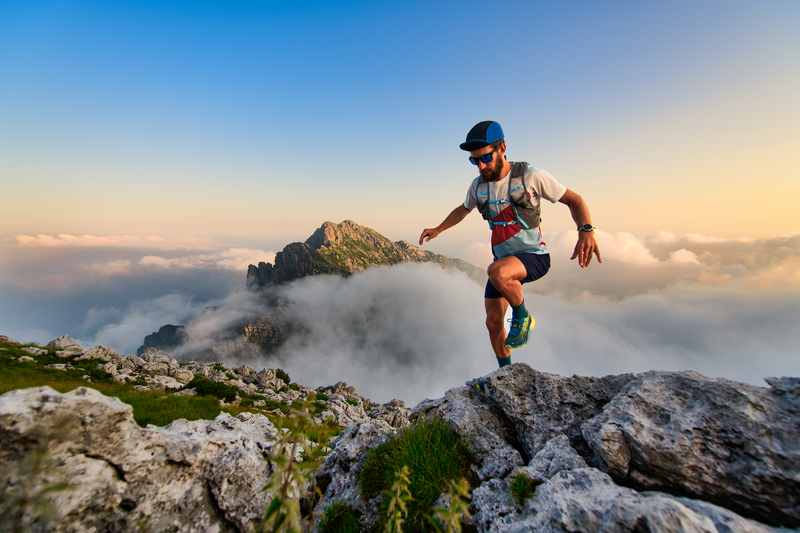
94% of researchers rate our articles as excellent or good
Learn more about the work of our research integrity team to safeguard the quality of each article we publish.
Find out more
REVIEW article
Front. Space Technol. , 11 October 2024
Sec. Space Exploration
Volume 5 - 2024 | https://doi.org/10.3389/frspt.2024.1457487
This article is part of the Research Topic Innovation in Medical Space Technology View all 7 articles
Innovative solutions are required to make long-duration space missions feasible. Crew performance and health is paramount to the success of anticipated Moon and Mars missions. Metabolic reduction via a quasi-torpor state is a possible mitigation strategy that can reduce consumable payload, which is necessary given the lack of available resupply options, and to reduce psychological stress, which is a risk for such lengthy missions. Even in lunar or cis-lunar missions, a quasi-torpor state could be implemented as an emergency countermeasure for critical situations where life support becomes limited. However, to date no studies have tested a quasi-torpor state in humans, and the impacts of intentional prolonged metabolic reduction on physiological and psychological parameters are unknown. To this end, we planned a three-phase study to provide proof-in-principle of the tolerability, feasibility, and side effects of a non-intravenous alpha-2-adrenergic receptor agonist for moderate sedation. This was accomplished by 1) determining the dosing and metabolic effects for different non-intravenous routes of alpha-2-adrenergic receptor agonist drugs; 2) assessing the degree of metabolic reduction and side effects during a 24-h quasi-torpor protocol; and 3) evaluating participant performance and total metabolic reduction achieved over a 5-day quasi-torpor protocol. We also aim to determine how skeletal muscle health and performance are affected by this quasi-torpor state. Quasi-torpor induced changes in skeletal muscle health and performance, as well as impacts on cognition and psychological stress, also have implications for terrestrial situations that result in prolonged confinement (e.g., austere environments such as submarine or remote scientific or military deployment and protracted critical illness). The findings of this three-phase study will be immediately applicable as a rescue strategy for emergencies during current or upcoming space missions. They will also identify key physiological and practical questions that need to be addressed for future deployment in long-duration space missions. This paper reviews the relevant literature that informed our rationale and approaches for this three-phase study.
Long-duration space missions will require innovative strategies to reduce consumables, counteract psychological stress due to mission duration and confinement, and improve survivability during emergencies that threaten the crew’s resources or life support (NASA Human Research Roadmap) For example, the anticipated Mars mission will involve a minimum 760-day roundtrip journey from Earth to Mars during which time there will be no opportunity for resupply and communications will be delayed (NASAa; NASAb) One potential solution to address the aforementioned risks is metabolic reduction, similar to the torpor or hibernation strategy that many animals employ during periods of resource scarcity. While much has been observed regarding torpor and hibernation in mammals, (Ruf and Geiser, 2015; Junkins et al., 2022) little is known about how a similar strategy might be induced or imitated in humans. Small mammals such as 13-lined ground squirrels and Arctic ground squirrels are considered obligate hibernators that hibernate on a circannual rhythm for several months. (Grabek et al., 2019; Frare and Drew, 2021; Jinka et al., 2011) During hibernation, these squirrels markedly reduce their core body temperature to 4
We are currently investigating whether a medication-induced prolonged sleep state can safely reduce metabolic rate in humans. Our strategy focuses on increasing the ratio of sleep:awake time using a combination of light sedation and mild external cooling to reduce the resting metabolic rate. We developed a three-phase experimental plan in healthy participants to 1) determine an efficacious non-intravenous dose of an alpha-2-adrenergic receptor (A2AR) agonist drug; 2) test the ability to induce sedation and metabolic reduction in a 24-h protocol; 3) test the ability to maintain drug-enhanced sleep and metabolic reduction over a 5-day protocol. We have completed and reported the first phase of this study (Callaway et al., 2024). We are measuring the psychological, cognitive, and muscle health changes associated with this prolonged sleep state. We have expanded upon our extensive prior experience with both cooling and sedation in critically ill and healthy humans to maximize metabolic reduction and minimize invasiveness of the procedures (Moore et al., 2008; Sonder et al., 2018; Callaway et al., 2020; Callaway et al., 2015; Rittenberger et al., 2019). This paper describes our approach to inducing prolonged sleep to facilitate metabolic reduction in humans, discusses our rationale for experimental choices made, and highlights knowledge gaps for future studies to explore and optimize this approach in humans.
