radiation (Table 3) given the relatively large presence of nuclear power in the projected Swedish electricity grid. Except for GWP100, the grid electricity-driven SAF (PEM) could potentially have higher impacts in the other environmental categories compared to jet fuel.
The ranking of well-to-wake GWP100 of the different SAF types does not change despite the changes in allocation rules and energy sources (Table 3; Figure 7). From the results of well-to-pump GWP100, it can be seen that even though SAF (PEM) is still the worst performer among the SAFs, the potential climate impact of burden-borne SAF (FR) and SAF (BL) is not as insignificant as their burden-free counterparts.
Discussions
Comparing GWP With Earlier Studies
Earlier studies reported by Kolosz et al. (2020), Larson et al. (2020), Obnamia et al. (2020), Pamula et al. (2021), and Prussi et al. (2021), etc. compared life cycle GWP100 of different SAF to that of conventional jet fuel. Based on the feedstock types and modeling choices, we find that Prussi et al.'s (2021) and Fagerström et al.'s (2021) studies bear some resemblance to ours. Prussi et al.'s (2021) assessment of the well-to-wake life cycle (excluding non-CO2 impacts) of forest residue-based SAF resulted in a GWP100 of 8.3 g CO2−eq/MJ, in contrast to 38 g CO2−eq/MJ yielded from our study (Figure 7). If non-CO2 impacts are discounted from our evaluation, well-to-wake GWP100 of SAF (FR) is calculated as 1.9 g CO2−eq/MJ. This value is lower because first, our study focuses on site-specific SAF whereas Prussi et al.'s (2021) represent the global average where transport contributes to a large part of the GWP. Secondly, our LCA is prospective and projects technology to the future commercial scale to avoid overestimation of potential environmental impacts in 2030, whereas Prussi et al.'s (2021) study was retrospective. Fagerström et al. (2021) studied large-scale electrofuel production in Sweden and reported a well-to-pump GWP100 of Fischer-Tropsch synthesized electrofuel of 19 g CO2−eq/MJ, compared to our average 8 g CO2−eq/MJ [SAF (AWE) or SAF (PEM) in Figure 7]. Despite the large-scale production considerations, Fagerström et al. (2021) did not optimize SAF production or consider future times in the background systems. Given that the values of foreground technology and background systems projection into the future captured by our study are not reflected in other earlier studies, we conclude that a comparison between prospective and retrospective LCA might not yield any meaningful results.
Non-CO2 Emissions
Although the Swedish 30% SAF mandate is termed ambitious, its potential to mitigate climate change, according to Figure 7, is marginal. The inherent benefits of SAF, namely the absence of fossil carbon in the fuel, are only apparent in cases when air operations are powered by 100% SAF, a level that is yet to be approved by the regulator (ASTM, 2021). The non-CO2 emissions, on the other hand, are potentially equally impactful on climate change across the board, signifying aviation non-CO2 effects cannot be eliminated by the sole use of SAF. GWP20 amplifies the significance of the short-lived net photochemical effect of NOX emissions in the near time horizon. Meanwhile, the potential impact of aviation-induced contrail cirrus, which is known to have a strong influence on radiative forcing despite its short lifespan (Lee et al., 2021), is not included in the LCA. In other words, the overall GWP of non-CO2 emissions could amount to even higher values than demonstrated in Figure 7. From the LCA perspective, mitigating non-CO2 emissions from SAF is of utmost importance. Insofar research such as Lührs et al.'s (2021) proposed climate optimized flight planning to avoid contrail formation and Freeman et al.'s (2018) assessment on combustor modification to minimize NOX emissions are all subjected to tradeoffs in the form of fuel penalty. That is to say, a tradeoff analysis between short-lived climate forcers and long-lived greenhouse gas is necessary. Given that the uncertainties associated with the non-CO2 impacts remain high (Lee et al., 2021) and unified methodology to estimate non-CO2 cruise emissions or represent CO2-equivalent emissions metrics are still lacking, intensifying research effort to tackle these obstacles is salient if the benefits of SAF are to be realized.
Climate Change Hotspot in Electrofuel Production
In the well-to-pump life cycle of SAF, steel and concrete used for wind turbine construction are identified as the climate impact hotspots for electrofuel, SAF (AWE) or SAF (PEM), production. Steel and concrete industries in Sweden today are undergoing fossil-free transitions. If incorporating such changes in the LCA background scenario, the GWP of wind power produced electricity is likely to be lower. However, fossil-free steel and low carbon concrete production would only come into operation between 2025 and 2040 (Vattenfall, 2019b; SSAB, 2022), making them non-applicable to the 2030 target given that historically, the new onshore wind farm would take up to 10 years from approval application to final commission (Vattenfall, 2022). Alternatively, utilizing electricity from the Swedish production mix could result in a lower GWP in electrofuel production due to a lower share of wind power in the mix (see Section Sensitivity Analysis). On the other hand, the necessary expansion of the Swedish grid to cover the additional electricity demand in 2030 (see Section SAF Technology Pathway Selection Step 1: Feedstock Types and Availability and Figure 5) is not only a lengthy process (Energimyndigheten, 2021b) but would also entail an increase in wind turbine construction to make up for the production volume (NEPP, 2020). In short, lowering the potential climate impact of electrofuel production is highly dependent on renewable electricity availability, which in turn relies on the timely development of the underlying technologies, such as steel and concrete making. Without speedy and systematic processes of establishing additional renewable electricity capacity and carrying out sustainable transitions in the steel and concrete industries, attaining the desired climate mitigation effect through large-scale electrofuel production by 2030 might be infeasible.
