- 1Chemistry Department, Faculty of Science, Hacettepe University, Ankara, Turkey
- 2Department of Materials Science and Nanotechnology Engineering, Near East University, Mersin, Turkey
Water contamination by heavy metal pollution is induced by rapid industrialization and urbanization. Removal of heavy metal ions from wastewater is of prime importance for a clean environment and human health. The heavy metal problem is seriously threatened to human health. In addition, these metals are toxic and carcinogenic. They cause serious problems for aquatic ecosystems and especially humans. Different methods have been utilized to remove heavy metals from the wastewater, such as membrane filtration, adsorption, and ion exchange. Adsorption is one of the most efficient processes to clean contaminated water. The adsorption process presents advantages such as availability, low cost, and eco-friendly nature. The commercial adsorbents and the polymeric adsorbents are showed a high removal capacity for heavy metal ions capturing and removing from wastewater. Several researchers have widely used cryogels as a unique bio-adsorbent for heavy metals removal from wastewaters. Cryogels are efficient for the removal of heavy metal ions. This manuscript comprehensively and critically reviews current research in heavy metal adsorption by cryogels that prepared various technology and highlights the main advantages of these materials. Cryogels are synthetic polymers used in adsorption experiments in recent years. Because of their macropores, they provide an excellent advantage as an adsorbent in continuous and batch adsorption processes. The process applied for cryogel formation is called cryogelation. These macroporous gel matrices can be produced with different shapes and the gels are of interest in the bioseparation area since they can meet needs that conventional chromatographic media are less suitable to fulfill. The structure, synthesis, and composition of various cryogels are presented. Cryogels are generally synthesized by bulk polymerization under semifrozen conditions at −12 and 18°C for 12 to 24 h. The cryogels have a high removal capacity rate of over 90%. In the cryogels based studies, Fourier transforms infrared spectroscopy (FT-IR), scanning electron microscopy (SEM), thermal, surface area, elemental, and computerized microtomography (μCT) analyses can be used for the characterization of cryogels structure.
Introduction
In the past century, industrialization has accelerated, increasing the demand for indiscriminate exploitation of global resources, aggravating the globe's environmental pollution crisis (Briffa et al., 2020). Unlike other pollutants such as petroleum hydrocarbons and home and municipal waste, which can be seen in the environment, trace metals can start building up to dangerous amounts without being seen. Heavy metals are described as metallic elements with a higher density than that of water (Tchounwou et al., 2012). Heavy metals also include metalloids, such as arsenic, that can cause toxicity at low levels of exposure, based on the notion that heaviness and toxicity are linked (Duffus, 2002). Environmental contamination by these metals has been a growing problem for the environment and global healthcare in recent years. Furthermore, human contamination has increased drastically due to an exponential expansion in their use in various industrial, agricultural, residential, and technical applications (Braadl, 2005).
Heavy metals are particularly problematic since they are non-degradable and hence linger. Metals have long been abundant on Earth, and nearly 75% of the total of the elements in the periodic table are categorized as metals. Heavy metals make up most of the contaminants designated by the US Environmental Protection Agency as trace inorganic pollutants (Wuana et al., 2010). Heavy metals can be found in wastewater from industrial processes. Even at very low amounts in water, cadmium is a non-essential metal and a health concern (Manzoori et al., 2007). Although cobalt can be used to treat anemia, too much of it in the body might impair the hematological systems and cause skin reactions (Wang et al., 2018). Food and drinking water containing more than 30.0 μg/L of uranium is harmful to the kidneys (Liu et al., 2018). Environmental rules to limit heavy metal ion concentrations in water are getting more restrictive to eliminate these potentially adverse health impacts (Fu and Wang, 2011).
To protect communities and the planet, harmful heavy metal ions should be eliminated from wastewater. Chemical precipitation (Huisman et al., 2006; Özverdi and Erdem, 2006), ion exchange (Alyüz and Veli, 2009), adsorption (Burakov et al., 2018), membrane filtration (Hube et al., 2020), electrochemical treatment technologies (Ya et al., 2017), and other ways for removing heavy metal ions are all being used. The adsorption method has several advantages, including availability, cheap cost, and environmental friendliness. Commercial adsorbents and polymeric adsorbents demonstrated a high removal capability for capturing and removing heavy metal ions from wastewater. Several studies have employed cryogels as a novel bio-adsorbent for the removal of heavy metals from wastewaters.
When it comes to designing new adsorbents and separation methods, studying the adsorption mechanism is critical. The clarification of the heavy metal adsorption process on cryogels is a difficult task, and the literature typically approaches the topic using phenomenological and imprecise assumptions and models. Different adsorption mechanisms have been discussed in the literature, such as physisorption, which includes electrostatic interaction and attraction by van der Waals forces, metal coordination and complexation, ion exchange, and chemical sorption by chelation, or a good mix of these mechanisms (Baimenov et al., 2020b). Heavy metal adsorption on macroporous polymers is determined by the materials' structure and often involves many pathways (Khan and Lo, 2016). The functional groups, chelating agents, surface charge, and active components such as NPs all play important roles in the physicochemical properties of the surfaces. When it comes to selectivity and capacity, surface interactions with functional groups are the most important element (Baimenov et al., 2018). Amino-functionalized materials, for example, are effective at removing both anionic and cationic metal species; anionic species are removed via electrostatic interaction, ion exchange, or hydrogen bonding, whereas cationic species are removed via coordination with amino groups (Akilbekova et al., 2018).
There is a big demand for improved technologies concerning the simple processing of cryogels. Recent developments in macroporous cryogels fabricated at subzero temperatures have demonstrated efficiency for processing environmental applications. Cryogels have been demonstrated to have perfect mass transfer characteristics, enabling solutions of high flow rates to be passed through them with low resistance. Their unique combination of properties such as biocompatibility, macroporosity, physical stability, chemical stability, and ease of preparation, renders these materials interesting candidates for a wide range of potential water contamination applications. This chapter summarizes current heavy metal adsorption studies using cryogels manufactured using diverse technologies and the primary benefits of these materials. Synthesis, characterization, applications, and heavy metal removal of cryogels are summarized in the following sections.
Cryogels
Cryogels are three-dimensional cross-linked structures made by reacting monomer or polymer solutions at low temperatures below the solvent's boiling point (Saylan and Denizli, 2019). The gel system is considered a potential scaffold material for many applications because of the cryogel system's unique composition of highly porous properties, adequate osmotic stability, and mechanical strength. The gel system is considered a potential scaffold material for many applications. Önnby summarized the application of cryogels in wastewater and water (Önnby, 2016), where biomedical applications were reviewed by Memic et al. (2019). The latest reviews were done by Baimenov et al. (2020b) which summarizes the removal of heavy metals by cryogels from aqueous solutions and by Savina et al., related to with the application of biodegradable cryogels as drug carrier and tissue engineering materials (Savina et al., 2021). The most crucial feature of cryogels is their synthesized simplicity and an aqueous solvent(s) for cryogel syntheses, making them suitable for a wide range of biotechnological and environmental applications (Akgönüllü et al., 2017).
Although there has been a rise in interest in cryogelation over the last four decades, the earliest observation of network architectures forming after freezing dates back over a century (Lottermoser, 1908). When Mahler et al., froze silica gels to generate fibers, they were the first to use ice-templating to obtain specified topologies (Mahler and Bechtold, 1980). Soon after, the ability to process porous polymers was established (Vainerman et al., 1981; Lozinsky et al., 1982), and ceramists could create equivalent macroporous ceramic structures after a decade (Fukasawa et al., 2001). The procedure's adaptability sparked renewed interest in cryogelation, culminating in an increasing number of publications published in the recent decade.
Understanding the mechanism of cryogel production and describing the macroporous architecture of cryogels were the original goals of the initial research. After the supermacroporous cryogels were described using known techniques, researchers began looking for new ways to use these matrices. The first few uses of cryogels, according to the literature, were based on the immobilization of bacterial cells and enzymes onto cryogel matrices (Lozinsky et al., 1989). Cryogels became popular in the 1990s when many groups worldwide began to utilize the supermacroporous materials' physicochemical features. These macroporous gel matrices have been widely used in biomedical and biotechnological fields (Memic et al., 2019), including chromatography, immobilization of biomolecules and cells, molecular imprinting, environmental technology (Önnby, 2016; Baimenov et al., 2020b), tissue engineering (Savina et al., 2021), and therapeutical purposes drug discovery and screening of hazardous materials (Saylan and Denizli, 2019).
Cryogels Synthesis
According to Lozinsky and Okay, Gels are systems of a polymer and an immobilized solvent where the macromolecular chains are linked together by chemical or physical connections to form a three-dimensional network (Lozinsky and Okay, 2014). When the used solvent is water, we're talking about hydrogels; when the solvent is an organic liquid, we're talking about organogels. Once the solvents are water–organic combinations, several intermediate versions are undoubtedly feasible. Gels are covalent gels, ionic gels, noncovalent or physical gels, or mixed variations based on the interchain connections between polymers forming the structure. The molecular structure of the individual polymers and the methodology of gel formation specify the overall morphology of the gel.
Although extensive research, the engineering and control of gel-based systems still pose some challenges owing to the inverse coupling of a set of network features. When the extent of crosslinking in gels is reduced to maximize the mesh size, the gels degrade more quickly. Furthermore, when processed in the swelled form, weakly crosslinked gels are delicate materials; they often break at very modest pressures due to the absence of the adequate energy dissipation pathway in the gel matrix. Furthermore, in many applications, the interaction rate of gels to different influences is not rapid enough. So, the kinetics of gel structural change requires the solvent's polymer system absorption or desorption, and it is a diffusive mechanism. This is a slow methodology that becomes slower as it approaches the critical point. As a result, in many current and proposed gel applications, the design of gels with strong mechanical properties and a quick response rate is critical. Interconnected pore structures are needed to convert the diffusive mechanism into convective to overcome this problem. Table 1 has been shown different techniques for macroporous gel formation.
According to basic technology, the monomer–crosslinker mixture is copolymerized by free-radical crosslinking in the presence of an inert material (named as porogen or diluent), which is soluble in the monomer mixture (Kanamori et al., 2007). A phase separation needs to occur through the network creation process to create macroporous structures, and the development of further crosslinks should fix the two-phase structure. The inert material is withdrawn from the network following polymerization, producing the pores inside the strongly crosslinked polymer network. This reaction-induced phase separation mechanism of porosity creation results in macroporous gels made up of agglomerates of polymer pieces of varied sizes. Although phase-separated microporous gels respond quickly to external stimuli, their aggregate-like shape eventually considerably loses mechanical characteristics (Sayil and Okay, 2001). Furthermore, the network chains do not behave like flexible polymer chains sensitive to external stimuli since a considerable amount of crosslinker is required to generate phase separation during gelation.
Cryogels are gel architectures that form in substantially frozen liquids or colloidal dispersions of components suitable for gelling, as shown in Figure 1. Solvent crystals are employed as porogen in the cryogelation process. Solvent crystals that have been defrosted succeed in large, interconnecting pores. Cryogelation is a cost and workforce economical way for producing macroporous gels compared to other methods (Plieva et al., 2011). Cryogels also have the advantage of being flexible in their production and functioning primarily with water as a solvent, enabling this type of material more cost-effective, and ecologically responsible (Rie et al., 2015). Moreover, as stated in a good study by Henderson et al., many parameters can be easily fine-tuned to create cryogels for their particular usage (Henderson et al., 2013). The type of cross-linker, polymer molecular weight, cryo-concentration, cryogelation temperature, and cooling rate are all variables that can be altered.
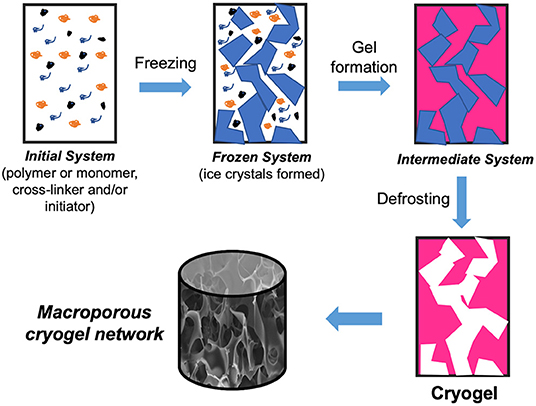
Figure 1. Cryogel fabrication scheme: initial system (gel starting material is cooled to below freezing point); frozen system (ice crystals form and solutes concentrate in non-frozen zones); intermediate system (gel is created in the non-frozen liquid between ice crystals); cryogel is formed after defrosting.
The main critical parameters impacting the distinctive features of the produced cryogels are the content and concentration of the starting solution, freezing temperature, cooling rate, type of crosslinker, the molecular weight of the polymer and incubation period. Adjusting any of the manufacturing variables throughout the cryogelation procedure will modify the morphology of the cryogel, such as polymer wall thickness, pore size, pore structure, and porosity (Bakhshpour et al., 2019; Akgönüllü et al., 2020b). The structural characteristics of the generated cryogels can be influenced by the number of monomers in the starting solution. The cryogel exhibits a decreased pore size as the starting concentration increases due to the creation of thicker sidewalls, resulting in a stiff and less spongy architecture. In general, the beginning monomer exists in a non-frozen phase, and its concentration influences the size of that phase, resulting in the creation of the cryogel's resultant polymeric wall. As a consequence, a bigger non-frozen component will develop, with a reduced porous structure and thicker pore sidewalls (Kirsebom et al., 2010).