Metabolic rate comprises the consumption of fuel and oxygen (O2), which produces carbon dioxide (CO2), work, and heat. Food provides the metabolic fuel for humans, and typical energy consumption for a 70 kg adult man aged 30–59.9 years engaged in moderate daily activity is 2,750 kilocalories/day, 190 kJ/day, or 11.4 MJ (3,166.67 W-hours) (Food and Agriculture Organization of the United Nations, 2004). Oxygen consumption is proportional to the fuel consumed in aerobic metabolism, and a resting adult consumes about 3.5 mL O2/kg/min (1 metabolic equivalent, MET) (Food and Agriculture Organization of the United Nations, 2004; Jetté et al., 1990). This rate can increase briefly to more than 10 METs during vigorous exercise (Jetté et al., 1990). Differing amounts of CO2 are produced for each unit of O2 consumed, depending on the proportion of fuel sources (fat, carbohydrate, or protein). Assuming humans sleep 7–8 h per 24-h day (∼30%), their metabolic rate is expected to be around 1.0 MET (Jetté et al., 1990). During waking hours, even quietly working humans will have a mean metabolic rate of 1.4 METs. (Jetté et al., 1990). Thus, daily energy consumption will average (30% x 1.0 METs) + (70% x 1.4 METs) = 1.3 METs.
We speculate that increasing the proportion of sleep time to 80% of the day will reduce the average metabolic rate: (80% x 1.0 METs) + (20% x 1.4 METs) = 1.1 METs. By itself, this change in the proportion of sleep time from 30% of the day to 80% of the day would afford a 20% reduction (from 1.3 to 1.1 METs) in total fuel and oxygen consumption each day (Figure 1A, B).
Figure 1. Rationale for Approach. Point (A). At baseline, humans sleep 30% of the day and have a resting metabolic rate around 1.0 METs. Point (B). Increasing sleep duration to 80% of the day reduces daily O2 and fuel consumption by 20%. Point (C). Reducing metabolic rate during sleep to 0.8 METs further reduces O2 and fuel consumption to 30% below baseline.
Anesthetic drugs can further reduce metabolic rate below quiet resting rates. We speculate that drug-induced sleep could allow metabolic rate to decline below 1.0 METs. For an individual who is sleeping 80% of the day, modest reductions in resting metabolic rate can provide significant savings in fuel and O2 consumption. For example, a 20% reduction in resting metabolic rate from 1.0 METs to 0.8 METs would reduce average metabolic rate: (80% x 0.8 METs) + (20% x 1.4 METs) = 0.9 METs. Thus, the combination of prolonging sleep to 80% of the day and metabolic reduction of 20% during sleep, would reduce overall fuel and O2 consumption by 30% (from 1.3 to 0.9 METs) (Figure 1C).
Reduction of temperature also decreases metabolic rate by slowing the chemical reactions throughout the body. We have measured a 5.2% reduction in O2 consumption for each 1°C decrease in core body temperature in sedated healthy volunteers for whom drugs inhibited shivering (Flickinger et al., 2024). Similar reductions in cerebral metabolic rate have been reported under general anesthesia (Michenfelder and Milde, 1991). It is conceivable that reducing core body temperature would further decrease resting metabolic rate and could amplify the metabolic reduction provided by anesthetic drugs.
There are at least three important practical considerations for implementation of an anesthetic-induced reduction in metabolic rate as a spaceflight countermeasure. First, drug administration should be minimally invasive. Peripheral intravenous catheters used in hospital care have a failure rate of 4%–9% per day (Takahashi et al., 2020). While central venous catheters may have a lower failure rate of 0.3% per day, they are more complex to insert and have thrombotic and infectious complications (McManus et al., 2024). Maintaining intravenous catheters for long-duration spaceflight is impractical. Therefore, to avoid intravenous drugs, we sought an oral, mucosal or transcutaneous drug regimen. Second, mechanical support of respiration is complex and hazardous. Non-invasive ventilation can avoid invasive airway management, but it also has failure rates of 30%–70% when used for acute respiratory failure (Rolle et al., 2022), and over 20% incidence of adverse events when used for chronic respiratory conditions (Wilson et al., 2020). Therefore, we sought a drug that produces minimal respiratory depression. Third, constant sedation requires plans to manage urine, solid waste, and nutrition. In medical settings, catheters to manage these needs also have high failure rates and complications (Gomes et al., 2015). Therefore, we targeted a regimen that included some waking time during each 24-h period during which a participant could self-manage nutrition and excretion.
We planned a three-phase study to 1) test the dosing and metabolic effects of a non-intravenous A2AR agonist for light sedation during a 6-h laboratory visit; 2) assess the degree of metabolic reduction achieved by our selected drug over a 24-h laboratory visit; and 3) evaluate the tolerability and feasibility of the protocol, as well as the ability to reduce metabolic rate and increase sleep time to 80% over five experimental days. The primary outcome for these experiments is the change in metabolic rate during the intervention relative to baseline. We measure the baseline resting metabolic rate in each participant prior to initiation of the drug, and then observe the change in metabolic rate over time following drug administration. We collect an array of physiologic data as well as blood samples for pharmacokinetic analysis. Participants complete serial psychophysiological batteries. We use performance and non-invasive muscle composition measures to assess changes in muscle health and function.
In Phase 1 and Phase 2 experiments, we measure O2 consumption and CO2 production captured via canopy (during rest) or facemask (during exercise) using a Parvo TrueOne 2400 metabolic cart (Parvo Medics, Salt Lake City, UT, United States). The difference between ambient and exhaled CO2 and O2 values allows calculation of oxygen consumption (VO2), CO2 production (VCO2), respiratory exchange ratio, and total energy expenditure (de and Weir, 1948). In Phase 3, we measure changes in metabolic rate using both serial indirect calorimetry assessments via the Parvo metabolic cart as well as the gold-standard doubly labeled water method.
Additional physiologic measurements include electrocardiogram, respiratory rate, pulse plethysmography, blood pressure, and core temperature. We use an ingested telemetry capsule (eCelsius, BodyCap, Herouville Saint-Claire, France) to continuously capture gastrointestinal core temperature. In Phase 1 experiments we examined heat flux from the body surface using one-cm2 sensors on the forehead, torso, arm and leg; we collected respiratory rate using a circumferential chest belt; and we used bioreactance to measure cardiac stroke volume and estimate cardiac output. We simplified our array of physiologic sensors and monitoring for longer Phase 2 and Phase 3 experiments for subject comfort, and focused on collecting continuous electrocardiogram, pulse plethysmography, and core temperature with serial blood pressure measurements.