Potential Burden Shifting Effect
Our analysis shows that even though SAF might outperform conventional jet fuel in the climate impact category, it could contribute to a higher environmental impact when other impact categories such as MDP, land use or toxicity are taken into account (Figures 8, 9). The majority of the indicated burden-shifting effects are attributable to the use of electricity generated by wind power, either as an independent source or as part of the electricity grid. Reducing dependency on wind power might alleviate the potential burden-shifting effects. However, care should be taken when alternative power sources are considered. Without the additional wind power capacity, Sweden might have to balance the future electricity demand (see Figure 5) with grid power or an increasing proportion of EU imports in the electricity consumption mix (NEPP, 2020). While the former could mean a higher impact from ionizing radiation (see Section Sensitivity Analysis and Table 3), the latter could imply a larger life cycle climate impact due to a larger share of electricity generated from coal and gas (NEPP, 2020). It is clear that each alternative has its drawback and a tradeoff analysis between potential climate change, resource depletion and human health could be relevant support for the eventual choice. Another contributor to the increasing MDP values is the use of cobalt in SAF production. Cobalt, however, is arguably the most appropriate Fischer-Tropsch catalyst for SAF production (Dry, 2002), where further minimization of its use in the Fischer-Tropsch process is impractical. Alternative SAF production pathways such as alcohol-to-jet could be further investigated if the use of cobalt is deemed undesirable.
Implication of Feedstock Competition
Through the allocation of environmental burden to forest residue and black liquor, the sensitivity analysis (Section Sensitivity Analysis) demonstrates that feedstock competition could lead to higher GWP, ionizing radiation, MDP, toxicity impacts and land use. In the case of black liquor, it is however a mere indication of a shift of burden accountability from one sector to another, rather than an absolute increase in environmental burden. The potential environmental impacts associated with “100% SAF (BL)—burden borne” (Table 3) would have been emitted or used by the pulp mill even if black liquor is burden-free. This example highlights the limitation of allocation as a method to account for feedstock competition of co-products. In the case of forest residue, the analysis provides a reasonable indication of the possible outcomes of an intentional increase in the production of waste materials. As seen in Table 3, while the potential climate impact of the SAF allocated to burden-borne forest residue is still lower than that of jet fuel, its potential impacts on land use and toxicity could be comparatively higher. The observed effects might resonate with Bryngemark's (2019) analysis of competition for forest raw materials, where intense forest feedstock competition could lead to increased harvest levels, hence higher potential environmental impacts. Even though quantifying the potential increase in waste-based feedstock or potential substitutable materials is out of the system boundary of this study, our LCA illuminates the risk of higher potential environmental impacts due to the excessive demand for waste materials, thus highlighting the need for tight collaboration between sectors that are dependent on the common feedstock, to avoid any potential feedstock overuse. Alternatively, other waste-independent SAF technology pathways might be explored to compensate for the limitations of the highly competed feedstock in the overall production volume in Sweden.
Socio-Technical System Approach
The fact that electricity generation, green steel making, fossil-free concrete or feedstock competitions etc. are hitherto central in the discussion surrounding SAF production (see Sections Sensitivity Analysis, Climate Change Hotspot in Electrofuel Production, Potential Burden Shifting Effect, and Implication of Feedstock Competition) is a reminder of the complexity of a socio-technical system and its interdependency on factors beyond its system boundary (see Section SAF Technology Pathway Selection Step 1: Feedstock Types and Availability and Figure 5). One of the most important consequences of a systems approach is that it highlights the fact that fundamental changes are needed in the way that both SAF production and other industrial sectors would be affected (Jenkins, 1969). In particular, it demands that SAF production deployment be carried out on a more interdisciplinary basis and that industries in Sweden need to be organized in a more integrated way than before. In this sense, the systems approach facilitates LCA studies in accounting for the environmental sustainability of innovative technologies beyond their technical considerations and incorporating socio-political perspectives (Lai et al., 2022). Still, we have utilized a rather limited scope and dimensions of STS in our study, and our simplified version of actors' interdependency analysis could well mean that some actors or interactions are not accounted for. Also, our approach is purely qualitative and, therefore, does not include aspects that can be analyzed with quantitative methods, e.g., quantifying the future availability of feedstock types. For future research, it might be interesting to integrate the STS approach in a wider perspective, as well as put more emphasis on economic and social-cultural actors in the assessment.