Temperature and time of cryogel formation have a significant impact on the pore structure of the cryogel, including the shape, size, and density of the pores. Polymerization at lower freezing temperatures produces smaller and more frequent ice crystals, resulting in cryogels with smaller hole diameters. Furthermore, if the temperature is not low enough, the solution will be non-frozen, resulting in the creation of an undesired architecture. In the macrometer region, a temperature range of 10 to 21°C and a freezing time of 24 h is stated to give an ideal linked ice crystal structure (Bäcker et al., 2016).
The cooling rate is another parameter that affects the interconnected macroporous structure of the cryogel. Polymerization will proceed in the absence of porogens (solvent crystals) and a cryogel with smaller pores will be formed instead of a highly interconnected cryogel if cross-linking happens much faster than ice crystal formation. Lower cooling rates result in bigger ice crystal formation, resulting in a cryogel structure that is densely linked and macroporous (Memic et al., 2019).
The cross-linking process is carried out in one of two ways: chemical or physical. Covalent reactions and cross-linking of monomers or prepolymers are used to create chemical cross-linking. At the cryogelation temperature, chemically cross-linked cryogels are generated when reactive species (monomers, prepolymers) polymerize between ice crystals. Physical cross-linking, on the other hand, is caused by ionic or secondary interactions between polymer chains. Physical cross-linking can be used to create cryogel scaffolds, but the resulting macropores are typically tiny (<10 μm) with poor gel properties (like brittleness and stability) which can be limiting issues in some applications. In contrast, chemically cross-linked cryogels are generally more physically stable and have more defined and predictable features.
The viscosity and chain entanglement of a polymer can be affected by its concentration and molecular weight. Changes in any of these factors, therefore, can result in considerable variances in the final cryogel structure. Lower molecular weight polymers, in general, result in the creation of bigger holes within the final cryogel at a certain polymer concentration. The free water concentration in the polymer solution drops as the molecular weight of the polymer increases, resulting in smaller holes and thicker polymer walls inside the manufactured cryogels. Furthermore, increasing the polymer concentration at a constant molecular weight increases the availability of crosslinking groups while decreasing the availability of free water, resulting in smaller cryogel pore sizes (Plieva et al., 2011).
Cryogels formed from a singular precursor, such as a natural or synthetic polymer, are the basic (Lozinsky, 2002; Ulery et al., 2011). Polymers originating from plants or animals, such as polyesters, polysaccharides, and proteins, are examples of natural polymers (Volova et al., 2017). These polymers closely resemble the extracellular matrix's chemical and physical features. 3D architectural scaffolds made from naturally generated polymers have subsequently gotten a lot of interest for their ability to support mammalian cell development and proliferation in vitro and in vivo (Kumbar et al., 2014). Alginate (Textile, 2017), cellulose (Ciolacu et al., 2016), collagen (Brougham et al., 2017), chitosan (Ji et al., 2011; Biswas et al., 2016), gelatin (Barbetta et al., 2009; Demir and Bölgen, 2017), hyaluronic acid (Oelschlaeger et al., 2016), starch (Bagri et al., 2011) and silk (Ak et al., 2013; Yetiskin and Okay, 2017), as well as, rubber (Oztoprak and Hekimoglu, 2014), and their derivatives, have all been used successfully in cryogel manufacturing. Several synthetic polymers have variable physical and degrading features, making them suitable for a wide range of biotechnological applications (Kumbar et al., 2014). Because of their ability to a breakdown in biological conditions, poly(l-lactic) (PLLA) (Conoscenti et al., 2017), poly(ethylene glycol) PEG (Bäcker et al., 2016; Wang and Yang, 2018), poly(vinyl alcohol) (PVA) (Jeong et al., 2015; Ceylan et al., 2016; Göktürk et al., 2016), and poly(hydroxyethyl methacrylate) (PHEMA) (Bereli et al., 2012) are among the most commonly used synthetic polymers for the creation of cryogels. The main disadvantages of synthetic polymers in biotechnological applications are the lack of bioactivity and bioadhesive qualities. This drawback can be overcome by covalently connecting short synthetic peptides and/or numerous bioadhesive functionalities during cross-linking or combining them with natural polymers (Caló et al., 2016). Bäcker et al. (2016) describe the production and characterization of cryogel combinations containing polyhydroxyethyl methacrylate, alginate, gelatin, and poly(ethylene glycol) diacrylate (pHAG–PEGda) and their effects on cultivating the epithelial prostate cancer cell line LNCaP. Ingavle et al. (2015) investigated the binding efficiencies of protein A, monoclonal antibodies to the cryogel column by chemically coupling the affinity ligand (protein A) to reactive hydroxyl and epoxy-derivatized monolithic cryogels. Newland et al. (2015) used EDC/sulfo-NHS driven crosslinking of amino terminated starPEG and Alexa 647 tagged heparin to create cryogel microcarriers.
Cryogels are very stable, capable of heavy metal removal, easy to prepare, economical to synthesize and can be manufactured in large quantities with good reproducibility. Separation and purification techniques based on these materials have received much attention recently. Owing to these advantages, they are widely used in many applications such as heavy metal removal, purification of biomolecules, drug delivery systems, antibacterial materials (Bakhshpour et al., 2018, 2020; Diken Gür et al., 2021).
Cryogels Characterization
Cryogels' characteristics, such as their elasticity, swelling, and interconnecting pore structures, can all play a part in determining their stability and, subsequently, their use. Cryogels typically offer improved mechanical characteristics, macroporosity, and swelling capability than other hydrogels, which has driven their use in different applications. Orakdogen and Boyaci presented about how to make pH-responsive nano-sized composite hydrogels and cryogels that have better mechanical properties (Orakdogen and Boyaci, 2016). Ari and Sahiner conducted the structural, thermal, and morphological characterization of inulin cryogels by following the previously published literature on hyaluronic acid (HA) cryogels (Ari and Sahiner, 2020). Cryogels may quickly return to their original shape and size following mechanical compressions, such as syringe injecting, because of these unique properties, making them a crucial consideration when designing materials for various specialized applications (Bencherif et al., 2012). A variety of approaches for determining these critical physical qualities and characterizations are covered below.
Structural and Morphological Characterization
Nitrogen porosimetry is commonly used to investigate porous materials' micro-and mesoporous structures. For the estimation of the surface area, pore-volume, and pore size variation, the nitrogen adsorption-desorption isotherms are combined with Brunauer-Emmett-Teller (BET), Density Functional Theory (DFT), and Barrett, Joyner, and Halenda (BJH) equations (Onorevoli et al., 2018). Due to the existence of micropores in the polymer walls, only the BET equation can be used to determine the specific surface area because polymeric cryogels are super-macroporous materials by nature (Tekin et al., 2011; Baydemir et al., 2014; Kim and Lee, 2019a). Poly(HEMA-VIM)/poly(HEMA) composite cryogel was made by embedding poly(HEMA-VIM) particles generated with suspension polymerization into poly(2-hydroxyethyl methacrylate), poly(HEMA) cryogel to improve surface area (Tekin et al., 2011). Using multipoint BET measurements, the surface area of poly(HEMA-VIM) cryogel was found to be 39.7 m2/g, while the surface area of poly(HEMA-VIM)/poly(HEMA) composite cryogel was found to be 78.6 m2/g. Baydemir et al., used BET analysis to determine how adding PHEMAPA beads improved the surface area of the cryogels (Baydemir et al., 2014). Using BET analysis, Kim and Lee demonstrated that the functionalized p(AA-co-MA-co-AAc) cryogel had a smaller specific surface area and pore volume than the p(AA-co-MA-co-AAc) cryogel (Kim and Lee, 2019a). Some researchers employed mercury porosimetry to estimate the specific surface area of macroporous cryogels (Jain and Kumar, 2009). Using a cryogelation process, glutaraldehyde (GA) cross-linked poly(vinyl alcohol)/activated carbon (PVA/GA/AC) composites were made in the form of monolithic rods by Zheng et al. (2012) and the mercury porosimetry measurements reveal that narrow macropores in the same size range play a substantial role. However, using this procedure does not ensure reliable data because substantial structural changes and even complete loss of the whole network of pores can occur throughout the drying of cryogels during the study on mercury porosimeter (Gun et al., 2013). Furthermore, because cryogels are formed of soft matter, shirking off the cryogels occurs when the intrusion/extrusion of mercury under high pressures, affecting the accuracy of the research.
One of the essential qualities essential to separation methods is fluids' permeability and flow rate within hydrogels (Aşir et al., 2005). Because of their interconnecting macropores, monolithic cryogel tools have been described as potential filters for water treatment. This allows for unrestricted solute flow and diffusion (Alkan, 2015). Andrabi et al. (2016) created a supermacroporous cryogel column of chitosan-dimethylaminoethyl methacrylate (DMAEMA)-magnetite. The cryogels' large interconnecting holes with porosity >85% allow for rapid solvent transport. The cross-linker ratio, the type of monomers, and the size of the cryogel column all have an impact on permeability.
When a cryogel absorbs a solvent, the swelling ratio quantifies the proportional increase in weight. When cryogels swell, the holes within the network of cryogels quickly fill with the solvent. A portion of the solvent also diffuses across the polymer walls. Because of their vast pores and excellent pore interconnectivity, cryogels have a substantially larger swelling capacity than typical hydrogels (Bencherif et al., 2012). Gels that have been fully hydrated and swelled frequently have a rubbery and soft texture, having reduced interfacial tension with body fluid, which is a significant property for biological applications. The degree of cryogel cross-linking has been demonstrated to impact cryogel swelling (Shih et al., 2018) significantly. The degree of cross-linking is thought to alter the density of the polymer walls inside the cryogel, which in turn affects the scaffolds' ability to absorb a certain amount of solvent.
The most basic method for studying the macroporosity of cryogels is optical microscope imaging. Cryogels are widely studied using microscopic approaches. Sur et al. (2012) for example, used numerous microscopic approaches to characterize nanofibers containing collagen and amphiphile peptide (SEM, TEM, AFM, cryo-TEM, and confocal reflection microscopy). Even though various microscopic approaches were used, only the surface area was estimated using quantitative analysis of microscopic pictures. The majority of microscopic image assessment has been performed on a qualitative or semi-quantitative basis.
Two critical parameters in adsorption studies of cryogels are the point of zero charges (pHPZC) and isoelectric point (IEP) (Gun et al., 2013). The pH where the surface charge of the material is zero is known as the point of zero charges (Gulicovski et al., 2015a). When the pH is less than pHPZC, the adsorbent has a positively charged surface, while when the pH is more than pHPZC, the adsorbent has a negatively charged surface (Önnby et al., 2015). As a result, when the solution pH is more than pHPZC, a cationic adsorbate has a higher affinity for the adsorbent and vice versa. The isoelectric point is another word for the electrokinetic phenomenon. In the absence of selective adsorption on the surface, the isoelectric point corresponds to the pH at which the electrokinetic potential equals zero. Potentiometric and conductometric titrations usually calculate IEP with acidic and basic solutions or use zeta potential calculating equipment (Tatykhanova et al., 2012). Dragan et al., developed novel composite sorbents containing chitosan (CS) and a synthetic polycation, poly(vinyl amine) (PVAm), in an 80:20 w/w ratio, dual-cross-linked with glutaraldehyde (GA) and ethylene glycol diglycidyl ether (EGDGE), and microspheres of porous strong base anion exchangers with (vinylbenzyl diethyl-2-hydroxyethyl)ammonium chloride functional groups. Potentiometric titration from acidic to basic pH was used to measure the composite cryobeads' pHPZC before and after loading with Cr(VI) (Dragan et al., 2017).
Mechanical Characterization
Cryogels must strike a compromise between porosity and mechanical properties, both a problem and an important characteristic. Low porosity causes mechanical imbalance and resistance to fluid flow through the cryogel, whereas high porosity impairs mechanical characteristics. Cryogels' high elasticity and good mechanical qualities allow them to be used in various circumstances, enhancing their reusability in adsorption purposes. Compression study using uniaxial stress (Young's modulus) is commonly used to evaluate the mechanical characteristics of cryogels. Berillo et al., found that non-chemically cross-linked cryogels based on chitosan and gold complexes had an elasticity of 1.03–6.7 kPa, equivalent to covalently cross-linked cryogels. The same study discovered that keeping samples in the frozen condition for longer increases their Young's modulus, linked to low ligand concentrations and a delayed ligand exchange mechanism (Berillo et al., 2014).
It was discovered that adding diamino hexane to the p(Jeff600-co-GA) enhances the cryogel's Young's modulus by increasing the length of the polymer chain (Erdem et al., 2017). Young's modulus of p(SA) and p(SA)-Ag NPs cryogels with Ag concentration of 167 mg/g was compared by Loo et al. (2014). Young's modulus was found to increase from 2.6 to 3.2 kPa when cryogels were modified. Savina et al., investigated the mechanical properties of p(HEMA-co-PEGDA) cryogels. Composite cryogels [p(HEMA-co-PEGDA)/Fe2O3] and [p(HEMA-co-PEGDA)/Fe3O4] (h = 10 mm and d = 10 mm) crushed up to 85% with the highest loading of 18 N and discovered that no structure degradation occurs (Savina et al., 2011). Young's modulus for p(HEMA-co-PEGDA) was 16.4 ± 1.9 kPa, while it was 46.8 ± 4.9 kPa and 57.6 ± 10.8 kPa for the composite p(HEMA-co-PEGDA)/Fe2O3 and p(HEMA-co-PEGDA)/Fe3O4 cryogels, respectively. Shu et al. (2017) showed that immobilizing TiO2 nanopowder inside a cryogel's structure improved its flexibility and compressive strength. The Young's modulus of the preliminary p(AAM) cryogel (h = 10 mm and d = 10 mm) was 36.23 ± 0.82 kPa, whereas the TiO2 impregnated cryogel had Young's modulus of 44.48 ± 1.21 kPa.