We measure changes in muscle composition using electrical impedance myography (EIM, Myolex device, Myolex, Boston, MA, United States), a non-invasive method to measure muscle fiber consistency based on the properties of electrical impedance and capacitance that can be altered by free water, connective tissue, and fat (Sanchez and Rutkove, 2017; Rutkove and Sanchez, 2019). EIM allows us to evaluate changes in muscle composition over time and is highly reproducible. Previously, EIM has been employed to measure changes in muscle composition and health in neurodegenerative diseases like amyotrophic lateral sclerosis (Tarulli et al., 2009), and has been used in rats to measure changes induced by partial weight-bearing and hindlimb suspension models of atrophy that simulate weightlessness-induced muscle changes, (Mortreux et al., 2018),, (Mortreux et al.),, (Mortreux et al., 2019a; Semple et al., 2020), as well as in mice in low earth orbit (Sung et al.). Presently, we are using EIM as a virtual muscle biopsy (Mortreux et al., 2019b) in Phase 2 and Phase 3 experiments to track changes in muscle groups highly susceptible to unloading/disuse atrophy: Tibialis Anterior, Gastrocnemius, Quadriceps Femoris, Biceps Brachii. For our experiments, EIM is obtained prior to drug initiation and again prior to exercise at Time 23-h post drug initiation.
We also conduct functional strength assessments measuring changes in isokinetic muscle strength and mean power (e.g., changes in peak torque and concentric/eccentric velocity) to assess muscle health and function for Phase 2 and Phase 3 experiments. Grip strength, considered the simplest strength test and a vital sign of health to follow over time, is obtained at baseline (using a Jamar dynamometer, Chicago, IL, United States) and at the end of each drug administration period in Phase 1, 2, and 3 experiments.
During the 5-day protocols, we collect plasma and urine samples to measure changes in creatinine kinase M, nitrogen, 3-methylhistidine, glucose, and ketones as surrogates for catabolic or anabolic states. We hypothesize that the increased proportion of low activity time, combined with reduced caloric intake, could result in a catabolic state.
Finally, we employ a suite of computer-based psychometric tests to assess attention, memory, information processing, and fine motor skills (Joggle Research, Seattle, WA, United States) (Basner et al., 2015) as well as multidomain performance under increased task load (Multiple Attribute Test Battery-II). (Hart, 2006).
In Phase 1, we evaluated the ability of two A2AR agonists, combined with external cooling using gel-adhesive pads on the back of the torso (Arctic Sun, Franklin Lakes, NJ, United States), to reduce metabolic rate and core temperature. We tested dexmedetomidine (single 1 μg/kg sublingual dose vs single 4 μg/kg dose swallowed orally vs single sublingual loading dose of 2 μg/kg followed by a 1 μg/kg/hour dexmedetomidine subcutaneous infusion for 6 h) and tizanidine (8 mg or 16 mg dose taken once orally). The protocol methods for Phase 1 have been described in detail elsewhere (Callaway et al., 2024). For Phase 1, we recruited healthy volunteers aged 18–55 years, without significant comorbidities (non-smokers without sleep disorders or chronic medical problems) and of typical body weight (>55 kg), body mass index (18.5–30 kg/m2), resting heart rate (50–100 beats/minute), resting blood pressure (100–150 systolic, 60–90 diastolic), normal electrocardiogram (no conduction abnormalities or dysrhythmias), Epworth Sleepiness Scale (<11), and maximum oxygen consumption (VO2 max, must be greater than one standard deviation below the mean for age or less than two standard deviations above the mean for age). We used the Bruce Treadmill Protocol (Will and Walter, 1999) with Parvo TrueOne 2400 metabolic cart and face mask (Parvo Medics, Salt Lake City, UT, United States) to assess VO2 max.
In Phase 2, we tested if a loading dose of dexmedetomidine (2 μg/kg sublingual) followed by an 18-h subcutaneous infusion of dexmedetomidine (either 0.5 μg/kg/hour or 1.0 μg/kg/hour, randomized) could induce 20-h of rest or sleep during a 24-h laboratory session. In this phase of the study, we streamlined several physiologic assessments to minimize the intrusiveness of various sensors and monitors. Subjects completed the same screening processes as in Phase 1. To determine baseline activity, participants completed 1 week of actigraphy and a sleep diary prior to the study day. Participants then repeated actigraphy and sleep diaries for 1 week after protocol to assess post-protocol recovery.
We assessed participants’ ability to complete an exercise regimen at 23-h after the start of drug infusion because regular exercise will be critical for maintaining muscle health on a long-duration space mission. Participants performed a series of isometric exercises while lying reclined in a stretcher before moving on to a series of resistance exercises based around an isoinertial flywheel (Table 1). The exercise regimen takes approximately 1 hour with built-in rest between sets. Participants are fitted with a facemask to capture their VO2 during exercise. We used EIM to non-invasively ascertain information regarding muscle health and we obtained measurements before drug initiation and again prior to exercise at 23 h after the start of drug.
Participants may eat and drink at any time during the protocol; however, they are encouraged to ingest a minimum of one-16 oz bottle of Gatorade, one-16 oz bottle of water, and one-10 oz protein shake (either vegan pea protein or whey-based protein) prior to engaging in exercise.
Phase 3 is a proof-of-concept study in which participants will engage in five consecutive 24-h protocols (Figure 2), flanked by 1-week actigraphy and sleep diary assessments. Doubly labeled water is used to calculate total energy expenditure over the 5-day period. Doubly labeled water is a non-invasive technique that relies on the rates of excretion of heavy isotopes of oxygen and hydrogen, from a specific dose of water, to calculate energy expenditure (Trabulsi et al., 2003; Tooze et al., 2007). Pre-study and post-study dual x-ray absorptiometry will be obtained, in addition to daily EIM measurements, to assess changes in body composition (i.e., fat mass and fat-free mass). Nutrition is of paramount importance to muscle health and function and a participants’ ability to perform daily tasks during long duration space flight. In consideration of the impact of prolonged bed rest, we also developed a diet with rational composition and timing to optimize lean body mass preservation and accompany the exercise regimen (Figures 3, 4).