Uncertainties
System boundaries, allocation methods, assumptions, technology projection and scenarios taken in the foreground and background systems are the main sources of contextual or model uncertainties in this study. Traditional statistical or analytical methods used to treat quantitative uncertainty in LCA are difficult to apply in prospective LCA, where quantification or statistical evaluation of uncertainties of future unknowns might be infeasible (van der Giesen et al., 2020). To a minor extent, uncertainties are treated by assessing different SAF technology pathways, testing allocation methods, energy sources (Section Sensitivity Analysis) and the use of expert findings published in the peer-reviewed literature in the upscaling of emerging technologies. Still, the results could be different should the choices of SAF technology pathways, technology projection methods and background scenarios vary. Thus, for future study, ranges and extreme scenarios such as fossil-free steel production, low carbon electricity production mix in the EU or other IAM shared socio-economic pathways (O'Neill et al., 2014) to represent the future background, etc. could be incorporated to better understand the implications and uncertainty associated with future development. Furthermore, the collection of primary data from the SAF value chain, if available, might also improve the accuracy of the life cycle inventory. The use of the cutoff approach in which system expansion induced by potential waste material diversion is not accounted for (Section Assumptions, Cut-Off, and Allocation) is a limitation of the study, particularly when system expansion is prioritized by ISO 14044 (2006) over allocation. Hence, we suggest that sensitivity analyses to understand the implication of system expansion, due to diversion of feedstock and substitution effect of co-products from SAF production, to be incorporated in the future LCA of SAF.
Conclusion
The study aimed to explore SAF technology pathways and quantify, from the perspective of fuel user, the potential environmental impacts that can be attributed to different SAF. A STS approach was employed to identify the future Swedish SAF feedstock options, hence leading to technology pathways, and a prospective LCA was performed to compare the life cycle global warming potential, mineral depletion potential, toxicity impacts, land use and ionizing radiation of jet fuel and SAF stemming from different technology pathways. Our analysis employs a cutoff approach to assume that the waste material such as forest residue and black liquor are available as burden-free resources, in which the impacts of potential feedstock competition or potential substitution effects induced by feedstock diversion are not quantified. This approach does not capture the system-wide consequences of the use of lignocellulosic-based SAF but rather answers the question “what impacts can be attributed to different SAF?”, applying the same system scope and delimitations as that of for instance the Ecoinvent cutoff database or the EU Renewable Energy Directive.
The potential climate impact attributed to non-CO2 species emitted during aircraft operation through the combustion of jet fuel or SAF dominated the analysis of the life cycle global warming potential of the aviation fuels, particularly in the short time horizon. The fact that SAF is not free from non-CO2 emissions prompted the urgency for further research and development, as well as policy measures to mitigate the potential impacts contributed by the non-CO2 species. Efforts to unify methodology in estimating non-CO2 cruise emissions from different types of SAF and devising metrics to represent such emissions are sought to both improve the understanding of SAFs' life cycle global warming potential and facilitate decision making.
Electricity generated by wind power, utilized either as a dedicated source or as part of the electricity grid, was identified as the major contributor to electrofuels' life cycle global warming, mineral depletion potential, toxicity impacts and land use potential. From a socio-technical system perspective, it is no surprise that steel, copper and concrete industries were found to be part of the underlying causes of electrofuels' less-than-desired environmental performance given that wind turbine construction was tied to these industries. The use of grid electricity might reduce the demand for mineral resources but could incur higher ionizing radiation. The potential environmental impact of future electrofuel production depends on whether and to which extent steel and concrete industries can become more environmentally sustainable as well as whether Sweden can meet the increasing demand for renewable energy in the future and address future tradeoffs. As long as the underlying systems or technologies are not fossil-free or low carbon, electrofuel production in Sweden might not achieve the life cycle environmental performances as desired.
Under the assumption that no burdens are allocated to waste material used as feedstock, lignocellulosic-based SAF could have a better environmental performance compared to jet fuel. In the cases when waste materials are attached with economic values, the potential environmental impacts including land use and toxicity allocated to the lignocellulosic-based SAF could exceed that of the jet fuel. For that matter, to take advantage of the potential environmental superiority that the lignocellulosic-based SAF could offer, a tight collaboration between waste feedstock users is suggested to avoid over-exploitation of resources.
Conceptualizing SAF feedstock supply as a sub-segment of a STS was a fruitful approach, showcasing how fuel feedstock could be wrapped in a web of relations with other agents as well as how co-evolution of several industrial sectors could unfold. In general, a STS approach could be beneficial for any technology-centric studies, underscoring the significance of cross-sectorial and interdisciplinary collaborations. Nevertheless, our use of the STS approach was limited and experimental in the current study, opening new avenues for future research. We encourage other scholars to join the debate and develop wider methodological approaches employing various STS approaches in LCA studies.
Data Availability Statement
The original contributions presented in the study are included in the article/Supplementary Material, further inquiries can be directed to the corresponding author.
Author Contributions
YYL: conceptualization, methodology, investigation, writing—original draft, and writing—review and editing. EK: methodology, supervision, and writing—review and editing. AB: conceptualization, supervision, and writing—review and editing. All authors contributed to the article and approved the submitted version.
Funding
This work was supported by the Swedish Energy Agency (Grant No. 50332-1).
Conflict of Interest
The authors declare that the research was conducted in the absence of any commercial or financial relationships that could be construed as a potential conflict of interest.