Cryogel Applications
Cryogels can be used as a carrier for heterogeneous catalysts (Sahiner and Seven, 2014) or biocatalysts (Hedström et al., 2008), as a solid phase matrix in chromatography for the isolation or removal of (bio)macromolecules like enzymes (Efremenko et al., 2006), proteins (Bereli et al., 2011; Andaç et al., 2012), nucleic acid (Odabaşi et al., 2010), cells (Lozinsky, 2002) and contaminants (Plieva et al., 2003) as a drug carrier system (Kostova et al., 2011), or as a scaffold for tissue engineering (Plieva and Mattiasson, 2007; Savina et al., 2007; Dainiak et al., 2010) due to their unique structure. Cryogels offer effective delivery of nutrients and elimination of metabolomics waste in cell growth due to their tissue-like flexibility and good flow-through qualities.
In their review, Ertürk and Mattiasson (Ertürk and Mattiasson, 2014) provide an in-depth look at the various applications of cryogels in bioseparation. In summary, large pores, low-pressure drop, short diffusion route length, and brief residence periods of biomolecules in the column are all critical requirements for using cryogel matrices as stationary phases. Fluids are transported through macropores, while sorption and chemical reactions occur on the cryogel walls. Because of their wide pores, cryogels have a modest internal surface area. In comparison to packed-bed or monolithic columns, this reduces their binding capability. Several techniques have been devised, including graft polymerization on the interface of the gels (Yao et al., 2007; Bibi et al., 2013), sequential freeze-thawing procedure (Galaev et al., 2008), and the creation of composite cryogels using a particle embedding method to solve this problem (Erzengin et al., 2011; Koç et al., 2011).
These materials exhibit the optimum kinetic properties in fixed-bed applications due to their linked 3D porous structure with a wall thickness of only a few microns. Furthermore, cryogels' enormous pore sizes enable the creation of organic-inorganic cryogels for the selective removal of extremely hazardous metal and metalloid ions without obstructing solution flow in monoliths. The adsorptive performance of the cryogel adsorbents toward different heavy metals via batch and fixed-bed modes is discussed in the following sections.
Heavy Metals
Various industries commonly release heavy metals in the wastewater. The surface treatment practices, tannery, leather, textile, paint, dyes & pigment, petroleum refining industries, wood processing, photographic-film production, and also electroplating cause the pollution of the wastewater with heavy metals such as lead, zinc, chromium, copper, platinum, titanium, cadmium, nickel, vanadium, and silver (Renu and Singh, 2017). The toxicity of these metals badly and heavily affects humans and animals and causes irreversible damage, life-threatening illness, and physical discomfort to the vital body system (Zhao et al., 2018).
There are two categories for sources of entering the heavy metal into the water and wastewater. The first category is artificial ways, including urban wastewater, wastewater and industrial waste, and the second is natural ways, including rainfall, dissolution of soluble salts, and soil erosion. However, the leading cause of these pollutants is the increase of the various industries and urban population. Nowadays, 35 elements are classified as heavy metals, that 23 elements are known as the most toxic metals. Some factors determine the toxicity of heavy metals, such as the biological role of the metals and the type of metal in the life cycle (Hasanpour and Hatami, 2020). Mercury, lead, arsenic, cadmium and arsenic are the common metals that perform a significant role in human poisoning. On the other hand, a small amount of chromium, zinc, and copper need for the human body, but large quantities of these elements can be caused human poisoning. Table 2 is shown the summaries of the sources, toxic effects and the standard concentration of some heavy metals on human health.
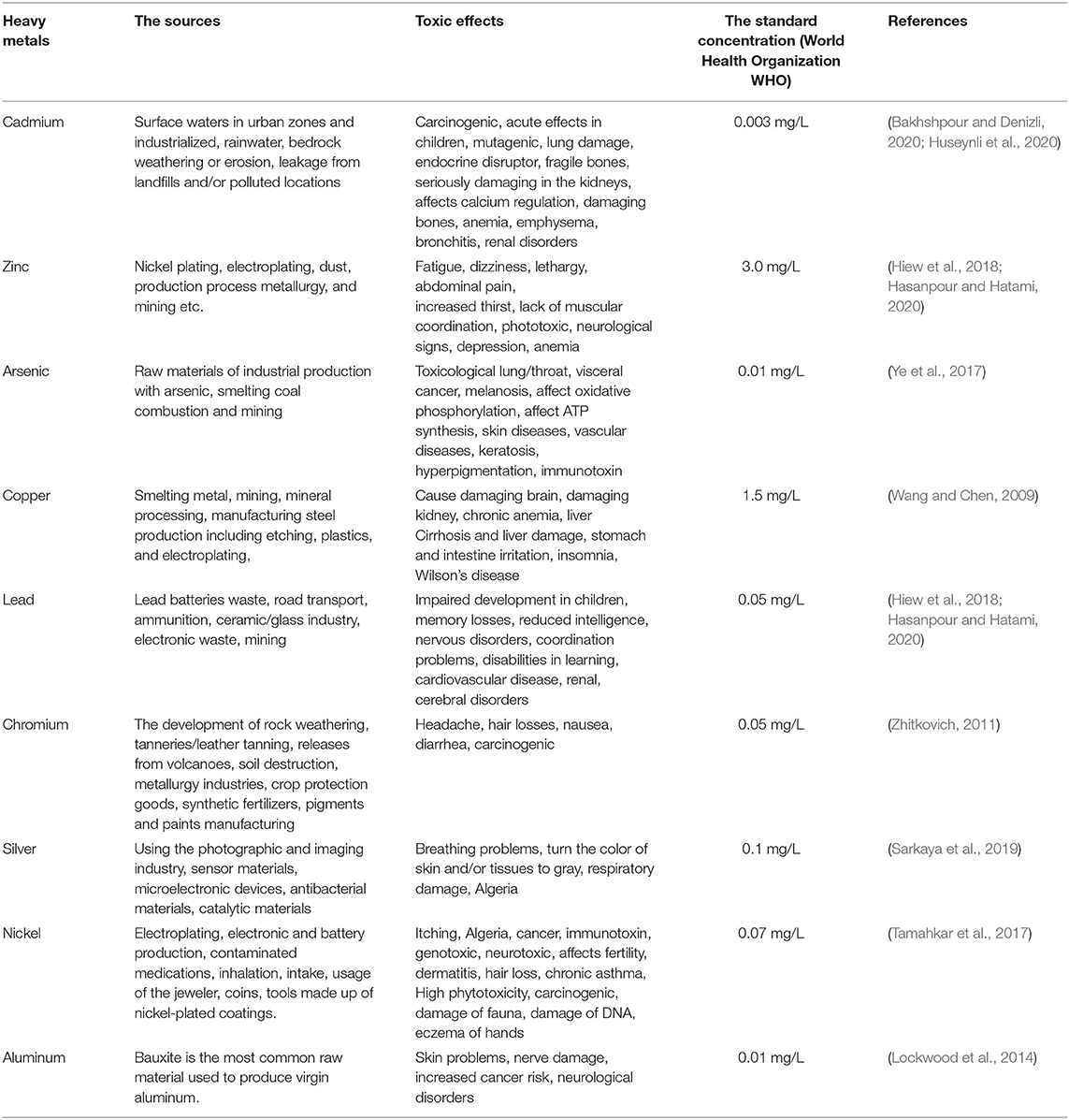
Table 2. The summaries of the sources, toxic effects and the standard concentration of some heavy metals on human health.
Removal Of Heavy Metal Ions From Wastewater
Removal of Cd
Cadmium (Cd) a highly toxic heavy metal ion found naturally in the environment. Cd(II) ions removal is studied with a composite polymeric system. Cysteine based polymeric beads and cryogels were utilized for Cd(II) ions removal. Here, two different molar ratios of cysteine based functional monomer (1:1 and 2:1) were employed. SEM and FTIR were used for the structural characterization of the prepared cryogels. The adsorption studies of Cd(II) ions were obtained in different initial concentrations of Cd(II), pH, and temperature. According to these experiments, the authors reported 76.35 and 98.8 μmol/g maximum adsorption amount for 1:1 and 2:1 molar ratio ion-imprinted composite cryogels. In addition, the adsorption capacity of Cd(II) removal from synthetic wastewater was reported as 98.8% (Huseynli et al., 2020). Tabakli et al. (2015) developed particles embedding ion-imprinted cryogels for removal of Cd(II) ion. Ion-imprinted polymer-based cryogels are interesting adsorbents that have been synthesized for the specific removal of heavy metal ions from effluents. 2-hydroxyethyl methacrylate (HEMA) was used as a monomer, and N-methacryloyl-L-cysteine methyl ester (MAC) was used as a functional monomer in the particles system. Then, Poly(HEMA-MAC) particles were embedded into the cryogel network. They reported that these cryogels showed high removal capacity and affinity against Cd(II) ions. The experimental maximum removal capacity was calculated as 32.15 μg/g polymer. Finally, the selectivity of ion-imprinted particles embedded cryogels was examined using heavy metal ions such as Cd(II), Pb(II), and Zn(II). When the results were examined, it was reported that ion-imprinted-based cryogels recognized Cd(II) ions with higher selectivity. SEM image of Poly(HEMA-MAC) cryogels and selectivity result was shown in Figure 2.
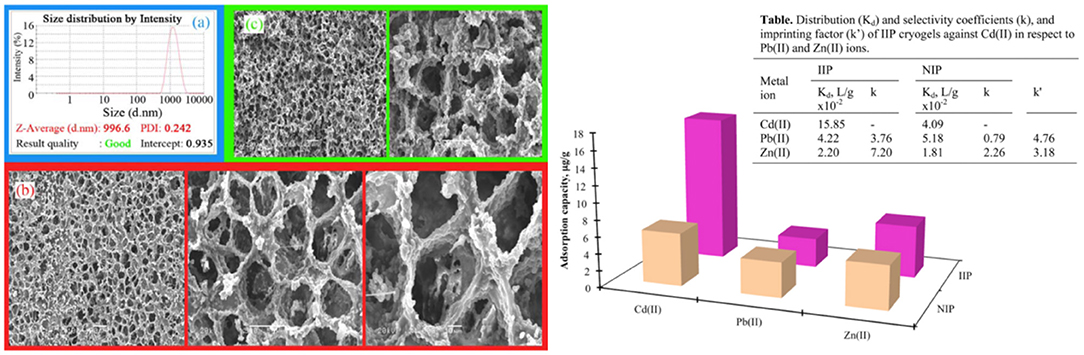
Figure 2. SEM images poly(HEMA–MAC) particles embedded cryogel and selectivity result: Cryogelation conditions: at −12 °C for 24 h and thawing was performed at room temperature along with washing with 20 mL of deionized water (Tabakli et al., 2015).
In another study, Baimenov et al. (2020a) synthesized amphoteric cryogels by the use of free-radical co-polymerization of acrylate-based precursors (methacrylic acid and 2-acrylamido-2-methyl-1-propansulfonic acid) with allylamine at different ratios. They characterized the cryogels using FT-IR, SEM/EDX, XPS and zeta potential measurements. The prepared cryogels were used to remove Cd(II) ions from aqueous solutions at various pH and initial concentrations. Equilibrium studies revealed a maximum sorption capacity in the range of 132–249 mg/g. Leaching experiments indicated the stability of Cd(II) in the cryogel structure. They used these cryogels to compare commercially available adsorbents for the removal of Cd(II) from various water matrices such as tap water, river water and ultrapure water. As a result, under the experimental conditions used, the cryogels can be more effective adsorbents.
N-acetylcysteine attached poly(acrylamide-co-methyl methacrylate) [poly(AAm-MMA)] cryogels were synthesized by Evli et al., for sensitive removal of different heavy metal ions. For this purpose, they used the free radical cryopolymerization technique and then, the cryogels were modified using N-acetylcysteine. After preparing the cryogel, FTIR, SEM and EDX analyses were used for characterization studies. Removed amounts of heavy metal were demonstrated by stripping voltammetry and the heavy metal removal efficiency was found to be 90.74 for Cd(II). In addition, they showed that these cryogels were successfully used for the removal of heavy metals from environmental and biological media (Evli et al., 2020).
Removal of Ni
Nickel (Ni) is essential metal element and is defined as a component in a number of enzymes, participating in important metabolic reactions. However, Ni(II) ion intake over the permissible levels results in different types of disease such as pulmonary fibrosis, renal edema, skin dermatitis, and gastrointestinal distress (e.g., nausea, vomiting, diarrhea). It is therefore, essential to remove Ni(II) from wastewater before disposal (Marti, 2012).