Figure 2. Phase 2 and 3 Experiment Overview In Phase 2, the participant undergoes a resting metabolic rate, then the subcutaneous infusion is initiated and the participant lies quietly for up to 20 h. Subsequently the participant exercises while having their oxygen consumption measured. The participant also performs cognitive testing before, during and after the drug infusion. This cycle is repeated for a total of five 24-h periods in Phase 3.
Figure 3. NASA Standard Diet (control diet) based on National Academy of Medicine Dietary Reference Intake Equations*.
Innovative solutions that maintain or augment crew performance and health can increase feasibility and safety of long-duration space missions. Crew performance and health is paramount to the success of anticipated Moon and Mars missions. A quasi-torpor state can reduce consumable payload, which is necessary given the lack of available resupply options, and reduce psychological stress, which is a risk for years-long missions. Even in lunar or cis-lunar missions, quasi-torpor could be an emergency countermeasure for critical situations where life support becomes limited. For example, reducing metabolic rate could slow the accumulation of CO2 after a catastrophic failure of CO2 removal systems long enough to allow rescue, repair, or return to earth.
However, to date there are no prior studies testing or implementing quasi-torpor in humans. We are providing proof-in-principle of the tolerability, feasibility, and side-effects of this intervention. We are also determining how skeletal muscle health and performance are affected by this quasi-torpor state. Our results so far show that it is feasible to reduce human metabolism using non-intravenous A2AR agonists combined with minimal external cooling (Callaway et al., 2024). If this strategy is tolerable over 24-h and 5-day protocols, rapid deployment may be possible. Focusing on quasi-torpor as a means to study changes in skeletal muscle health and performance, and changes in cognition and psychological stress, will also have implications for terrestrial situations that result in prolonged confinement (e.g., austere environments such as submarine or remote scientific or military base; protracted critical illness). The findings of this three-phase study will be directly applicable to current and near-term long-duration space missions and will identify physiological and practical questions that need to be answered for rapid deployment in the next few years. Potential protocols will require more testing in ground analogs of long-duration space missions.
In the next sections, we discuss our reasoning for certain decisions made when planning our experiments and lessons learned from our results to date. We have successfully completed Phases 1 and 2 of our three-phase experimental plan, and are currently enrolling in Phase 3, during which time we will incorporate doubly labeled water to quantify energy expenditure over the 5-day period. Our rationale was informed by the wealth of information already available in the anesthesia literature, animal torpor and hibernation studies, the NASA Technical Reports Server archives, and bed rest literature regarding exercise and nutrition countermeasures. There are many remaining questions that require solutions prior to testing of this protocol in ground analogs of long-duration space missions. As we implement different phases of this experiment we are left with more questions and knowledge gaps than at the outset.
We considered previous studies of sedative medications’ effects on metabolism and known mechanisms of action and side effects when selecting candidate medications for this protocol. There are multiple sedative and hypnotic medication classes and intraclass options that can prolong sleep and potentially inhibit cold-induced thermogenesis, thereby reducing the resting metabolic rate. We sought to test medications that are unlikely to be habit-forming or abused and that do not induce respiratory depression or deep sedation. We also desired a rapid onset and cessation of drug effects for safety reasons; if a future astronaut needs to emerge from quasi-torpor during an emergency, a medication with a short half-life and inactive metabolites provides faster return to baseline. Additionally, we need a drug formulation that does not require intravenous administration as this is not feasible for long-duration spaceflight. These parameters immediately precluded the use of inhaled anesthetics, ketamine, propofol, opioids, sedating antidepressants, melatonin, and sedating antipsychotics.
Benzodiazepines have been used in prior low earth orbit missions as needed (Dijk et al., 2001), and at high doses have been shown to reduce metabolism and lower the cold thermogenesis temperature threshold (Kurz et al., 1995; Hostler et al., 2009). Benzodiazepines also alter rapid eye movement sleep (REM) and deep sleep resulting in degradation of sleep quality following use as well as a rebound increase in REM sleep after cessation of use. (Griffin et al., 2013; Kales et al., 1975), These drugs impair performance and can have significant carry-over effects into subsequent work cycles (Griffin et al., 2013). Additionally, benzodiazepines have the potential for abuse, induce respiratory depression, and can quickly result in tolerance requiring higher doses to maintain the same effect (Griffin et al., 2013). Similarly, barbiturates are known to result in even greater respiratory depression, sleep disturbances, decreased performance, and have a high potential for tolerance and abuse. (Kales et al., 1975)., (Skibiski et al., 2024),
Non-benzodiazepine sedative-hypnotic agents include selective gamma-aminobutyric acid-A (GABAA) agonists such as the imidazopyridine zolpidem (Ambien), the cyclopyrrolones zopiclone and eszopiclone (Imovane and Lunesta, respectively), and the pyrazolopyrimidines zaleplon (Sonata) and Indiplon (Monti et al., 2015). Their selectivity reduces unwanted side effects of tolerance, abuse, and rebound insomnia compared to other sedative-hypnotic medications including benzodiazepines and barbiturates, and also reduces alteration of non-REM and REM sleep architecture (Monti et al., 2015). All agents in this class are relatively rapidly acting with relatively short half-lives in their immediate-release forms (Monti et al., 2015); however, only oral formulations are available which limits titration of these drugs for longer periods of time. These drugs have been used by NASA previously, but effects on metabolic rate are not known. Alternative formulations may make them suitable for testing in a future metabolic reduction study.
A1-adenosine receptors are highly distributed in the central nervous system and peripherally. A1-adenosine receptors are of interest as drug targets because animals such as ground squirrels increase their levels of adenosine monophosphate during hibernation via activation of these receptors (Frare and Drew, 2021; Jinka et al., 2011; Silvani et al., 2018). A1-adenosine receptor agonists have been investigated in mice and rats where they produce significant hypotension; demonstration of safety in larger animals is an area for ongoing research (Silvani et al., 2018). No human trials are yet available; therefore, these drugs may have potential use applications in the future but are not yet suitable for our experiments.