Publisher's Note
All claims expressed in this article are solely those of the authors and do not necessarily represent those of their affiliated organizations, or those of the publisher, the editors and the reviewers. Any product that may be evaluated in this article, or claim that may be made by its manufacturer, is not guaranteed or endorsed by the publisher.
Supplementary Material
The Supplementary Material for this article can be found online at: https://www.frontiersin.org/articles/10.3389/frsus.2022.912676/full#supplementary-material
References
Ail, S. S., and Dasappa, S. (2016). Biomass to liquid transportation fuel via Fischer Tropsch synthesis – technology review and current scenario. Renew. Sustain. Energy Rev. 58, 267–286. doi: 10.1016/j.rser.2015.12.143
Algunaibet, I. M., and Guillén-Gosálbez, G. (2019). Life cycle burden-shifting in energy systems designed to minimize greenhouse gas emissions: novel analytical method and application to the United States. J. Clean. Prod. 229, 886–901. doi: 10.1016/j.jclepro.2019.04.276
Arvidsson, R., Tillman, A.-M., Sandén, B. A., Janssen, M., Nordelöf, A., Kushnir, D., et al. (2018). Environmental assessment of emerging technologies: recommendations for prospective LCA. J. Indust. Ecol. 22, 1286–1294. doi: 10.1111/jiec.12690
ASTM (2021). ASTM D7566-20c: Standard Specification for Aviation Turbine Fuel Containing Synthesized Hydrocarbons. Available online at: https://www.astm.org/d7566-20c.html (accessed Feburary 27, 2022).
Bareiß, K., de la Rua, C., Möckl, M., and Hamacher, T. (2019). Life cycle assessment of hydrogen from proton exchange membrane water electrolysis in future energy systems. Appl. Energy 237, 862–872. doi: 10.1016/j.apenergy.2019.01.001
Bergek, A., Hekkert, M., Jacobsson, S., Markard, J., Sandén, B., and Truffer, B. (2015). Technological innovation systems in contexts: conceptualizing contextual structures and interaction dynamics. Environ. Innov. Soc. Trans. 16, 51–64. doi: 10.1016/j.eist.2015.07.003
Börjesson, P., Hansson, J., and Berndes, G. (2017). Future demand for forest-based biomass for energy purposes in Sweden. Forest Ecol. Manag. 383, 17–26. doi: 10.1016/j.foreco.2016.09.018
Bryngemark, E. (2019). Second generation biofuels and the competition for forest raw materials: a partial equilibrium analysis of Sweden. Forest Policy Econ. 109, 102022. doi: 10.1016/j.forpol.2019.102022
Bui, M. S., Adjiman, C., Bardow, A. J., Anthony, E. Boston, A., Brown, S., et al. (2018). Carbon capture and storage (CCS): the way forward. Energy Environ. Sci. 11, 1062–1176. doi: 10.1039/C7EE02342A
Christley, E., Karakaya, E., Frauke, U. In progress. Constructing Future Transition Pathways from Regime Expectations in the Aviation Industry (Working paper).
Cossutta, M., McKechnie, J., and Pickering, S. J. (2017). A comparative LCA of different graphene production routes. Green Chem. 19, 5874–5884. doi: 10.1039/C7GC02444D
Cousins, A., Cottrell, A., Lawson, A., Huang, S., and Feron, P. H. M. (2012). Model verification and evaluation of the rich-split process modification at an Australian-based post combustion CO2 capture pilot plant. Greenhouse Gases Sci. Technol. 2, 329–345. doi: 10.1002/ghg.1295
Cucurachi, S., van der Giesen, C., and Guinée, J. (2018). “Ex-ante LCA of emerging technologies,” in Procedia CIRP, 25th CIRP Life Cycle Engineering (LCE) Conference, 30 April – 2 May 2018. Copenhagen, 69, 463–468.
de Koning, A., Kleijn, R., Huppes, G., Sprecher, B., van Engelen, G., and Tukker, A. (2018). Metal supply constraints for a low-carbon economy? Resour. Conserv. Recycl. 129, 202–208. doi: 10.1016/j.resconrec.2017.10.040
Dry, M. E. (2002). The fischer–tropsch process: 1950–2000. Catalysis Today, Fischer-Tropsch Synth. Eve XXI Century 71, 227–241. doi: 10.1016/S0920-5861(01)00453-9
Ecoinvent (2022). System Models. Ecoinvent Database. Available online at: https://ecoinvent.org/the-ecoinvent-database/system-models/ (accessed February 27, 2022).
Ekvall, T., Björklund, A., Sandin, G., and Jelse, K. (2020). Modeling Recycling in Life Cycle Assessment (Final project report No. 47270–1). Strategic Innovation Programmes. Gothenburg: Swedish Life Cycle Center.
Elgowainy, A., Han, J., Wang, M., Carter, N., Stratton, R., Hileman, J., et al. (2012). Life-Cycle Analysis of Alternative Aviation Fuels in GREET (No. ANL/ESD/12-8). Energy Systems Division. Argonne National Laboratory.