Ion-imprinted polymeric materials recently are used for prepared selective materials in the removal of heavy metals. Also, the different structure configuration of cryogels is presented as an effective alternative for this sensitive and selective removal due to its combination with ion-imprinted technology. In this aim, supermacroporous and high flexible cryogel columns were synthesized by Tamahkar et al., to remove Ni(II) ions. This study's remarkable combination of histidine based functional monomer and Ni(II) ions with the two different molar ratios optimized the adsorption capacity (Figure 3). The Ni(II)-MIP cryogels were prepared by cryo-polymerization of N-isopropyl acrylamide (NIPAm) and N-methacryloyl-l-histidine (MAH) was chosen as functional monomer. After synthesized the two types of ion-imprinted cryogel columns, the characterization studies were done with SEM, FT-IR and swelling tests. They reported 1.89 mg/g maximum adsorption capacity for type one cryogel column as named MIP1 and 5.54 mg/g for other (MIP2) cryogels column (Tamahkar et al., 2017).
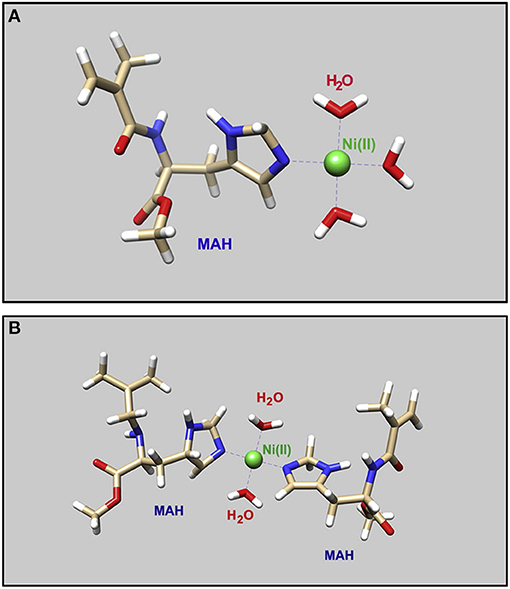
Figure 3. (A) Geometrical optimization of the molecular structure of (1:1) molar ratio for MAH-Ni(II) pre-complexes and (B) (2:1) molar ratio (Tamahkar et al., 2017).
Heavy metals ions are very harmful for environment if they are discharged into soils or natural water resources. It takes place in many applications in industry and therefore in our daily life. Papancea et al. prepared poly(vinyl alcohol) hydrogel membranes (PVA HG) for sorption and transport of metal ions (here Ni, Cu and Pb). For this purpose, physical crosslinking through the freezing/thawing method was used and was analyzed using aqueous nitrate solutions of copper, lead, and nickel, at wide concentrations ranging from 1 to 100 mM. The surface morphology and sorption effect of PVA HG were characterized by SEM, FTIR, swelling and loading degrees. They reported that analysis of adsoprtion and diffusion coefficients of 100 mM aqueous solutions of Ni2+, Cu2+, and Pb2+ was shown the diffusion process may be primarily defined by hydrodynamic models (Papancea et al., 2010). PVA membranes are synthesized by irradiation crosslinking or by PVA grafting with acrylic acid and styrene. PVA is a well-known biomaterial, and has also been used as a matrix for the removal of metal ions by biosorption or bioaccumulation.
Removal of Ag
The silver NPs (AgNPs) have unique antimicrobial features and they are one of the most broadly employed nanoparticles in a range of consumer goods including clothing, food packaging, medical tools, and cleaning agents. The common employ inevitably leads to AgNP release into the environment. AgNPs have potential adverse effects on human health and the environment (Sun et al., 2013). For this purpose, the Sarkaya et al., present a novel alternative method to prevent the problem of Silver releasing into the environment. Silver ions Ag(I) imprinted poly(hydroxyethyl methacrylate) (PHEMA)-based cryogels were prepared using N-methacryloyl-L-cysteine as functional monomer. They developed silver ions imprinted polymeric matrix and reported 49.27 mg/g maximum adsorption from an aqueous solution. They used photographic film to obtain the matrix's affinity as a natural Ag(I) ion source. Finally, the successful experiments were studied, and 72.8% recovery was demonstrated for Ag(I) imprinted matrix while this data was reported 0.62% for Ag(I) non-imprinted matrix. The reusability of the matrix was reported more than 10 times (Sarkaya et al., 2019). The mainly advantages of molecularly imprinted polymers (MIPs) are their high selectivity and affinity for the target molecule employed in the imprinting procedure (Akgönüllü et al., 2020a,c, 2021).
Removal of As
Arsenic (As) is a highly toxic metalloid and a natural environmental contaminant that humans are routinely exposed to in food, water, air, and soil (Hughes et al., 2011). Long-term arsenic-contaminated water exposure and ingestion can cause severe health effects, including neurological disorders, cancer, and skin lesions. The WHO designates a strict minimum limit of 0.01 mg/L in drinking water (Mandal and Suzuki, 2002; Mohan and Pittman, 2007). In aqueous systems, inorganic arsenic predominantly exists as oxyanions of arsenate (H3AsO4, +5 oxidation state) and arsenite (H3AsO3, +3 oxidation state). (Smedley and Kinniburgh, 2002; Türkmen et al., 2021). Arsenite (As(III)) ions are more mobile and toxic when compared to arsenate (As(V)) ions [119]. Several different methods for removing arsenic metalloids include ion exchange, adsorption, membrane filtration, and coagulation-flocculation. The adsorption method was used for removing arsenic due to the significant advantages such as high removal efficiency, simple process, and cost-effectiveness (Cheng et al., 2009).
The novel carbon cryogel/ceria composite for arsenic(III) adsorption process was developed by Arsic et al., They were performed adsorption kinetic, the effect of pH, and initial concentration of As(III) on removal. The rate was examined in a batch system. Carbon cryogel was synthesized by freeze-drying and carbonization in an inert atmosphere at 800°C. The characterization of carbon-cryogels by using a field emission (FE)-SEM showed that the homogeneous distribution of ceria on the surface of the carbon cryogel was achieved. The carbon cryogel/ceria and carbon cryogel specific surface area was calculated 614 m2/g and 620 m2/g, respectively. In this study, pH 5 was reported for the maximum adsorption capacity. Adsorption dose experiments on pure carbon-cryogel and carbon cryogel-ceria were carried out for the same amount of adsorbed arsenic(III) ions in the mass of 100 mg adsorbent. Compared with carbon-cryogel, the As(III) adsorption capacity of the carbon cryogel/ceria composite was increased by 20 times (Minovi et al., 2016). In another study, mesoporous carbon cryogel (CC) were prepared by Minovic. Resorcinol–formaldehyde (RF)-based cryogels were obtained using sol-gel polycondensation, and freeze-drying was used by t-butanol. The carbon cryogel adsorbent was used for the removal of As(III). They have investigated the adsorption of As(III) on carbon cryogel as a function of As(III) concentration and solution pH (pH range 2–11) (Gulicovski et al., 2015b).
Sahiner et al., developed poly[(3-Acrylamidopropyl)trimethylammonium chloride] [p(APTMACl)] cryogels for removing arsenate anions from an aqueous sample. It showed rapid swelling in water in about 7 secs. The maximum arsenate adsorption capacity was obtained about 120 mg/g for cryogels. According to the Langmuir and Freundlich adsorption isotherm models applied to adsorption arsenate anions by polymeric cryogels, the arsenate adsorption conformed to the Langmuir adsorption isotherm models (Sahiner et al., 2015).
A cryogels incorporated iron-aluminum double hydrous oxides (Fe-Al-Cryo) were synthesized by Kumar. These composite based cryogels were used for the adsorption of arsenic (III). Here, the Langmuir isotherm model was showed the best fit for As(III) adsorption. In addition, 24.6 mg/g maximum adsorption capacity was demonstrated. This study aimed to reduce the As(III) concentration in wastewater below 0.15 mg/L by synthesized cryogel without using any pretreatment step (Kumar et al., 2013).
Otero-gonzález et al. (2020) developed a novel nanostructured iron oxide macroporous cryogels for removing As(III). In the synthesis method, regardless of the nature of the copolymer forming the cryogel structure, iron oxide was allowed to disperse homogeneously in the cryogel reaction mixture. Here, monomer (AAm or HEMA) and crosslinker (MBAA or PEGDA) were used to prepare monolithic macroporous hybrid cryogels using co-polymerising under cryogenic conditions. Therefore, two different cryogels were synthesized by using AAm monomer and MBAA crosslinker and the other hand HEMA monomer using PEGDA crosslinker. An excellent mechanical property was reported for the acrylamide-based cryogel with 118 mg/g maximum adsorption capacity for As(III) and best fit with the Langmuir model.
Kurozumi et al. (2015) prepared polyacrylamide cryogels for removal of As(V). They synthesized the procedure by freezing monomer solution of acrylamide with cross-linker N, N-methylene bis(acrylamide) and polymerizing initiated by N,N,N′,N′-tetramethyl ethylenediamine, and ammonium peroxodisulfate at sub-zero temperatures. Then, Iron hydroxide oxide particles were embedded on the surface of the cryogel wall. The polyacrylamide cryogel adsorbent containing iron hydroxide oxide particles adsorbed many As(V) at low and high concentrations successfully. The synthesis procedure of polyacrylamide cryogels was demonstrated in Figure 4.
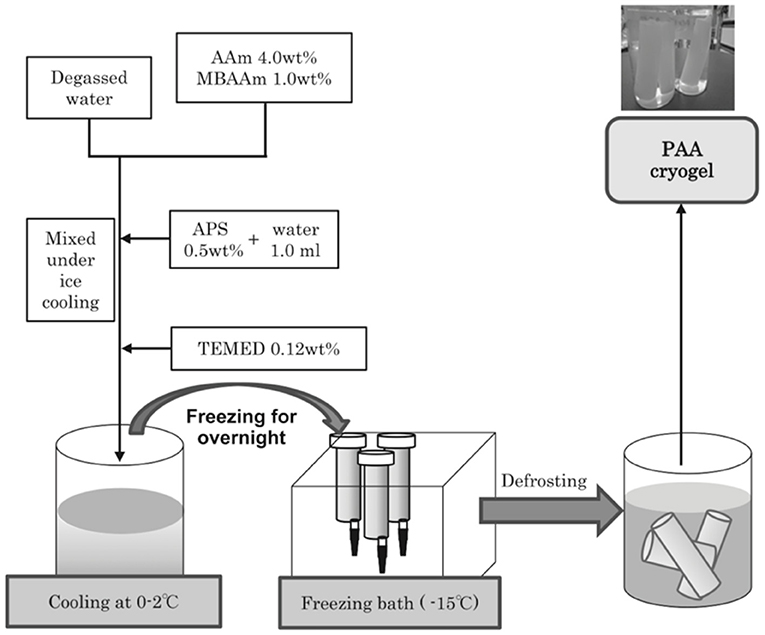
Figure 4. Preparation of the polyacrylamide cryogel: polymerization system; a program controlled refrigerated ethanol bath and at −15°C for 24 h (Kurozumi et al., 2015).
Purohit et al., developed a cost-effective and water-stable transition metal oxide doped cross-linked chitosan cryogel for highly sensitive removal of arsenic ions from potable water. The synthesized CuO–ZnO doped cross-linked chitosan cryogels were characterized by SEM, EDAX, XRD and FTIR. They reported that the adsorption kinetics fit the Freundlich isotherm model. The arsenic contamination was reduced to 76% after 2 h of contact with only 10 mg of adsorbent. They have cited it as a potential candidate for low-cost arsenic removal (Purohit et al., 2020).
The common goal of the studies given above is the social and economic importance of developing easy and cost-effective systems for the contamination of arsenic metalloids, which is a global concern.
Removal of Pb
Lead (Pb) has a low melting point, is soft and straightforward to operate, and is utilized in various applications, including pharmaceuticals, paint pigments, mascara, and batteries (Yari et al., 2015). Pb is also toxic in a trace amount and strongly affects ecosystems and the human body through various routes. Pb was taken up in the body through oral administration, inhalation, and other methods, is not easily excreted and accumulate in the body. This accumulated Pb can cause blood disorders, anorexia, gastrointestinal symptoms such as constipation, problems with the nervous system and kidney malfunction (Huang et al., 2011). Therefore, it is essential to remove Pb from water and wastewater.
Kim and Lee (2019b) developed a cryogel to absorb lead (Pb) ions from an aqueous solution. The poly(allylamine-co-methacrylamide-co-acrylic acid) [p(AA-co-MA-co-AAc)] cryogel and later functionalized with thiourea using methyl isothiocyanate. It contains amine groups and carboxyl groups. The functionalized p(AA-co-MA-co-AAc) cryogel exhibited the highest adsorption amount at pH 7 and 400 mg/L. Equilibrium isotherm data have been reported to fit the Langmuir isotherm better. The results show that the functionalized cryogel surfaces are uniform, and monolayer adsorption occurs. A new adsorbent for removing Pb ions from wastewater was developed by Park and Min (2012). They synthesized poly(vinyl alcohol)/hydroxyapatite (PVA/HAp) composite cryogel using water and freeze-thawing process. The maximum adsorption capacity of the HAp-based cryogels and PVP-based cryogel was 69 and 108 mg/g, respectively.
In another study, Kim et al., prepared a poly(allylamine-co-methacrylamide-co-acrylic acid) [p(AA-co-MA-co-AAc)] cryogel containing amine and carboxyl groups and then subsequently functionalized with thiourea using methyl isothiocyanate (Kim and Lee, 2019a). The functionalized p(AA-co-MA-co-AAc) cryogel was applied to the adsorption of Pb ions from an aqueous solution. They reported the amount of Pb adsorption. The functionalized p(AA-co-MA-co-AAc) cryogel was analyzed by FTIR and SEM. Also, they characterized pore shape, pore distribution, and specific surface area of the materials. They studied the effect of the solution pH and initial concentration on Pb adsorption. The functionalized p(AA-co-MA-co-AAc) cryogel exhibited the highest adsorption amount at pH 7 and 400 mg/L.