Orexin receptor antagonist drugs are a relatively newer class of medications to treat insomnia (Herring et al., 2012; Scammell and Winrow, 2011). Orexin receptors are found in the hypothalamus and are vital for wakefulness; narcolepsy develops due to damage or loss of orexin-producing neurons (Scammell and Winrow, 2011; Grimaldi et al., 2014). Orexin receptors are concentrated in the brain with minimal distribution in the periphery, resulting in fewer off-target effects by orexin receptor antagonists such as respiratory depression or hemodynamic instability, though the drugs can blunt the response to hypercarbia (Scammell and Winrow, 2011). These drugs do not appear to promote dependence or abuse. (Herring et al., 2012; Scammell and Winrow, 2011), Existing orexin receptor antagonists have a long half-life (9–13 h for a standard dose), so they are currently not ideal for our protocol (Herring et al., 2012).
Primary A2AR agonists include dexmedetomidine, tizanidine, xylazine, lofexidine, clonidine, guanabenz, guanfacine, and medetomidine (Giovannitti et al., 2015). A2AR agonists produce sedation via central inhibition of norepinephrine release from the presynaptic neuron resulting in reduced ascending noradrenergic activity (Giovannitti et al., 2015). Peripheral effects of norepinephrine and sympathetic inhibition include decreased blood pressure and heart rate (Giovannitti et al., 2015). A2AR agonists have varying affinities for alpha-1 and alpha-2 receptors, which may explain why different drugs in the class have varying degrees of hemodynamic change at doses that are equipotent for sedation (Giovannitti et al., 2015).
Xylazine and medetomidine are commonly used in veterinary anesthesia (Kamibayashi et al., 2000). They have both been found to be contaminants in the global fentanyl supply and can result in severe soft tissue destruction; therefore, they are not attractive candidates for our protocol (Drug Enforcement Administration, 2022). Clonidine and guanfacine are commonly used as antihypertensives, but they also possess sedative effects and have been used for opioid withdrawal and well as in autism spectrum disorders and attention deficit and hyperactivity disorders (Giovannitti et al., 2015; Kamibayashi et al., 2000). Clonidine is also sometimes used as an intravenous sedative outside the United States (Kamibayashi et al., 2000). Guanabenz is an older antihypertensive no longer used in the United States, so it is not an ideal candidate for our protocol (Kamibayashi et al., 2000). Clonidine and meperidine reduce post-operative shivering through their central A2AR effects, but meperidine is a potentially addictive opioid receptor agonist that can result in marked respiratory depression (Kamibayashi et al., 2000). Clonidine has been shown to reduce metabolic rate in a previous study (Takahashi et al., 1997). Lofexidine, similar to clonidine, has antihypertensive properties but is more commonly used to manage opioid withdrawal symptoms, though it carries a risk of QT-prolongation. Clonidine, guanfacine, and lofexidine have lengthy half-lives, which make them less titratable. We considered this a disadvantage for the purposes of our protocol.
Tizanidine is a commonly prescribed muscle relaxant and produces mild sedation as a side effect (Giovannitti et al., 2015). It is orally administered and generally well tolerated with minimal hemodynamic effects. Dexmedetomidine is usually administered intravenously, but it is also absorbed from mucous membranes and after enteral administration. It was recently approved for agitation as a sublingual film, and it is also available intranasally. We have explored intravenously administered dexmedetomidine in multiple previous studies (Callaway et al., 2015; Rittenberger et al., 2019; Flickinger et al., 2024; Rittenberger et al., 2021). A single bolus of 1 μg/kg dexmedetomidine can reduce shivering after cooling with intravenous fluids for up to 90 min (Callaway et al., 2015). Continued infusions at up to 1.5 μg/kg/hour allow sustained reductions of core body temperature (Rittenberger et al., 2019; Flickinger et al., 2024). After considering these and other A2AR agonist drugs, we chose to test tizanidine and dexmedetomidine in our Phase 1 trials.
In our current experiments, sublingual dexmedetomidine increased plasma levels to sedating levels within 20–30 min (Callaway et al., 2024). Swallowed oral dexmedetomidine had variable absorption which is perhaps affected by stomach contents or emptying. Dexmedetomidine has a rapid elimination and offset, which makes it more easily titrated relative to tizanidine. A sustained-release dexmedetomidine formulation could provide for longer drug delivery but would be less titratable. Ideally, a transcutaneous delivery system such as a patch, which exists for another A2AR agonist (Clonidine), could facilitate titration and accurate timing of drug-induced metabolic reduction for longer periods while allowing for more immediate cessation of drug effects than an ingested formulation. Currently, there is no available transcutaneous delivery system for dexmedetomidine; we are presently using a subcutaneous infusion as an approximation of a transcutaneous patch in our 24-h and 5-day protocols. This delivery system provides reliable and titratable plasma levels of drug with the ability to immediately discontinue drug delivery in cases of marked bradycardia or hypotension. The subcutaneous infusion is a minimally invasive approach for drug delivery, and we use the same subcutaneous catheters at those employed for insulin pumps in diabetes. These catheters can be maintained long-term and if needed can be placed by the user; however, these catheters may require repositioning every few days and do pose a risk of infection or skin complications. Given the lack of other transcutaneous delivery methods immediately available, we will employ the subcutaneous approach in our 24-h and 5-day protocols as this approach does provide proof in principle.
In our studies, alpha-2-adrenergic agonists did not reduce respiratory rate by any clinically significant amount (Callaway et al., 2024; Flickinger et al., 2024). Continuous pulse-oximetry detected no change in oxygenation during drug-induced sleep (Callaway et al., 2024). Dexmedetomidine is used in medical settings to facilitate weaning from mechanical ventilation, specifically because it can sedate without affecting respiratory drive or airway reflexes. Therefore, these drugs have a desirable profile relative to other anesthetics that cause respiratory depression or loss of airway protection. In a series of experiments comparing routes and doses of dexmedetomidine, inhibition of cold-induced thermogenesis was more reliable with plasma levels of 0.2–1.0 ng/mL (Callaway et al., 2024). These levels were achieved with oral bolus doses of 4 μg/kg or subcutaneous infusions of 1 μg/kg/hour (Callaway et al., 2024).