Energimyndigheten (2021b). Proposal to the Swedish National Strategy for Hydrogen, Electrofuel and Ammonia (Förslag till Sveriges nationella strategi för vätgas, elektrobränslen och ammoniak) (No. ER2021:34). Bromma: Arkitektkopia AB.
Energimyndigheten (2021a). The Scenarios of the Swedish Energy System (2020). (Scenarier över Sveriges Energisystem 2020) (No. ER 2021:6). Bromma: Arkitektkopia AB.
EU (2018). Directive (EU) 2018/2001 of the European Parliament and of the Council of 11 December 2018 on the Promotion of the Use of Energy from Renewable Sources (Text with EEA Relevance. Brussels: EU, 328.
EU (2021a). Proposal for a Regulation of the European Parliament and of the Council on Ensuring a Level Playing Field for Sustainable Air Transport. Brussels: EU.
EU (2021c). Reducing Emissions from Aviation. Climate Action - European Commission. Available online at: https://ec.europa.eu/clima/eu-action/transport-emissions/reducing-emissions-aviation_en (accessed February 27, 2022).
EU (2022). EU Taxonomy: Complementary Climate Delegated Act. European Commission. Available online at: https://ec.europa.eu/commission/presscorner/detail/en/ip_22_711 (accessed February 28, 2022).
Fagerström, A., Grahn, D., Lundberg, S., Ghosh, S., Creaser, D., Olsson, L., et al. (2021). Large scale bio electro jet fuel production integration at CHP-plant in Östersund, Sweden (No. B2407). Stockholm: IVL.
Foit, S. R., Vinke, I. C., de Haart, L. G. J., and Eichel, R.-A. (2017). Power-to-Syngas: an enabling technology for the transition of the energy system? Angew. Chem. Int. Ed. 56, 5402–5411. doi: 10.1002/anie.201607552
Fossil Free Sweden (2021). Hydrogen Strategy for Fossil Free Competitiveness. Stockholm: Fossil free Sweden.
Freeman, S., Lee, D. S., Lim, L. L., Skowron, A., and De León, R. R. (2018). Trading off aircraft fuel burn and NOx emissions for optimal climate policy. Environ. Sci. Technol. 52, 2498–2505. doi: 10.1021/acs.est.7b05719
Garud, R., and Gehman, J. (2012). Metatheoretical perspectives on sustainability journeys: evolutionary, relational and durational. Res. Policy Spec. Sect. Sustain. Trans. 41, 980–995. doi: 10.1016/j.respol.2011.07.009
González-Castaño, M., Dorneanu, B., and Arellano-García, H. (2021). The reverse water gas shift reaction: a process systems engineering perspective. React. Chem. Eng. 6, 954–976. doi: 10.1039/D0RE00478B
Grigoriev, S. A., Fateev, V. N., Bessarabov, D. G., and Millet, P. (2020). Current status, research trends, and challenges in water electrolysis science and technology. Int. J. Hydr. Energy Prog. Hydrogen Prod. Utiliz. 45, 26036–26058. doi: 10.1016/j.ijhydene.2020.03.109
Gustafsson, K., Sadegh-Vaziri, R., Grönkvist, S., Levihn, F., and Sundberg, C. (2021). BECCS with combined heat and power: assessing the energy penalty. Int. J. Greenhouse Gas Control 108, 103248. doi: 10.1016/j.ijggc.2020.103248
Hansson, J., Hackl, R., Taljegard, M., Brynolf, S., and Grahn, M. (2017). the potential for electrofuels production in sweden utilizing fossil and biogenic CO2 point sources. Front. Energy Res. 5, 4. doi: 10.3389/fenrg.2017.00004
Hauschild, M. Z., Rosenbaum, R. K., and Olsen, S. I. (eds.). (2018). Life Cycle Assessment. Cham: Springer.
Huijbregts, M. A., Steinmann, Z. J., Elshout, P. M., Stam, G., Verones, F., Vieira, M. D., et al. (2016). ReCiPe 2016. A Harmonized Life Cycle Impact Assessment Method at Midpoint and Endpoint Level Report I: Characterization (No. RIVM Report 2016-0104). Bilthoven: National Institute for Public Health and the Environment.
ICAO (2019). Resolution A40-18: Consolidated Statement of Continuing ICAO Policies and Practices Related to Environmental Protection - Climate Change (ICAO resolution No. A40–18). Montreal, QC: ICAO.
IEA (2007). Black Liquor Gasification-summary and Conclusions from the IEA Bioenergy ExCo54 Workshop (Workshop report No. ExCo:2007:03). Rotorua: IEA.
Iribarren, D., Petrakopoulou, F., and Dufour, J. (2013). Environmental and thermodynamic evaluation of CO2 capture, transport and storage with and without enhanced resource recovery. Energy 50, 477–485. doi: 10.1016/j.energy.2012.12.021
ISO 14044 (2006). Environmental Management. Life Cycle Assessment. Requirements and Guidelines. First Edn. Geneva: International Organization for Standardization.