The great attention of 3D cryogels with interconnected macropore structures is increased owing to their immense application potential in wastewater cleanup. Zhong et al. (2021) fabricated a pure poly(vinyl imidazole) cryogel through an improved cryogelation method, adding the pre-freezing–thawing step, which reduced the temperature difference between the different positions of the precursor solution during the reaction. They reported that the prepared 3D cryogel featured a robust underwater elastic performance. The adsorption capacity of Ni(II), Cu(II), Zn(II), Pb(II), Co(II), and Cd(II) ions from aqueous solutions by cryogel was explored. They reported the specific adsorption of Cu(II), in which the adsorption capacity and removal efficiency reached 148 mg/g and 99.99%, respectively.
Another wastewater clean-up study was reported by Zhang et al. (2021) they developed a strategy to fabricate the poly(VIM) cryogel using micro fibrillated cellulose (MFC) as a reinforcing agent. Structural characterizations indicated that the poly(VIM)/MFC showed interconnected macropores of 12 ± 10 μm and high VIM contents. They reported batch adsorption with adsorption capacities of the poly(VIM)/MFC cryogel to Cu(II), Pb(II), Zn(II), Cd(II), Ni(II), and Co(II) were 87.4, 53.9, 48.6, 44.3, 23.8, and 20.1 mg/g, respectively. Dynamic adsorption displayed that the loaded Cu(II) ions could be rapidly adsorbed and hardly flow through the poly(VIM)/MFC cryogel, indicating the extremely fast adsorption at the flow mode.
Removal of Hg
Mercury (Hg) is one of the most toxic ion elements (Fu et al., 2019). Hg is highly poisonous even at trace levels for living organisms. It is frequently discharged into the aquatic environment through industrial wastewaters, including those produced by the fertilizer, mining, pharmaceutical industries, and electroplating and metal processing (Arshadi et al., 2018). Zhu et al. prepared SiO2 cryogel through the sol-gel method and freeze-drying process. The SiO2 cryogels were functionalized with sulfhydryl groups. The commercial water glass was chosen as the silica source to prepare silica cryogel, and 3-Mercaptopropyl-Trimethoxysilane (MPTMS) was used as the modifier. They reported that has been successfully synthesized high specific surface area silica cryogel. Four heavy metal ions including Cd(II), Zn(II), Cu(II), and Pb(II) were used to investigate the specific effect on the removal of Hg(II) by the SiO2-SH cryogel. The removal rate of Hg always remained the highest compared to other ions. The theoretical maximum removal capacity from the Langmuir isotherm model was calculated to be 709.2 mg/g. They reported that the removal rate of Hg(II) was decreased from 99.96 to 81.95% after five adsorption/desorption cycles (Zhu et al., 2021).
Removal of Cu
Copper (Cu) as a heavy metal element is hazardous to human health and the environment. It is broadly employed in industry and is accumulating in their waste streams (Al-Harahsheh et al., 2015). Tang et al. (2020) developed cellulose nanofibril (CNF) cryogel beads for Cu(II) removal. The cellulose nanomaterial has been broadly employed in various fields such as wastewater treatment, energy storage, oil recovery, biomedical, agriculture to food additives. The highly carboxylated and chemically crosslinked CNF cryogel beads were synthesized by a one-step ester cross-linking protocol. The maleic anhydride (MA) was used as the crosslinker. The presence of carboxyl groups and ester linkages were characterized by FTIR and conductometric-potentiometric titration. The carboxylic was content of up to 2.78 mmol/g. They have also investigated the effect of CNF concentration on the morphology and wet mechanical strength of the crosslinked cryogel beads. The pH of 5.6 was selected as the optimum pH for conducting the adsorption studies. The maximum removal capacity of Cu (II) was found to be 82.17 mg/g. The results fitted the data better by the pseudo-second-order model. The maximum removal capacities of Cu(II) was determined from the Langmuir model were 60.92 mg/g and 84.12 mg/g for CNF-MA 1% and CNF-MA 2%, respectively. They reported that cryogel beads are robust and efficient to remove Cu(II) ions. In addition, the regeneration of beads was shown perfectly. As a result, the designed CNF-MA cryogel beads are promising adsorbents for heavy metal ions removal because of their quick removal rate, robust mechanical strength and, perfect reusability. Furthermore, catalyst and organic solvent were not needed.
Common cryogel and non- cryogel materials used in heavy metal removal are tabulated in Table 3.
The cryogels presented in this review displayed good performance with high removal capacity, quick absorption rates, and high selectivity, as well as pH insensitivity. Thus, they have considerable potential as an absorbent for the removal of heavy metal ions in wastewater containing multiple compounds.
Conclusions
The materials based on cryogels are novel and talented adsorbents. Cryogels consist of a three-dimensional flexible polymeric matrix prepared using freeze-thaw techniques. Cryogels are macroporous cross-linked matrices and have many advantages due to their chemical and structural stability. The cryogel is a cryogenically structured polymeric matrix with applications in various fields, including environment, medicine, food technology, cell/ tissue engineering, etc. The chemical and physical properties of cryogels, including elasticity, ease of chemical modification, macroporosity, and water permeability have been the subject of strong research in various fields such as catalysis, purification, biotechnology, bioremediation, biosensors, and regenerative medicine. Cryogels showed perfect removal performance and high selectivity for nutrients, heavy metal ions, and toxic dyes from an aqueous medium. With the quick growth of urbanization, heavy metal ions (such as copper (II), lead (II), silver(I), cadmium(II), arsenic(III), nickel(II), and mercury(II)) produced from mining, machinery manufacturing, and chemical engineering processes have been discharged, causing serious water pollution worldwide. The pollution of surface and groundwater with heavy metal elements is a severe global concern, both environmentally, as well as concerning human health and also for other life forms through bioaccumulation along food chains. Therefore, steps should be taken to reduce the number of such elements in water to acceptable levels. In the presented works, a maximum adsorption capacity of over 90% has been obtained for heavy metal ions with the developed cryogels. The general cryogelation procedure conditions of all works have been achieved at the −12 and −18°C range for 12 to 24 h. Moreover, the thawing has been performed at room temperature along with washing with deionized water in the last step for this procedure. This review provides an overview of the recent macroporous cryogels to remove heavy metal ions from water waste. This paper also discusses the cryogels synthesis techniques, characterization process, and applications to remove heavy metal ions from aqueous solutions. Compared to traditional adsorbents, cryogel polymers exhibit some advantages, including permeability and fast kinetics, which can be significant in many applications. Cryogels and their functionalized forms seem to have an excellent adsorption capacity of up to hundreds of mg/g for various heavy metal ions. Although cryogels show developed adsorption capability, they still face restrictions for large scale applications, such as low mechanical strength under certain conditions. As a result, the preparation of new cryogel composites that can efficiently remove heavy metal ions from aqueous environments and their use in widely application fields are essential.
Author Contributions
DT, MB, SAk, SAş, and AD contributed to the conception, design of the study, and wrote sections of the manuscript. All authors contributed to manuscript revision, read, and approved the submitted version.
Conflict of Interest
The authors declare that the research was conducted in the absence of any commercial or financial relationships that could be construed as a potential conflictof interest.
Publisher's Note
All claims expressed in this article are solely those of the authors and do not necessarily represent those of their affiliated organizations, or those of the publisher, the editors and the reviewers. Any product that may be evaluated in this article, or claim that may be made by its manufacturer, is not guaranteed or endorsed by the publisher.
References
Ak, F., Oztoprak, Z., Karakutuk, I., and Okay, O. (2013). Macroporous silk fibroin cryogels. Biomacromolecules 14, 719–727. doi: 10.1021/bm3018033
Akgönüllü, S., Battal, D., Yalcin, M. S., Yavuz, H., and Denizli, A. (2020c). Rapid and sensitive detection of synthetic cannabinoids JWH-018, JWH-073 and their metabolites using molecularly imprinted polymer-coated QCM nanosensor in artificial saliva. Microchem. J. 153, 104454. doi: 10.1016/j.microc.2019.104454
Akgönüllü, S., Yavuz, H., and Denizli, A. (2017). Preparation of imprinted cryogel cartridge for chiral separation of l-phenylalanine. Artif. Cells, Nanomedicine Biotechnol. 45, 800–807. doi: 10.1080/21691401.2016.1175445
Akgönüllü, S., Yavuz, H., and Denizli, A. (2020a). SPR nanosensor based on molecularly imprinted polymer film with gold nanoparticles for sensitive detection of aflatoxin B1. Talanta 219, 121219. doi: 10.1016/j.talanta.2020.121219
Akgönüllü, S., Yavuz, H., and Denizli, A. (2021). Development of gold nanoparticles decorated molecularly imprinted—based plasmonic sensor for the detection of aflatoxin M1 in milk samples. Chemosensors 9, 363. doi: 10.3390/chemosensors9120363
Akgönüllü, S., Bakhshpour, M., Idil, N., Andaç, M., Yavuz, H., and Denizli, A. (2020b). Versatile polymeric cryogels and their biomedical applications. Hacettepe J. Biol. Chem. 48, 99–118. doi: 10.15671/hjbc.629355
Akilbekova, D., Shaimerdenova, M., Adilov, S., and Berillo, D. (2018). Biocompatible scaffolds based on natural polymers for regenerative medicine. Int. J. Biol. Macromol. 114, 324–333. doi: 10.1016/j.ijbiomac.2018.03.116
Al-Harahsheh, M. S., Zboon, K., Al, Al-Makhadmeh, L., Hararah, M., and Mahasneh, M. (2015). Fly ash based geopolymer for heavy metal removal: A case study on copper removal. J. Environ. Chem. Eng. 3, 1669–1677. doi: 10.1016/j.jece.2015.06.005
Alizadeh, B., Ghorbani, M., and Salehi, M. A. (2016). Application of polyrhodanine modified multi-walled carbon nanotubes for high efficiency removal of Pb (II) from aqueous solution. J. Mol. Liq. 220, 142–149. doi: 10.1016/j.molliq.2016.04.065
Alkan, H. (2015). Poly(hydroxyethyl methacrylate)-co-N-methacryloyl-(L)- histidine methyl ester Based Cryogel for the Removal of Fe3+ from Human Plasma effected with Beta Thalassemia. Hacettepe J. Biol. Chem. 43, 179–185. doi: 10.15671/HJBC.20154314240