It is well known that astronauts generally have decreased sleep length on mission despite allowance for 8-h rest cycles (Dijk et al., 2001; Jones et al., 2022; Stampi, 1994). Astronauts demonstrate desynchronization of hormonal and activity rhythms from the artificially prescribed circadian schedule during space flight, resulting in cumulative sleep deprivation (Dijk et al., 2001). Hence, sleep medications are the second most commonly used medications during low earth orbit missions. (Dijk et al., 2001; Barger et al., 2014) We chose to maintain a 24-h earth-based cycle but alter the rest period by increasing rest time to 20-h with 4 hours of activity for our Phase 2 and 3 proof-of-concept ground-based studies. This choice allowed us to maximize potential metabolic reduction during the rest time while minimizing overall alterations in the 24-h earth-based cycle. It also simplified scheduling of laboratory activities. Because any quasi-torpor intervention could dramatically disrupt many circadian rhythms, alternative rest:activity ratios and total times should be investigated. For example, Mars has a 24.6-h day and synchronizing to the destination clock might optimize crew performance and wellbeing. Alternatively, longer rest:activity cycles, such as 30 or 36 h, might maximize metabolic savings.
Longer sleep durations have positive effects on astronaut neurobehavioral functioning in low earth orbit missions, (Dijk et al., 2001; Jones et al., 2022) yet too much sleep may indeed be detrimental to astronaut wellbeing or perceptions thereof. We hypothesize that longer durations of rest will reduce the opportunities for crew conflict and dysphoria from confinement on long-duration space missions in cramped quarters, in addition to reducing consumable requirements. However, extensive cognitive and psychological testing will need to be performed to determine whether a prolonged sleep strategy truly provides psychological benefit. Furthermore, sleep architecture is known to be altered during low earth orbit missions (Dijk et al., 2001; Koller et al., 2021; Monk et al., 1998) and it is unknown if a sedation-induced prolonged sleep countermeasure strategy affects sleep architecture. We are collecting limited electroencephalographic data during the 5-day protocols, but longer-term monitoring is necessary for more robust assessments of sleep architecture. This highlights a critical knowledge gap to our protocol and any similar countermeasure strategy.
Exercise is presently the main approach to reduce muscle atrophy that occurs due to unloading of the body during low earth orbit space missions. Aerobic exercise is the primary countermeasure for protection from aerobic deconditioning (measured by pre-flight to post-flight changes in maximal oxygen consumption, VO2max). Yet appreciable declines in VO2max and general aerobic conditioning still occur and knowledge gaps regarding optimization of exercise protocols for long-duration space missions remain (Rivas et al., 2023). Additionally, aerobic exercise is metabolically demanding and it is unknown how it might impact the metabolic reduction desired during a quasi-torpor state. Resistance exercise training, which is used to minimize muscle atrophy and preserve muscle strength and quality (Rivas et al., 2023), also attenuates VO2max losses as well as muscle atrophy in ground-based bed rest analogs and during space flight (Ploutz-Snyder et al., 2018; Stremel et al., 1976; Engelke et al., 1998; Greenleaf et al., 1989). Therefore, designing a less metabolically expensive protocol focused on resistance exercise could protect muscle architecture and function as well as reduce the decline in VO2max during long-duration space missions. We developed a specific exercise protocol (Table 1) built around devices with small physical footprints, and we are monitoring the metabolic cost of this protocol during our trials. The flywheel and the isometric ball used in this protocol conform to the space restrictions expected on Artemis and Mars missions. Comparing the muscle-preserving efficacy of this metabolically less-costly protocol to current aerobic protocols will be an important future study.
Appropriate nutrition during long-duration space missions can also help to prevent muscle atrophy and ensure astronauts’ safety and mission success. Inadequate food and nutrition is one of the highest priority risks for the Human Research Program and is currently assessed as a 3x4 LxC risk, meaning that it has a High Likelihood of occurring (>1%), and High Consequences for both mission health and performance (e.g., death, severe reduction in performance), and for long term health (e.g., major impact on quality of life) (NASA Human Research Roadmap) Despite the existence of a standardized diet for moderately active adults (Baba et al., 2020), astronauts experience significant energy deficits resulting in weight loss (Matsumoto et al., 2011) as large as 5 kg per month (Stein et al., 1999), which can lead to reduced protein synthesis and loss of lean mass. This energy deficit is mainly caused by two factors: astronauts do not have a sufficient caloric intake (Stein and Blanc, 2011; Heer et al., 2000) and in-flight exercise countermeasures significantly increase total energy expenditure (Bergouignan et al., 2016). As plans develop for longer-term space missions, increased research is needed to optimize health and performance of astronauts and reduce health risks. (Smith et al., 2005; Smith and Zwart, 2021; Tang et al., 2021; NASA, 2020; Smith et al., 2021) Given that the loss of lean tissue often seen with space missions is associated with detriments to multiple organ systems (Smith et al., 2005; Smith and Zwart, 2021), it is critical that lean tissue be preserved as much as possible. Doing so requires that the exercise performed during space missions is fueled by ample energy and provision of the necessary macro- and micro-nutrients (Smith and Zwart, 2021). In addition to the recognized demands of long-duration space missions, the interaction of a quasi-torpor state with muscle mass and nutritional status remains unknown. For our Phase 1 and Phase 2 experiments we did not mandate a pre-study or on-study diet. However, for Phase 3 we are implementing a specific diet regimen designed to minimize glucose shifts and muscle catabolism during the prolonged sleep period while maximizing energy availability during the short awake period. Future studies will need to evaluate in more depth what the optimal timing and composition of nutrients is to support resting metabolic rate reduction and lessen muscle disuse atrophy, bone resorption, and other untoward catabolic events.
We have extensive clinical and laboratory experience with light to moderate external cooling (Moore et al., 2008; Sonder et al., 2018; Callaway et al., 2020; Callaway et al., 2015; Rittenberger et al., 2019; Flickinger et al., 2024; Hostler et al., 2009; Rittenberger et al., 2021). External cooling used in clinical applications, such as after cardiac arrest, reduces many physiologic processes and our hypothesis for this experimental series was that sedation plus active light external cooling would be synergistic and compounding to reduce overall metabolism. Our experiments to date have found that central body temperature slowly falls after sedation and causes a reduction in metabolism (Callaway et al., 2024). This is consistent with a loss of total body heat content after a reduction in heat production (Elmer and Callaway, 2023). An important observation from our trials is that we do not require very cold external cooling; contact of skin with circulating water at 20°C-25°C will remove enough heat to facilitate a 1-2°C decrease in core body temperature from baseline without causing discomfort (Callaway et al., 2024). It remains to be seen if there is a synergistic effect of hypothermia and sedation on metabolic reduction.