Jafri, Y., Furusjö, E., Kirtania, K., and Gebart, R. (2016). Performance of a pilot-scale entrained-flow black liquor gasifier. Energy Fuels 30, 3175–3185. doi: 10.1021/acs.energyfuels.6b00349
Jafri, Y., Wetterlund, E., Mesfun, S., Rådberg, H., Mossberg, J., Hulteberg, C., et al. (2020). Combining expansion in pulp capacity with production of sustainable biofuels – Techno-economic and greenhouse gas emissions assessment of drop-in fuels from black liquor part-streams. Appl. Energy 279, 115879. doi: 10.1016/j.apenergy.2020.115879
Jenkins, G. (1969). The Systems Approach. Birmingham: University of Lancaster, Department of Systems Engineering.
Kleijn, R., van der Voet, E., Kramer, G. J., van Oers, L., and van der Giesen, C. (2011). Metal requirements of low-carbon power generation. Energy 36, 5640–5648. doi: 10.1016/j.energy.2011.07.003
Koj, J. C., Wulf, C., Schreiber, A., and Zapp, P. (2017). Site-dependent environmental impacts of industrial hydrogen production by alkaline water electrolysis. Energies 10, 860. doi: 10.3390/en10070860
Kolosz, B. W., Luo, Y., Xu, B., Maroto-Valer, M. M., and Andresen, J. M. (2020). Life cycle environmental analysis of ‘drop in’ alternative aviation fuels: a review. Sustain. Energy Fuels 4, 3229–3263. doi: 10.1039/C9SE00788A
Kulanovic, A., and Nordensvärd, J. (2021). Exploring the political discursive lock-ins on sustainable aviation in Sweden. Energies 14, 7401. doi: 10.3390/en14217401
Kuparinen, K., Vakkilainen, E., and Tynjälä, T. (2019). Biomass-based carbon capture and utilization in kraft pulpmills. Mitig. Adapt. Strateg. Glob. Change 24, 1213–1230. doi: 10.1007/s11027-018-9833-9
Lai, Y. Y., Christley, E., Kulanovic, A., Teng, C. C., Björklund, A., Nordensvärd, J., et al. (2022). Analysing the opportunities and challenges for mitigating the climate impact of aviation: a narrative review. Renew. Sustain. Energy Rev. 156, 111972. doi: 10.1016/j.rser.2021.111972
Larson, E. D., Kreutz, T. G., Greig, C., Williams, R. H., Rooney, T., Gray, E., et al. (2020). Design and analysis of a low-carbon lignite/biomass-to-jet fuel demonstration project. Appl. Energy 260, 114209. doi: 10.1016/j.apenergy.2019.114209
Lee, D. S., Fahey, D. W., Skowron, A., Allen, M. R., Burkhardt, U., Chen, Q., et al. (2021). The contribution of global aviation to anthropogenic climate forcing for 2000 to 2018. Atmos. Enviorn. 244, 117834. doi: 10.1016/j.atmosenv.2020.117834
Lührs, B., Linke, F., Matthes, S., Grewe, V., and Yin, F. (2021). Climate impact mitigation potential of european air traffic in a weather situation with strong contrail formation. Aerospace 8, 50. doi: 10.3390/aerospace8020050
Markard, J., Raven, R., and Truffer, B. (2012). Sustainability transitions: an emerging field of research and its prospects. Res. Policy Spec. Sect. Sustain. Trans. 41, 955–967. doi: 10.1016/j.respol.2012.02.013
Mawhood, R., Gazis, E., Jong, S., de Hoefnagels, R., and Slade, R. (2016). Production pathways for renewable jet fuel: a review of commercialization status and future prospects. Biofuel. Bioprod. Biorefin. 10, 462–484. doi: 10.1002/bbb.1644
Mazzoni, R., Cesari, C., Zanotti, V., Lucarelli, C., Tabanelli, T., Puzzo, F., et al. (2019). Catalytic biorefining of ethanol from wine waste to butanol and higher alcohols: modeling the life cycle assessment and process design. ACS Sustain. Chem. Eng. 7, 224–237. doi: 10.1021/acssuschemeng.8b02959
Mendoza Beltran, A., Cox, B., Mutel, C., van Vuuren, D. P., Font Vivanco, D., Deetman, S., et al. (2020). When the background matters: Using scenarios from integrated assessment models in prospective life cycle assessment. J. Indust. Ecol. 24, 64–79. doi: 10.1111/jiec.12825
Molino, A., Larocca, V., Chianese, S., and Musmarra, D. (2018). Biofuels production by biomass gasification: a review. Energies 11, 811. doi: 10.3390/en11040811
Morgan, T. J., Youkhana, A., Turn, S. Q., Ogoshi, R., and Garcia-Pérez, M. (2019). Review of biomass resources and conversion technologies for alternative jet fuel production in Hawai'i and tropical regions. Energy Fuels 33, 2699–2762. doi: 10.1021/acs.energyfuels.8b03001
Moss, R. H., Edmonds, J. A., Hibbard, K. A., Manning, M. R., Rose, S. K., van Vuuren, D. P., et al. (2010). The next generation of scenarios for climate change research and assessment. Nature 463, 747–756. doi: 10.1038/nature08823
Mutel, C. (2017). Brightway: an open source framework for life cycle assessment. J. Open Sour. Software 2, 236. doi: 10.21105/joss.00236
NEPP (2020). Insigts and Pathways of Enegy Transitions (Insikter och vägval energiomställningen) (No. Slutrapport). Stockholm: NEPP.