Alyüz, B., and Veli, S. (2009). Kinetics and equilibrium studies for the removal of nickel and zinc from aqueous solutions by ion exchange resins. J. Hazard. Mater. 167, 482–488. doi: 10.1016/j.jhazmat.2009.01.006
Andaç, M., Baydemir, G., Yavuz, H., and Denizli, A. (2012). Molecularly imprinted composite cryogel for albumin depletion from human serum. J. Mol. Recognit. 25, 555–563. doi: 10.1002/jmr.2202
Andrabi, S. M., Tiwari, J., Singh, S., Sarkar, J., and Kumar, A. (2016). International Journal of Polymeric Materials and Supermacroporous hybrid polymeric cryogels for efficient removal of metallic contaminants and microbes from water. Int. J. Polym. Mater. Polym. Biomater. ISSN 65, 636–645. doi: 10.1080/00914037.2016.1157795
Ari, B., and Sahiner, N. (2020). Biodegradable super porous inulin cryogels as potential drug carrier. Polym. Adv. Technol. 20, 1–11. doi: 10.1002/pat.5014
Arshadi, M., Eskandarloo, H., Abdolmaleki, M. K., and Abbaspourrad, A. (2018). A biocompatible nanodendrimer for efficient adsorption and reduction of Hg(II). ACS Sustain. Chem. 6, 13332–13348. doi: 10.1021/acssuschemeng.8b02965
Aşir, S., Uzun, L., Türkmen, D., Say, R., and Denizli, A. (2005). Ion-selective imprinted superporous monolith for cadmium removal from human plasma ion-selective imprinted superporous. Sep. Sci. Technol. 40, 3167–3165. doi: 10.1080/01496390500385376
Bäcker, A., Göppert, B., Sturm, S., Abaffy, P., Sollich, T., and Gruhl, F. J. (2016). Impact of adjustable cryogel properties on the performance of prostate cancer cells in 3D. Springerplus 5, 902. doi: 10.1186/s40064-016-2629-z
Bagri, L. P., Bajpai, J., and Bajpai, A. K. (2011). Evaluation of starch based cryogels as potential biomaterials for. Bull. Mater. Sci. 34, 1739–1748. doi: 10.1007/s12034-011-0385-9
Baimenov, A., Berillo, D., Abylgazina, L., Poulopoulos, S. G., and Inglezakis, V. J. (2018). Novel amphoteric cryogels for Cd2+ ions removal from aqueous solutions. Key Eng. Mater. 775, 376–382. doi: 10.4028/www.scientific.net/KEM.775.376
Baimenov, A., Berillo, D., Azat, S., Nurgozhin, T., and Inglezakis, V. (2020b). Removal of Cd2+ from water by use of super-macroporous cryogels and comparison to commercial adsorbents. Polymers (Basel). 12, 2405. doi: 10.3390/polym12102405
Baimenov, A., Berillo, D. A., Poulopoulos, S. G., and Inglezakis, V. J. (2020a). A review of cryogels synthesis, characterization and applications on the removal of heavy metals from aqueous solutions. Adv. Colloid Interface Sci. 276, 102088. doi: 10.1016/j.cis.2019.102088
Bakhshpour, M., and Denizli, A. (2020). Highly sensitive detection of Cd(II) ions using ion-imprinted surface plasmon resonance sensors. Microchem. J. 159, 105572. doi: 10.1016/j.microc.2020.105572
Bakhshpour, M., Idil, N., Perçin, I., and Denizli, A. (2019). Biomedical applications of polymeric cryogels. Appl. Sci. 19, 553. doi: 10.3390/app9030553
Bakhshpour, M., Topcu, A. A., Bereli, N., Alkan, H., and Denizli, A. (2020). Poly(Hydroxyethyl methacrylate) immunoaffinity cryogel column for the purification of human immunoglobulin M. Gels 6, 4. doi: 10.3390/gels6010004
Bakhshpour, M., Yavuz, H., and Denizli, A. (2018). Controlled release of mitomycin C from PHEMAH–Cu(II) cryogel membranes. Artif. Cells, Nanomedicine Biotechnol. 18, 840. doi: 10.1080/21691401.2018.1439840
Barbetta, A., Gumiero, A., Pecci, R., and Bedini, R. (2009). Gas-in-liquid foam templating as a method for the production of highly porous scaffolds. Biomacromolecules 10, 3188–3192. doi: 10.1021/bm901051c
Baydemir, G., Türkoglu, E. A., Andaç, M., Perçin, I., and Denizli, A. (2014). Composite cryogels for lysozyme purification. Biotechnol. Appl. Biochem. 62, 200–207. doi: 10.1002/bab.1259
Bencherif, S. A., Sands, R. W., Bhatta, D., Arany, P., Verbeke, C. S., and Edwards, D. A. (2012). Injectable preformed scaffolds with shape-memory properties. Proc Natl Acad Sci U S A 109, 19590–19595. doi: 10.1073/pnas.1211516109
Bereli, N., Saylan, Y., Uzun, L., and Denizli, A. (2011). L-Histidine imprinted supermacroporous cryogels for protein recognition. Sep. Purif. Technol. 82, 28–35. doi: 10.1016/j.seppur.2011.08.011
Bereli, N., Türkmen, D., and Denizli, A. (2012). Glutamic acid containing supermacroporous poly (hydroxyethyl methacrylate) cryogel disks for UO22+ removal. Mater. Sci. Eng. C 32, 2052–2059. doi: 10.1016/j.msec.2012.05.015
Berillo, D., Mattiasson, B., and Kirsebom, H. (2014). Cryogelation of chitosan using noble-metal ions: in situ formation of nanoparticles. Biomacromolecules 15, 2246–2255. doi: 10.1021/bm5003834
Bibi, N. S., Singh, N. K., Dsouza, R. N., Aasim, M., and Fernández-lahore, M. (2013). Synthesis and performance of megaporous immobilized metal-ion affinity cryogels for recombinant protein capture and purification. J. Chromatogr. A 1272, 145–149. doi: 10.1016/j.chroma.2012.11.036
Biswas, D. P., Tran, P. A., Tallon, C., and Connor, A. J. O. (2016). Combining mechanical foaming and thermally induced phase separation to generate chitosan scaffolds for soft tissue engineering. J. Biomater. Sci. Polym. Ed. 28, 207–226. doi: 10.1080/09205063.2016.1262164
Briffa, J., Sinagra, E., and Blundell, R. (2020). Heavy metal pollution in the environment and their toxicological effects on humans. Heliyon 6, e04691. doi: 10.1016/j.heliyon.2020.e04691
Brougham, C. M., Levingstone, T. J., Shen, N., Cooney, G. M., Jockenhoevel, S., Flanagan, T. C., et al. (2017). Freeze-drying as a novel biofabrication method for achieving a controlled microarchitecture within large, complex natural biomaterial scaffolds. Adv. Healthc. Mater. 5, 1700598. doi: 10.1002/adhm.201700598
Burakov, A. E., Galunin, E. V., Burakova, I. V., Kucherova, A. E., Agarwal, S., Tkachev, A. G., et al. (2018). Adsorption of heavy metals on conventional and nanostructured materials for wastewater treatment purposes : a review. Ecotoxicol. Environ. Saf. 148, 702–712. doi: 10.1016/j.ecoenv.2017.11.034
Caló, E., Barros, J. M. S., de, Fernández-Gutiérrez, M., Román, J. S., Ballamy, L., and Khutoryanskiy, V. V. (2016). Antimicrobial hydrogels based on autoclaved poly(vinyl alcohol) and poly(methyl vinyl ether-alt-maleic anhydride) mixtures for wound care applications. RSC Adv. 6, 55211–55219. doi: 10.1039/C6RA08234C
Ceylan, S., Göktürk, D., and Bölgen, N. (2016). Effect of crosslinking methods on the structure and biocompatibility of polyvinyl alcohol / gelatin cryogels. Biomed. Mater. Eng. 27, 327–340. doi: 10.3233/BME-161589
Cheng, H., Hu, Y., Luo, J., Xu, B., and Zhao, J. (2009). Geochemical processes controlling fate and transport of arsenic in acid mine drainage (AMD) and natural systems. J. Hazard. Mater. 165, 13–26. doi: 10.1016/j.jhazmat.2008.10.070
Ciolacu, D., Rudaz, C., Vasilescu, M., and Budtova, T. (2016). Physically and chemically cross-linked cellulose cryogels: structure, properties and application for controlled release. Carbohydr. Polym. 151, 392–400. doi: 10.1016/j.carbpol.2016.05.084
Conoscenti, G., Schneider, T., Stoelzel, K., Pavia, F. C., Brucato, V., and Goegele, C. (2017). PLLA scaffolds produced by thermally induced phase separation allow human chondrocyte growth and extracellular matrix formation dependent on pore size. Mater. Sci. Eng. C 80, 449–459. doi: 10.1016/j.msec.2017.06.011
Dainiak, M. B., Allan, I. U., Savina, I. N., Cornelio, L., James, E. S., James, S. L., et al. (2010). Biomaterials Gelatin—ibrinogen cryogel dermal matrices for wound repair : Preparation, optimisation and in vitro study. Biomaterials 31, 67–76. doi: 10.1016/j.biomaterials.2009.09.029
Demir, D., and Bölgen, N. (2017). International Journal of Polymeric Materials and Synthesis and characterization of injectable chitosan cryogel microsphere scaffolds. Int. J. Polym. Mater. Polym. Biomater. 66, 686–696. doi: 10.1080/00914037.2016.1255614
Diken Gür, S., Bakhshpour, M., Bereli, N., and Denizli, A. (2021). Antibacterial effect against both Gram-positive and Gram-negative bacteria via lysozyme imprinted cryogel membranes. J. Biomater. Sci. Polym. Ed. 0, 1–16. doi: 10.1080/09205063.2021.1892472
Dragan, E. S., Humelnicu, D., Dinu, M. V., and Olariu, R. I. (2017). Kinetics, equilibrium modeling, and thermodynamics on removal of Cr(VI) ions from aqueous solution using novel composites with strong base anion exchanger microspheres embedded into chitosan/poly(vinyl amine) cryogels. Chem. Eng. J. 330, 675–691. doi: 10.1016/j.cej.2017.08.004
Dubey, R., Bajpai, J., and Bajpai, A. K. (2015). Journal of Water Process Engineering Green synthesis of graphene sand composite (GSC) as novel adsorbent for efficient removal of Cr (VI) ions from aqueous solution. J. Water Process Eng. 5, 83–94. doi: 10.1016/j.jwpe.2015.01.004
Duffus, J. H. (2002). “Heavy Metals ”— A Meaningless Term? . Pure Appl. Chem. 74, 793–807. doi: 10.1351/pac200274050793
Efremenko, E., Votchitseva, Y., Plieva, F., Galaev, I., and Mattiasson, B. (2006). Purification of His 6—organophosphate hydrolase using monolithic supermacroporous polyacrylamide cryogels developed for immobilized metal affinity chromatography. Appl. Microbiol. Biotechnol. 70, 558–563. doi: 10.1007/s00253-005-0103-x
Erdem, A., Ngwabebhoh, F. A., Çetintaş, S., Bingöl, D., and Yildiz, U. (2017). Fabrication and characterization of novel macroporous Jeffamine/diamino hexane cryogels for enhanced Cu (II) metal uptake: Optimization, isotherms, kinetics and thermodynamic studies. Chem. Eng. Res. Des. 117, 122–138. doi: 10.1016/j.cherd.2016.10.010
Ertürk, G., and Mattiasson, B. (2014). Cryogels-versatile tools in bioseparation. J. Chromatogr. A 1357, 24–35. doi: 10.1016/j.chroma.2014.05.055
Erzengin, M., Ünlü, N., and Odabas, M. (2011). A novel adsorbent for protein chromatography : Supermacroporous monolithic cryogel embedded with Cu2+ -attached sporopollenin particles. J. Chromatogr. A 1218, 484–490. doi: 10.1016/j.chroma.2010.11.074
Evli, S., Karagözler, A. A., Güven, G., Orhan, H., Uygun, M., and Aktaş Uygun, D. (2020). Heavy metal removal by N-acetylcysteine-functionalized cryogels. Bull. Mater. Sci. 43, 107. doi: 10.1007/s12034-020-2060-5
Fu, F., and Wang, Q. (2011). Removal of heavy metal ions from wastewaters : a review. J. Environ. Manage. 92, 407–418. doi: 10.1016/j.jenvman.2010.11.011
Fu, L., Wang, S., Lin, G., Zhang, L., Liu, Q., Fang, J., et al. (2019). Post-functionalization of UiO-66-NH2 by 2,5-Dimercapto-1,3,4-thiadiazole for the high efficient removal of Hg(II) in water. J. Hazard. Mater. 368, 42–51. doi: 10.1016/j.jhazmat.2019.01.025
Fukasawa, T., Ando, M., Ohji, T., and Kanzaki, S. (2001). Synthesis of porous ceramics with complex pore structure by freeze-dry processing. J. Am. Ceram. Soc. 84, 230–232. doi: 10.1111/j.1151-2916.2001.tb00638.x
Galaev, Y., Mattiasson, B., Plieva, F. M., and Ekstro, P. (2008). Monolithic cryogels with open porous structure and unique double-continuous macroporous networks. Soft Matter 4, 2418–2428. doi: 10.1039/b804105a
Göktürk, I., Derazshamshir, A., Yilmaz, F., and Denizli, A. (2016). Poly (vinyl alcohol)/ polyethyleneimine (PVA / PEI) blended monolithic cryogel columns for the depletion of haemoglobin from human blood. Sep. Sci. Technol. 51, 1787–1797. doi: 10.1080/01496395.2016.1183677