Several major areas require further investigation. Determining the optimal exercise strategy (including exercise type, timing, duration, and frequency of training) to minimize muscle atrophy and loss of strength incurred during both the quasi-torpor protocol and long-duration space missions is critical to ensure crew safety and wellbeing. The exercise strategy also needs to balance the metabolic cost of exercise with maintenance of muscle volume and strength. Equally as important, defining the optimal nutrition strategy (including meal composition, micronutrients and supplements, and timing of ingestion of proteins, carbohydrates, fats, and fluids) to reduce muscle catabolism and promote lean muscle mass maintenance, or even enhance muscle protein anabolism, is vital to success of the quasi-torpor strategy. We also need to understand the physiological and psychological effects of repeated bouts of prolonged sleep over a longer period, such as several weeks or months. Additionally, we need to evaluate the physiological effects of prolonged sleep and metabolic reduction in microgravity, including whether the quasi-torpor protocol reduces susceptibility to the effects of radiation or microgravity. Other physiologic effects also require scrutiny, such as the short and long-term consequences of prolonged sleep and dexmedetomidine on both in-protocol and post-protocol sleep and sleep architecture; and the safety, tolerance, withdrawal, and possible addiction profile for long-term dexmedetomidine administration. Microgravity and radiation may additionally affect the shelf-life of any drug, pharmacologic testing of any candidate drug will be necessary to ensure fidelity during long-duration space missions. For example, the terrestrial shelf-life of dexmedetomidine is 2 years for undiluted unopened vials, while the diluted shelf-life is 48-h at room temperature or 14-day at refrigerated temperatures; the current drug stability would not suffice for a round-trip to Mars (Marquis et al., 2017). Finally, many logistical hurdles must be resolved, including but not limited to whether astronauts can safely self-administer this protocol routinely, and if astronauts can perform emergency or mission critical tasks during and following this protocol.
AW: Conceptualization, Investigation, Methodology, Writing–original draft, Writing–review and editing. KF: Conceptualization, Investigation, Methodology, Writing–review and editing. VW: Investigation, Writing–review and editing. RD: Investigation, Writing–review and editing. AJ: Investigation, Writing–review and editing. PP: Investigation, Writing–review and editing. JM: Investigation, Writing–review and editing. EZ: Investigation, Writing–review and editing. FG: Conceptualization, Investigation, Methodology, Writing–review and editing. BG: Investigation, Methodology, Writing–review and editing. MM: Investigation, Methodology, Writing–review and editing. KM: Investigation, Methodology, Writing–review and editing. DB: Methodology, Writing–review and editing. PE: Methodology, Writing–review and editing. CC: Conceptualization, Funding acquisition, Investigation, Methodology, Writing–review and editing.
The author(s) declare that financial support was received for the research, authorship, and/or publication of this article. This study was funded by the Translational Research Institute for Space Health (TRISH) and the National Aeronautics and Space Administration (NASA) under a cooperative agreement (NNX16AO69A). Dr. Mortreux received funding from NASA EPSCoR RI 80NSSC22M0040 for work related to this study.
The authors declare that the research was conducted in the absence of any commercial or financial relationships that could be construed as a potential conflict of interest.
All claims expressed in this article are solely those of the authors and do not necessarily represent those of their affiliated organizations, or those of the publisher, the editors and the reviewers. Any product that may be evaluated in this article, or claim that may be made by its manufacturer, is not guaranteed or endorsed by the publisher.
Baba, S., Smith, T., Hellmann, J., Bhatnagar, A., Carter, K., Vanhoover, A., et al. (2020). Space flight diet-induced deficiency and response to gravity-free resistive exercise. Nutrients 12 (8), 2400. doi:10.3390/nu12082400
Barger, L. K., Flynn-Evans, E. E., Kubey, A., Walsh, L., Ronda, J. M., Wang, W., et al. (2014). Prevalence of sleep deficiency and use of hypnotic drugs in astronauts before, during, and after spaceflight: an observational study. Lancet Neurol. 13 (9), 904–912. doi:10.1016/s1474-4422(14)70122-x
Basner, M., Savitt, A., Moore, T. M., Port, A. M., McGuire, S., Ecker, A. J., et al. (2015). Development and validation of the cognition test battery for spaceflight. Aerosp. Med. Hum. Perform. 86 (11), 942–952. doi:10.3357/amhp.4343.2015
Bergouignan, A., Stein, T. P., Habold, C., Coxam, V., O’ Gorman, D., and Blanc, S. (2016). Towards human exploration of space: the THESEUS review series on nutrition and metabolism research priorities. NPJ Microgravity 2, 16029. doi:10.1038/npjmgrav.2016.29
Blanco, M. B., Greene, L. K., Schopler, R., Williams, C. V., Lynch, D., Browning, J., et al. (2021). On the modulation and maintenance of hibernation in captive dwarf lemurs. Sci. Rep. 11 (1), 5740. doi:10.1038/s41598-021-84727-3
Callaway, C. W., Coppler, P. J., Faro, J., Puyana, J. S., Solanki, P., Dezfulian, C., et al. (2020). Association of initial illness severity and outcomes after cardiac arrest with targeted temperature management at 36 °C or 33 °C. JAMA Netw. Open 3 (7), e208215. doi:10.1001/jamanetworkopen.2020.8215
Callaway, C. W., Elmer, J., Guyette, F. X., Molyneaux, B. J., Anderson, K. B., Empey, P. E., et al. (2015). Dexmedetomidine reduces shivering during mild hypothermia in waking subjects. PLoS ONE 10 (8), e0129709. doi:10.1371/journal.pone.0129709
Callaway, C. W., Flickinger, K. L., Weissman, A., Guyette, F. X., DeMaio, R., Jonsson, A., et al. (2024). Alpha-2-adrenergic agonists reduce resting energy expenditure in humans during external cooling. Temperature 11, 280–298. doi:10.1080/23328940.2024.2339781
Choukér, A., Ngo-Anh, T. J., Biesbroek, R., Heldmaier, G., Heppener, M., and Bereiter-Hahn, J. (2021). European space agency’s hibernation (torpor) strategy for deep space missions: linking biology to engineering. Neurosci. Biobehav Rev. 131, 618–626. doi:10.1016/j.neubiorev.2021.09.054
de, V., and Weir, J. B. (1948). New methods for calculating metabolic rate with special reference to protein metabolism. J. Physiol. (Lond). 109 (1–2), 1–9.