Obnamia, J. A., MacLean, H. L., and Saville, B. A. (2020). Regional variations in life cycle greenhouse gas emissions of canola-derived jet fuel produced in western Canada. GCB Bioenergy 12, 818–833. doi: 10.1111/gcbb.12735
O'Malley, J., Pavlenko, N., and Searle, S. (2021). Estimating Sustainable Aviation Fuel Feedstock Availability to Meet Growing European Union demand (2021-13). Washington, DC: International Council on Clean Transportation.
O'Neill, B. C., Kriegler, E., Riahi, K., Ebi, K. L., Hallegatte, S., Carter, T. R., et al. (2014). A new scenario framework for climate change research: the concept of shared socioeconomic pathways. Clim. Change 122, 387–400. doi: 10.1007/s10584-013-0905-2
Pamula, A. S. P., Lampert, D. J., and Atiyeh, H. K. (2021). Well-to-wake analysis of switchgrass to jet fuel via a novel co-fermentation of sugars and CO2. Sci. Total Environ. 782, 146770. doi: 10.1016/j.scitotenv.2021.146770
Pavlenko, N., and Searle, S. (2021). Fueling Flight: Assessing the Sustainability Implications of Alternative Aviation Fuels (Working paper No. 2021–11). Washington, DC: ICCT.
Pei, M., Petäjäniemi, M., Regnell, A., and Wijk, O. (2020). Toward a fossil free future with HYBRIT: development of iron and steelmaking technology in Sweden and Finland. Metals 10, 972. doi: 10.3390/met10070972
Pettersson, K., Wetterlund, E., Athanassiadis, D., Lundmark, R., Ehn, C., Lundgren, J., et al. (2015). Integration of next-generation biofuel production in the Swedish forest industry – a geographically explicit approach. Appl. Energy 154, 317–332. doi: 10.1016/j.apenergy.2015.04.041
Piccinno, F., Hischier, R., Seeger, S., and Som, C. (2018). Predicting the environmental impact of a future nanocellulose production at industrial scale: application of the life cycle assessment scale-up framework. J. Clean. Prod. 174, 283–295. doi: 10.1016/j.jclepro.2017.10.226
Prussi, M., Lee, U., Wang, M., Malina, R., Valin, H., Taheripour, F., et al. (2021). CORSIA: the first internationally adopted approach to calculate life-cycle GHG emissions for aviation fuels. Renew. Sustain. Energy Rev. 150, 111398. doi: 10.1016/j.rser.2021.111398
Regeringskansliet (2020). Reduktionsplikt ska minska flygets klimatpåverkan. Regeringskansliet. Available online at: https://www.regeringen.se/pressmeddelanden/2020/12/reduktionsplikt-ska-minska-flygets-klimatpaverkan/ (accessed February 19, 2022).
Rotolo, D., Hicks, D., and Martin, B. R. (2015). What is an emerging technology? Res. Policy 44, 1827–1843. doi: 10.1016/j.respol.2015.06.006
Schenker, D. B. (2020). Transporting freight with train is a winner for the environment (Miljön vinnare när frakten går med tåg). Available online at: https://www.dbschenker.com/se-sv/om-oss/presscenter/nyheter-fr%C3%A5n-db-schenker/miljoen-vinnare-naer-frakten-g%C3%A5r-med-t%C3%A5g-635588 (accessed February 27, 2022).
Schmidt, O., Gambhir, A., Staffell, I., Hawkes, A., Nelson, J., and Few, S. (2017). Future cost and performance of water electrolysis: an expert elicitation study. Int. J. Hydr. Energy 42, 30470–30492. doi: 10.1016/j.ijhydene.2017.10.045
Schmidt, P., Batteiger, V., Roth, A., Weindorf, W., and Raksha, T. (2018). Power-to-liquids as renewable fuel option for aviation: a review. Chem. Ingenieur Technik 90, 127–140. doi: 10.1002/cite.201700129
Schulze, R., Abbasalizadeh, A., Bulach, W., Schebek, L., and Buchert, M. (2018). An Ex-ante LCA study of rare earth extraction from NdFeB magnet scrap using molten salt electrolysis. J. Sustain. Metall. 4, 493–505. doi: 10.1007/s40831-018-0198-9
Soone, J. (2020). Sustainable Aviation Fuels (Briefing No. PE 659.361 – November 2020). Rue Wiertz: European Parliamentary Research Service.
SSAB (2022). Timeline for HYBRIT and Fossil-Free Steel. SSAB. Available online at: https://www.ssab.com/company/sustainability/sustainable-operations/hybrit-phases (accessed February 27, 2022).
Stenkvist, M. (2021). The pre-conditions of wind power establishment (Nulägesbeskrivning - vindkraftens förutsättningar) (Prestudy). Bromma: Energimyndigheten.