Gulicovski, J. J., Stoiljkovic, M. M., Jokic, B. M., Minovic, T. Z., Matovic, B. Z., and Babic, B. M. (2015a). Microporous and Mesoporous Materials Surface characterization of mesoporous carbon cryogel and its application in arsenic (III) adsorption from aqueous solutions. Microporous Mesoporous Mater. 201, 271–276. doi: 10.1016/j.micromeso.2014.09.031
Gulicovski, J. J., Stoiljkovic, M. M., Jokic, B. M., Minovic, T. Z., Matovic, B. Z., and Babic, B. M. (2015b). Surface characterization of mesoporous carbon cryogel and its application in arsenic (III) adsorption from aqueous solutions. Microporous Mesoporous Mater. 201, 271–276. doi: 10.1016/j.micromeso.2014.09.03
Gun, V. M., Savina, I. N., and Mikhalovsky, S. V. (2013). Cryogels : morphological, structural and adsorption characterisation. Adv. Colloid Interface Sci. 187, 1–46. doi: 10.1016/j.cis.2012.11.001
Hasanpour, M., and Hatami, M. (2020). Application of three dimensional porous aerogels as adsorbent for removal of heavy metal ions from water/wastewater: a review study. Adv. Colloid Interface Sci. 284, 102247. doi: 10.1016/j.cis.2020.102247
Hayati, B., Maleki, A., Naja, F., Gharibi, F., Mckay, G., Kumar, V., et al. (2018). Heavy metal adsorption using PAMAM/CNT nanocomposite from aqueous solution in batch and continuous fixed bed systems. Chem. Eng. J. 346, 258–270. doi: 10.1016/j.cej.2018.03.172
Hedström, M., Plieva, F., Galaev, I. Y., and Mattiasson, B. (2008). Monolithic macroporous albumin / chitosan cryogel structure : a new matrix for enzyme immobilization. Anal. Bioanal. Chem. 390, 907–912. doi: 10.1007/s00216-007-1745-6
Henderson, T. M. A., Ladewig, K., Haylock, D. N., Mclean, K. M., and Connor, A. J. O. (2013). Cryogels for biomedical applications. J. Mater. Chem. B 1, 2682–2695. doi: 10.1039/c3tb20280a
Hiew, B. Y. Z., Lee, L. Y., Lee, X. J., Thangalazhy-Gopakumar, S., Gan, S., Lim, S. S., et al. (2018). Review on synthesis of 3D graphene-based configurations and their adsorption performance for hazardous water pollutants. Process Saf. Environ. Prot. 116, 262–286. doi: 10.1016/j.psep.2018.02.010
Huang, Z., Zheng, X., Lv, W., Wang, M., Yang, Q., and Kang, F. (2011). Adsorption of Lead (II) ions from aqueous solution on low-temperature exfoliated graphene nanosheets. Langmuir 27, 7558–7562. doi: 10.1021/la200606r
Hube, S., Eska, M., Hrafnkelsdóttir, K. F., Bjarnadóttir, B., Bjarnadóttir, M. Á., Axelsdóttir, S., et al. (2020). Direct membrane fi ltration for wastewater treatment and resource recovery : a review. Sci. Total Environ. 710, 136375. doi: 10.1016/j.scitotenv.2019.136375
Hughes, M. F., Beck, B. D., Chen, Y., Lewis, A. S., and Thomas, D. J. (2011). Arsenic exposure and toxicology : a historical perspective. Toxicol. Sci. 123, 305–332. doi: 10.1093/toxsci/kfr184
Huisman, J. L., Schouten, G., and Schultz, C. (2006). Biologically produced sulphide for purification of process streams, effluent treatment and recovery of metals in the metal and mining industry. Hyrdometallurgy 83, 106–113. doi: 10.1016/j.hydromet.2006.03.017
Huseynli, S., Bakhshpour, M., Qureshi, T., Andac, M., and Denizli, A. (2020). Composite polymeric cryogel cartridges for selective removal of cadmium ions from aqueous solutions. Polymers (Basel). 12, 1–15. doi: 10.3390/polym12051149
Ingavle, G. C., Baillie, L. W. J., Zheng, Y., Lis, E. K., Savina, I. N., Howell, C. A., et al. (2015). Biomaterials affinity binding of antibodies to supermacroporous cryogel adsorbents with immobilized protein A for removal of anthrax toxin protective antigen. Biomaterials 50, 140–153. doi: 10.1016/j.biomaterials.2015.01.039
Jain, E., and Kumar, A. (2009). Designing supermacroporous cryogels based on polyacrylonitrile and a polyacrylamide—chitosan semi- interpenetrating network. J. Biomater. Sci. Polym. Ed. 20, 877–902. doi: 10.1163/156856209X444321
Jeong, J., Shin, G., Park, S., Il, Y.u, K. J., Xu, L., and Rogers, J. A. (2015). Soft materials in neuroengineering for hard problems in neuroscience. Neuron Rev. 86, 175–186. doi: 10.1016/j.neuron.2014.12.035
Ji, C., Annabi, N., Khademhosseini, A., and Dehghani, F. (2011). Fabrication of porous chitosan scaffolds for soft tissue engineering using dense gas CO2. Acta Biomater. 7, 1653–1664. doi: 10.1016/j.actbio.2010.11.043
Kanamori, K., Nakanishi, K., and Hanada, T. (2007). New macroporous crosslinked polymer gels prepared via living radical polymerization. Mater. Res. Soc. 0947, A03-27. doi: 10.1557/PROC-0947-A03-27
Karami, H. (2013). Heavy metal removal from water by magnetite nanorods. Chem. Eng. J. 219, 209–216. doi: 10.1016/j.cej.2013.01.022
Khan, M., and Lo, I. M. C. (2016). A holistic review of hydrogel applications in the adsorptive removal of aqueous pollutants: Recent progress, challenges, and perspectives. Water Res. 106, 259–271. doi: 10.1016/j.watres.2016.10.008
Kim, M. Y., and Lee, T. G. (2019a). Removal of Pb(II) Ions from Aqueous Solutions using Functionalized Cryogels. Chemosphere 217. doi: 10.1016/j.chemosphere.2018.10.021
Kim, M. Y., and Lee, T. G. (2019b). Removal of Pb (II) ions from aqueous solutions using functionalized cryogels. Chemosphere 217, 423–429. doi: 10.1016/j.chemosphere.2018.10.02
Kirsebom, H., Topgaard, D., Galaev, I. Y., and Mattiasson, B. (2010). Modulating the porosity of cryogels by influencing the nonfrozen liquid phase through the addition of inert solutes. Langmuir 26, 16129–16133. doi: 10.1021/la102917c
Koç, I., Baydemir, G., Bayram, E., Yavuz, H., and Denizli, A. (2011). Selective removal of 17?–estradiol with molecularly imprinted particle-embedded cryogel systems. J. Hazard. Mater. J. 192, 1819–1826. doi: 10.1016/j.jhazmat.2011.07.017
Kostova, B., Momekova, D., Petrov, P., Momekov, G., Toncheva-moncheva, N., Tsvetanov, C. B., et al. (2011). Poly (ethoxytriethyleneglycol acrylate) cryogels as novel sustained drug release systems for oral application. Polymer (Guildf). 52, 1217–1222. doi: 10.1016/j.polymer.2011.01.049
Kumar, P. S., Önnby, L., and Kirsebom, H. (2013). Arsenite adsorption on cryogels embedded with iron-aluminium double hydrous oxides : possible polishing step for smelting wastewater? J. Hazard. Mater. 250, 469–476. doi: 10.1016/j.jhazmat.2013.02.022
Kurozumi, M., Yano, Y., Kiyoyama, S., Kumar, A., and Shiomori, K. (2015). Adsorption Properties of Arsenic (V) by Polyacrylamide Cryogel Containing Iron Hydroxide Oxide Particles Prepared by in situ Method. Resour. Process. 62, 17–23. doi: 10.4144/rpsj.62.17
Liu, X., Xu, X., Sun, J., Alsaedi, A., Hayat, T., Li, J., et al. (2018). Insight into the impact of interaction between attapulgite and graphene oxide on the adsorption of U (VI). Chem. Eng. J. 343, 217–224. doi: 10.1016/j.cej.2018.02.113
Lockwood, C. L., Mortimer, R. J. G., Stewart, D. I., Mayes, W. M., Peacock, C. L., Polya, D. A., et al. (2014). Applied Geochemistry Mobilisation of arsenic from bauxite residue (red mud) affected soils : effect of pH and redox conditions. Appl. Geochemistry 51, 268–277. doi: 10.1016/j.apgeochem.2014.10.009
Loo, S., Lim, T., Krantz, W. B., and Fane, A. G. (2014). Potential evaluation and perspectives on using sponge-like superabsorbent cryogels for onsite water treatment in emergencies. Desalin. Water Treat. 53, 1506–1515. doi: 10.1080/19443994.2014.943064
Lottermoser, A. (1908). Über das Ausfreren von. Hydrosolen. 41, 3976–3979. doi: 10.1002/cber.19080410398
Lozinsky, V. I. (2002). Cryogels on the basis of natural and synthetic polymers : preparation, properties and applications. Russ. Chem. Rev. 71, 489–511. doi: 10.1070/RC2002v071n06ABEH000720
Lozinsky, V. I., Faleev, N. G., Zubov, A. L., Ruvinov, S. B., Antonova, T. V., Vainerman, E. S., et al. (1989). Use of PVA-Cryogel entrapped citrobacter intermedius cells for continuous production of 3-Fluoro-L-Tyrosine. Biotechnol. Lett. 11, 43–48. doi: 10.1007/BF01026784
Lozinsky, V. I., and Okay, O. (2014). “Basic Principles of Cryotropic Gelation,” in Polymeric Cryogels, Advanced in Polymer Science, ed. O. Okay (Switzerland: Springer International Publishing Switzerland), 49–96.
Lozinsky, V. I., Vainerman, E. S., and Rogozhin, S. V. (1982). Study of cryostructurization of polymer systems. II. the influence of freezing of a reacting mass on the properties of products in the preparation of covalently cross-linked gels. Colloid Polym. Sci. 260, 776–780. doi: 10.1007/BF01452068
Mahler, W., and Bechtold, M. F. (1980). Freeze-formed silica fibers. Nature 285, 27–28. doi: 10.1038/285027a0
Malik, R., Dahiya, S., and Lata, S. (2017). An experimental and quantum chemical study of removal of utmostly quantified heavy metals in wastewater using coconut husk: a novel approach to mechanism. Int. J. Biol. Macromol. 98, 139–149. doi: 10.1016/j.ijbiomac.2017.01.100
Mandal, B. K., and Suzuki, K. T. (2002). Arsenic round the world : a review. Talanta 58, 201–235. doi: 10.1016/S0039-9140(02)00268-0
Manzoori, J. L., Abdolmohammad-zadeh, H., and Amjadi, M. (2007). Ultratrace determination of cadmium by cold vapor atomic absorption spectrometry after preconcentration with a simplified cloud point extraction methodology. Talanta 71, 582–587. doi: 10.1016/j.talanta.2006.04.036
Marti, M. A. (2012). Removal of nickel (II) ions from aqueous solutions by biosorption on sugarcane bagasse. J. Taiwan Inst. Chem. Eng. 43, 275–281. doi: 10.1016/j.jtice.2011.10.011
Memic, A., Colombani, T., Eggermont, L. J., Rezaeeyazdi, M., Steingold, J., Rogers, Z. J., et al. (2019). Latest advances in cryogel technology for biomedical applications. Adv. Ther. 1800114, 1–45. doi: 10.1002/adtp.201800114
Minovi, T., Kalijadis, A., Matovi, B., Stoiljkovi, M., Panti, J., Jovanovi, J., et al. (2016). Arsenic (III) adsorption from aqueous solutions on novel carbon cryogel/ceria nanocomposite. Process. Appl. Ceram. 10, 17–23. doi: 10.2298/PAC1601017M
Mohan, D., and Pittman, C. U. (2007). Arsenic removal from water/wastewater using adsorbents—a critical review. J. Hazard. Mater. 142, 1–53. doi: 10.1016/j.jhazmat.2007.01.006
Newland, B., Welzel, P. B., Newland, H., Renneberg, C., Kolar, P., Tsurkan, M., et al. (2015). Tackling cell transplantation anoikis : an injectable, shape memory cryogel microcarrier platform material for stem cell and neuronal cell growth. Nano Micro. Small 11, 5047–5053. doi: 10.1002/smll.201500898
Odabaşi, M., Baydemir, G., Karatas, M., and Derazshamshir, A. (2010). Preparation and characterization of metal-chelated poly (HEMA-MAH) monolithic cryogels and their use for DNA adsorption. J. Appl. Polym. Sci. 116, 1306–1312. doi: 10.1002/app.31505
Oelschlaeger, C., Bossler, F., and Willenbacher, N. (2016). Synthesis, structural and micro-mechanical properties of 3D hyaluronic acid-based cryogel scaffolds. Biomacromolecules 17, 580–589. doi: 10.1021/acs.biomac.5b01529
Önnby, L. (2016). “Application of cryogels in water and wastewater,” in Supermacroporous Cryogel, pp. 332–355.
Önnby, L., Harald, K., and Nges, I. A. (2015). Cryogel-supported titanate nanotubes for waste treatment : impact on methane production and bio-fertilizer quality. J. Biotechnol. 207, 58–66. doi: 10.1016/j.jbiotec.2015.05.014
Onorevoli, A. B., Pereira, G., Jacques, A., and Jacques, A. (2018). Characterization of feedstock and biochar from energetic tobacco seed waste pyrolysis and potential application of biochar as an adsorbent Bruna. J. Environ. Chem. Eng. 6, 1279–1287. doi: 10.1016/j.jece.2018.01.039
Orakdogen, N., and Boyaci, T. (2016). pH-stimulus on –off switching behavior and improved response rate of slightly charged poly (N, N -dimethylaminoethyl methacrylate) nano-sized composites with incorporated Laponite as crosslinker. REACT 102, 82–92. doi: 10.1016/j.reactfunctpolym.2016.03.008
Otero-gonzález, L., Mikhalovsky, S. V., Václavíková, M., Trenikhin, M. V., Cundy, A. B., Savina, I. N., et al. (2020). Novel nanostructured iron oxide cryogels for arsenic (As (III)) removal. J. Hazard. Mater. 381, 120996. doi: 10.1016/j.jhazmat.2019.120996
Oztoprak, Z., and Hekimoglu, T. (2014). Porous rubber cryogels : effect of the gel preparation temperature. Polym. Bull. 71, 1983–1999. doi: 10.1007/s00289-014-1167-5
Özverdi, A., and Erdem, M. (2006). Cu2+,Cd2+ and Pb2+ adsorption from aqueous solutions by pyrite and synthetic iron sulphide. J. Hazard. Mater. 137, 626–632. doi: 10.1016/j.jhazmat.2006.02.051
Papancea, A., Valente, A. J. M., and Patachia, S. (2010). PVA cryogel membranes as a promising tool for the retention and separation of metal ions from aqueous solutions. J. Appl. Polym. Sci. 118, 1567–1573. doi: 10.1002/app.32514
Park, J. H., and Min, B. G. (2012). Preparation of Poly (vinyl alcohol)/ hydroxyapatite cryogels for the removal of lead ions in water. Text. Sci. Eng. 49, 279–283. doi: 10.12772/TSE.2012.49.5.279
Plieva, F. M., Kirsebom, H., and Mattiasson, B. (2011). Preparation of macroporous cryostructurated gel monoliths, their characterization and main applications. J. Sep. Sci. 34, 2164–2172. doi: 10.1002/jssc.201100199
Plieva, F. M., Lozinsky, V. I., Yu, I., and Mattiasson, B. (2003). Direct chromatographic capture of enzyme from crude homogenate using immobilized metal affinity chromatography on a continuous supermacroporous adsorbent. J. Chromatogr. A 986, 275–290. doi: 10.1016/S0021-9673(02)01871-X
Plieva, F. M., and Mattiasson, B. (2007). Review Macroporous gels prepared at subzero temperatures as novel materials for chromatography of particulate-containing fluids and cell culture applications. J. Sep. Sci. 30, 1657–1671. doi: 10.1002/jssc.200700127
Purohit, S., Kumar, M., Tanmoyendu, C., Kanhaiya, C., Yadav, L., and Satapathi, S. (2020). Rapid removal of arsenic from water using metal oxide doped recyclable cross - linked chitosan cryogel. SN Appl. Sci. 2, 1–10. doi: 10.1007/s42452-020-2525-6
Red, L. R., Momeni, A., Farshi, B., Irani, M., and Mahmoudi, M. (2014). Removal of Ni2+ and Cd2+ ions from aqueous solutions using electrospun PVA/zeolite nanofibrous adsorbent. Chem. Eng. J. 256, 119–127. doi: 10.1016/j.cej.2014.06.066
Renu, M. A., and Singh, K. (2017). Heavy metal removal from wastewater using various adsorbents : a review. J. Water Reuse Desalin. 12, 387–419. doi: 10.2166/wrd.2016.104
Rie, J., Van, D.eclercq, H., Hoorick, J., Van, Dierick, M., and Vlierberghe, S. (2015). Cryogel-PCL combination scaffolds for bone tissue repair. J. Mater. Sci. Mater. Med. 26, 123. doi: 10.1007/s10856-015-5465-8
Sahiner, N., Demirci, S., Sahiner, M., and Yilmaz, S. (2015). The use of superporous p (3-acrylamidopropyl) trimethyl ammonium chloride cryogels for removal of toxic arsenate anions. J. Environ. Manage. 152, 66–74. doi: 10.1016/j.jenvman.2015.01.023
Sahiner, N., and Seven, F. (2014). The use of superporous p (AAc (acrylic acid)) cryogels as support for Co and Ni nanoparticle preparation and as reactor in H2 production from sodium borohydride hydrolysis. Energy 71, 170–179. doi: 10.1016/j.energy.2014.04.031
Sarkaya, K., Bakhshpour, M., and Denizli, A. (2019). Ag+ ions imprinted cryogels for selective removal of silver ions from aqueous solutions. Sep. Sci. Technol. 54, 2993–3004. doi: 10.1080/01496395.2018.1556300
Savina, I. N., Cnudde, V., Hollander, S. D., Hoorebeke, L., Van, M., Yu, I., et al. (2007). Cryogels from poly(2-hydroxyethyl methacrylate): macroporous, interconnected materials with potential as cell scaffolds. Soft Matter 3, 1176–1184. doi: 10.1039/b706654f
Savina, I. N., English, C. J., Whitby, R. L. D., Zheng, Y., Leistner, A., Mikhalovsky, S. V., et al. (2011). High efficiency removal of dissolved As (III) using iron nanoparticle-embedded macroporous polymer composites. J. Hazard. Mater. 192, 1002–1008. doi: 10.1016/j.jhazmat.2011.06.003
Savina, I. N., Zoughaib, M., and Yergeshov, A. A. (2021). Design and assessment of biodegradable macroporous cryogels as advanced tissue engineering and drug carrying materials. Gels 7, 79. doi: 10.3390/gels7030079
Sayil, C., and Okay, O. (2001). Macroporous poly (N-isopropyl) acrylamide networks: formation conditions. Polymer (Guildf). 42, 7639–7652. doi: 10.1016/S0032-3861(01)00279-8
Saylan, Y., and Denizli, A. (2019). Supermacroporous composite cryogels in biomedical applications. Gels 5, 20. doi: 10.3390/gels5020020
Shih, T., Blacklow, S. O., Li, A. W., Freedman, B. R., Bencherif, S., Koshy, S. T., et al. (2018). Injectable, tough alginate cryogels as cancer vaccines. Adv. Healthc. Mater. 12, 1701469. doi: 10.1002/adhm.201701469
Shin, K., Hong, J., and Jang, J. (2011). Heavy metal ion adsorption behavior in nitrogen-doped magnetic carbon nanoparticles: Isotherms and kinetic study. J. Hazard. Mater. 190, 36–44. doi: 10.1016/j.jhazmat.2010.12.102
Shu, Y., Huang, R., and Wei, X. (2017). Pb (II) Removal using TiO2-embedded monolith composite cryogel as an alternative wastewater treatment method. Water Air Soil Pollut 228, 375. doi: 10.1007/s11270-017-3559-2
Smedley, P. L., and Kinniburgh, D. G. (2002). A review of the source, behaviour and distribution of arsenic in natural waters. Appl. Geochemistry 17, 517–568. doi: 10.1016/S0883-2927(02)00018-5
Sun, Q., Li, Y., Tang, T., Yuan, Z., and Yu, C. (2013). Removal of silver nanoparticles by coagulation processes. J. Hazard. Mater. 261, 414–420. doi: 10.1016/j.jhazmat.2013.07.066
Sur, S., Pashuck, E. T., Guler, M. O., Ito, M., Stupp, S. I., and Launey, T. (2012). A hybrid nano fiber matrix to control the survival and maturation of brain neurons. Biomaterials 33, 545–555. doi: 10.1016/j.biomaterials.2011.09.093
Tabakli, B., Topçu, A. A., Döker, S., and Uzun, L. (2015). Particle-assisted ion-imprinted cryogels for selective Cd II ion removal. Ind. Eng. Chem. Res. 54, 1816–1823. doi: 10.1021/ie504312e
Tamahkar, E., Bakhshpour, M., Andaç, M., and Denizli, A. (2017). Ion imprinted cryogels for selective removal of Ni(II) ions from aqueous solutions. Sep. Purif. Technol. 179, 36–44. doi: 10.1016/j.seppur.2016.12.048
Tang, C., Brodie, P., Brunsting, M., and Chiu, K. (2020). Carboxylated cellulose cryogel beads via a one-step ester crosslinking of maleic anhydride for copper ions removal. Carbohydr. Polym. 242, 116397. doi: 10.1016/j.carbpol.2020.116397
Tatykhanova, G. S., Sadakbayeva, Z. K., Berillo, D., Galaev, I., Abdullin, K. A., Adilov, Z., et al. (2012). Metal complexes of amphoteric cryogels based on allylamine and methacrylic acid. Macromol. Symp. 317, 18–27. doi: 10.1002/masy.201100065
Tchounwou, P. B., Yedjou, C. G., Patlolla, A. K., and Sutton, D. J. (2012). “Heavy Metal Toxicity and the Environment,” in Molecular, Clinical and Environmental Toxicology, Experientia Supplementum, pp. 133–164.
Tekin, K., Uzun, L., Bektas, S., and Denizli, A. (2011). Preparation and characterization of composite cryogels containing imidazole group and use in heavy metal removal. React. Funct. Polym. 71, 985–993. doi: 10.1016/j.reactfunctpolym.2011.06.005
Textile, M. (2017). Influence of stage cooling method on pore architecture of biomimetic alginate scaffolds. Sci. Reports 7, 16150. doi: 10.1038/s41598-017-16024-x
Türkmen, D., Türkmen, M. Ö., Akgönüllü, S., and Denizli, A. (2021). Development of ion imprinted based magnetic nanoparticles for selective removal of arsenic (III) and arsenic (V) from wastewater. Sep. Sci. Technol. 21, 1–10. doi: 10.1080/01496395.2021.1956972
Ulery, B. D., Nair, L. S., and Laurencin, C. T. (2011). Biomedical applications of biodegradable polymers. Polym. Phys. 49, 832–864. doi: 10.1002/polb.22259
Vainerman, E. S., Lozinsky, V. I., and Rogozhin, S. V. (1981). Study of cryostructurization of polymer systems I . structure formation in solutions of thiol-containing polymers under freezing-thawing. Colloid Polym. Sci. 259, 1198–1201. doi: 10.1007/BF01525014
Volova, T. G., Yuri, S., Vinnik, S.hishatskaya, E. I., Markelova, N. M., and Zaikov, G. E. (2017). Natural-Based Polymers For Biomedical Applications.
Wang, J., and Chen, C. (2009). Biosorbents for heavy metals removal and their future. Biotechnol. Adv. 27, 195–226. doi: 10.1016/j.biotechadv.2008.11.002
Wang, J., and Yang, H. (2018). Superelastic and pH-responsive degradable dendrimer cryogels prepared by cryo-aza-michael addition reaction. Sci. Rep. 8, 7155. doi: 10.1038/s41598-018-25456-y
Wang, Y., Huang, H., Duan, S., Liu, X., Sun, J., Hayat, T., et al. (2018). A new application of a mesoporous hybrid of tungsten oxide and carbon as an adsorbent for elimination of Sr2+ and Co2+ from an aquatic environment. ACS Sustain. Chem. 6, 2462–2473. doi: 10.1021/acssuschemeng.7b03818
Wuana, R. A., Okieimen, F. E., and Imborvungu, J. A. (2010). Removal of heavy metals from a contaminated soil using organic chelating acids. Int. J. Environ. Sci. Technol. 7, 485–496. doi: 10.1007/BF03326158
Ya, V., Martin, N., Chou, Y., Chen, Y., and Choo, K. (2017). Electrochemical treatment for simultaneous removal of heavy metals and organics from surface finishing wastewater using sacrificial iron anode. J. Taiwan Inst. Chem. Eng. 7, 1–8.
Yao, K., Yun, J., Shen, S., and Chen, F. (2007). In-situ graft-polymerization preparation of cation-exchange supermacroporous cryogel with sulfo groups in glass columns. J. Chromatogr. A 1157, 246–251. doi: 10.1016/j.chroma.2007.05.015
Yari, M., Rajabi, M., Moradi, O., Yari, A., Asif, M., Agarwal, S., et al. (2015). Kinetics of the adsorption of Pb (II) ions from aqueous solutions by graphene oxide and thiol functionalized graphene oxide. J. Mol. Liq. 209, 50–57. doi: 10.1016/j.molliq.2015.05.022
Ye, S., Jin, W., Huang, Q., Hu, Y., Li, Y., Li, J., et al. (2017). Da-KGM based GO-reinforced FMBO-loaded aerogels for efficient arsenic removal in aqueous solution. Int. J. Biol. Macromol. 94, 527–534. doi: 10.1016/j.ijbiomac.2016.10.059
Yetiskin, B., and Okay, O. (2017). High-strength silk fi broin scaffolds with anisotropic mechanical properties. Polymer (Guildf). 112, 61–70. doi: 10.1016/j.polymer.2017.01.079
Zhang, J., Zhong, T., Xiang, Y., Zhang, X., and Feng, X. (2021). Microfibrillated cellulose reinforced poly (vinyl imidazole) cryogels for continuous removal of heavy metals. J. Appl. Polym. Sci. 138, 51456.51456. doi: 10.1002/app.51456
Zhang, Y., Chi, H., Zhang, W., Sun, Y., Liang, Q., Gu, Y., et al. (2014). Highly efficient adsorption of copper ions by a pvp-reduced graphene oxide based on a new adsorptions mechanism. Nano-Micro Lett. 6, 80–87. doi: 10.1007/BF03353772
Zhao, G., Huang, X., Tang, Z., Huang, Q., Niu, F., and Wang, X. (2018). Polymer-based nanocomposites for heavy metal ions removal from aqueous solution: a review. Polym. Chem. 9, 3562–3582. doi: 10.1039/C8PY00484F
Zheng, Y., Gun, V. M., Howell, C. A., Sandeman, S. R., Phillips, G. J., Kozynchenko, O. P., et al. (2012). Composites with macroporous poly(vinyl alcohol) cryogels with attached activated carbon microparticles with controlled accessibility of a surface. Appl. Mater. Interfaces 4, 5936–5944. doi: 10.1021/am301577c
Zhitkovich, A. (2011). Chromium in drinking water: sources, metabolism, and cancer risks. Chem. Res. Toxicol. 24, 1617–1629. doi: 10.1021/tx200251t
Zhong, T., Feng, X., Sun, L., Zhang, J., Tian, Y., and Zhang, X. (2021). Highly effective adsorption of copper ions by poly(vinyl imidazole) cryogels. Polym. Bull. 78, 5873–5890. doi: 10.1007/s00289-020-03413-y
Keywords: heavy metal ion, adsorbents, cryogels, removal, wastewater
Citation: Türkmen D, Bakhshpour M, Akgönüllü S, Aşır S and Denizli A (2022) Heavy Metal Ions Removal From Wastewater Using Cryogels: A Review. Front. Sustain. 3:765592. doi: 10.3389/frsus.2022.765592
Received: 27 August 2021; Accepted: 28 January 2022;
Published: 23 March 2022.
Edited by:
Mohamed Helmy Abdel-Aziz, Alexandria University, EgyptReviewed by:
Mohamed Bassyouni, Port Said University, EgyptAhmed Elgarahy, Port Said University, Egypt
Copyright © 2022 Türkmen, Bakhshpour, Akgönüllü, Aşır and Denizli. This is an open-access article distributed under the terms of the Creative Commons Attribution License (CC BY). The use, distribution or reproduction in other forums is permitted, provided the original author(s) and the copyright owner(s) are credited and that the original publication in this journal is cited, in accordance with accepted academic practice. No use, distribution or reproduction is permitted which does not comply with these terms.
*Correspondence: Adil Denizli, ZGVuaXpsaUBoYWNldHRlcGUuZWR1LnRy