Steubing, B., and de Koning, D. (2021). Making the use of scenarios in LCA easier: the superstructure approach. Int. J. Life Cycle Assess 26, 2248–2262. doi: 10.1007/s11367-021-01974-2
Steubing, B., de Koning, D., Haas, A., and Mutel, C. L. (2020). The activity browser — an open source LCA software building on top of the brightway framework. Software Impacts 3, 100012. doi: 10.1016/j.simpa.2019.100012
Tanaka, K., Peters, G. P., and Fuglestvedt, J. S. (2010). Policy update: multicomponent climate policy: why do emission metrics matter? Carbon Manag. 1, 191–197. doi: 10.4155/cmt.10.28
Trinh, J., Harahap, F., Fagerström, A., and Hansson, J. (2021). What are the policy impacts on renewable jet fuel in Sweden? Energies 14, 7194. doi: 10.3390/en14217194
Tsoy, N., Steubing, B., van der Giesen, C., and Guinée, J. (2020). Upscaling methods used in ex ante life cycle assessment of emerging technologies: a review. Int. J. Life Cycle Assess 25, 1680–1692. doi: 10.1007/s11367-020-01796-8
van der Giesen, C., Cucurachi, S., Guinée, J., Kramer, G. J., and Tukker, A. (2020). A critical view on the current application of LCA for new technologies and recommendations for improved practice. J. Clean. Prod. 259, 120904. doi: 10.1016/j.jclepro.2020.120904
Vattenfall (2019a). HYBRIT Orders Norwegian Electrolyzers for Fossil Free Steel Production in Luleå. Available online at: https://group.vattenfall.com/press-and-media/pressreleases/2019/hybrit-orders-norwegain-electrolyzers-for-fossil-free-steel-production-in-lulea (accessed February 27, 2022).
Vattenfall (2019b). Vattenfall and Cementa Take the Next Step Towards a Climate Neutral Cement. Available online at: https://group.vattenfall.com/press-and-media/pressreleases/2019/vattenfall-and-cementa-take-the-next-step-towards-a-climate-neutral-cement (accessed February 27, 2022).
Vattenfall (2022). How Does Approval Process for an Onshore Wind Park Work? (Hur fungerar tillståndsprocessen för en vindkraftpark på land?). Available online at: https://group.vattenfall.com/se/var-verksamhet/vindprojekt/faq-vindkraft/hur-fungerar-tillstandsprocessen-for-en-vindkraftpark-pa-land (accessed February 27, 2022).
Villares, M., Işildar, A., Mendoza Beltran, A., and Guinee, J. (2016). Applying an ex-ante life cycle perspective to metal recovery from e-waste using bioleaching. J. Clean. Prod. 129, 315–328. doi: 10.1016/j.jclepro.2016.04.066
Wei, H., Liu, W., Chen, X., Yang, Q., Li, J., and Chen, H. (2019). Renewable bio-jet fuel production for aviation: a review. Fuel 254, 115599. doi: 10.1016/j.fuel.2019.06.007
Wernet, G., Bauer, C., Steubing, B., Reinhard, J., Moreno-Ruiz, E., and Weidema, B. (2016). The ecoinvent database version 3 (part I): overview and methodology. Int. J. Life Cycle Assess. 21, 1218–1230. doi: 10.1007/s11367-016-1087-8
Yellishetty, M., Haque, N., and Dubreuil, A. (2012). “Issues and challenges in life cycle assessment in the minerals and metals sector: a chance to improve raw materials efficiency,” in Non-Renewable Resource Issues: Geoscientific and Societal Challenges, International Year of Planet Earth, eds R. Sinding-Larsen, and Wellmer (Dordrecht: Springer Netherlands), 229–246.
Zetterberg, L., Johnsson, F., and Möllersten, K. (2021). Incentivizing BECCS—a Swedish case study. Front. Clim. 3, 227. doi: 10.3389/fclim.2021.685227
Keywords: forest residue, black liquor, hydrogen, biogenic carbon dioxide, Fischer-Tropsch (synthesis), sustainability transition, emerging technologies, well-to-wake analysis
Citation: Lai YY, Karakaya E and Björklund A (2022) Employing a Socio-Technical System Approach in Prospective Life Cycle Assessment: A Case of Large-Scale Swedish Sustainable Aviation Fuels. Front. Sustain. 3:912676. doi: 10.3389/frsus.2022.912676
Received: 04 April 2022; Accepted: 09 June 2022;
Published: 07 July 2022.
Edited by:
Mikołaj Owsianiak, Technical University of Denmark, DenmarkReviewed by:
Daniel Fozer, Technical University of Denmark, DenmarkEleonore Pierrat, Technical University of Denmark, Denmark
Copyright © 2022 Lai, Karakaya and Björklund. This is an open-access article distributed under the terms of the Creative Commons Attribution License (CC BY). The use, distribution or reproduction in other forums is permitted, provided the original author(s) and the copyright owner(s) are credited and that the original publication in this journal is cited, in accordance with accepted academic practice. No use, distribution or reproduction is permitted which does not comply with these terms.
*Correspondence: Yat Yin Lai, eXlsYWlAa3RoLnNl