Dijk, D. J., Neri, D. F., Wyatt, J. K., Ronda, J. M., Riel, E., Ritz-De Cecco, A., et al. (2001). Sleep, performance, circadian rhythms, and light-dark cycles during two space shuttle flights. Am. J. Physiol. Regul. Integr. Comp. Physiol. 281 (5), R1647–R1664. doi:10.1152/ajpregu.2001.281.5.r1647
Drew, K. L., Frare, C., and Rice, S. A. (2017). Neural signaling metabolites may modulate energy use in hibernation. Neurochem. Res. 42 (1), 141–150. doi:10.1007/s11064-016-2109-4
Drug Enforcement Administration (2022). Xylazine. Drug Enforc. Adm. Divers. Control Div. Drug and Chem. Eval. Sect., Available at: https://www.deadiversion.usdoj.gov/drug_chem_info/Xylazine.pdf.
Elmer, J., and Callaway, C. W. (2023). Temperature control after cardiac arrest. Resuscitation 189, 109882. doi:10.1016/j.resuscitation.2023.109882
Engelke, K. A., Convertino, V. A., Doerr, D. F., and Maples, A. B. (1998). Restoration of peak vascular conductance after simulated microgravity by maximal exercise. Clin. Physiol. 18 (6), 544–553. doi:10.1046/j.1365-2281.1998.00133.x
Flickinger, K. L., Weissman, A., Elmer, J., Coppler, P. J., Guyette, F. X., Repine, M. J., et al. (2024). Metabolic manipulation and therapeutic hypothermia. Ther. Hypothermia Temp. Manag. 14 (1), 46–51. doi:10.1089/ther.2023.0010
Food and Agriculture Organization of the United Nations (2004). Human energy requirements: report of a joint FAO/WHO/UNU expert consultation, Rome, 17-24 october 2001 (FAO food and nutrition technical report series). Rome: FAO.
Frare, C., and Drew, K. L. (2021). Seasonal changes in adenosine kinase in tanycytes of the Arctic ground squirrel (Urocitellus parryii). J. Chem. Neuroanat. 113, 101920. doi:10.1016/j.jchemneu.2021.101920
Giovannitti, J. A., Thoms, S. M., and Crawford, J. J. (2015). Alpha-2 adrenergic receptor agonists: a review of current clinical applications. Anesth. Prog. 62 (1), 31–38. doi:10.2344/0003-3006-62.1.31
Gomes, C. A. R., Andriolo, R. B., Bennett, C., Lustosa, S. A. S., Matos, D., Waisberg, D. R., et al. (2015). Percutaneous endoscopic gastrostomy versus nasogastric tube feeding for adults with swallowing disturbances. Cochrane Database Syst. Rev. 2015 (5), CD008096. doi:10.1002/14651858.CD008096.pub4
Grabek, K. R., Cooke, T. F., Epperson, L. E., Spees, K. K., Cabral, G. F., Sutton, S. C., et al. (2019). Genetic variation drives seasonal onset of hibernation in the 13-lined ground squirrel. Commun. Biol. 2, 478. doi:10.1038/s42003-019-0719-5
Greenleaf, J. E., Bernauer, E. M., Ertl, A. C., Trowbridge, T. S., and Wade, C. E. (1989). Work capacity during 30 days of bed rest with isotonic and isokinetic exercise training. J. Appl. Physiol. 67 (5), 1820–1826. doi:10.1152/jappl.1989.67.5.1820
Griffin, C. E., Kaye, A. M., Bueno, F. R., and Kaye, A. D. (2013). Benzodiazepine pharmacology and central nervous system-mediated effects. Ochsner J. 13 (2), 214–223.
Grimaldi, D., Silvani, A., Benarroch, E. E., and Cortelli, P. (2014). Orexin/hypocretin system and autonomic control: new insights and clinical correlations. Neurology, 82.
Heer, M., Boerger, A., Kamps, N., Mika, C., Korr, C., and Drummer, C. (2000). Nutrient supply during recent European missions. Pflugers Arch. 441 (2-3 Suppl. l), R8–R14. doi:10.1007/s004240000334
Herring, W. J., Snyder, E., Budd, K., Hutzelmann, J., Snavely, D., Liu, K., et al. (2012). Orexin receptor antagonism for treatment of insomnia: a randomized clinical trial of suvorexant. Neurology 79 (23), 2265–2274. doi:10.1212/wnl.0b013e31827688ee
Hostler, D., Northington, W. E., and Callaway, C. W. (2009). High-dose diazepam facilitates core cooling during cold saline infusion in healthy volunteers. Appl. Physiol. Nutr. Metab. 34 (4), 582–586. doi:10.1139/h09-011
Jetté, M., Sidney, K., and Blümchen, G. (1990). Metabolic equivalents (METS) in exercise testing, exercise prescription, and evaluation of functional capacity. Clin. Cardiol. 13 (8), 555–565. doi:10.1002/clc.4960130809
Jinka, T. R., Tøien, Ø., and Drew, K. L. (2011). Season primes the brain in an arctic hibernator to facilitate entrance into torpor mediated by adenosine A(1) receptors. J. Neurosci. 31 (30), 10752–10758. doi:10.1523/jneurosci.1240-11.2011
Jones, C. W., Basner, M., Mollicone, D. J., Mott, C. M., and Dinges, D. F. (2022). Sleep deficiency in spaceflight is associated with degraded neurobehavioral functions and elevated stress in astronauts on six-month missions aboard the International Space Station. Sleep 45 (3), zsac006. doi:10.1093/sleep/zsac006
Junkins, M. S., Bagriantsev, S. N., and Gracheva, E. O. (2022). Towards understanding the neural origins of hibernation. J. Exp. Biol. 225 (1), jeb229542. doi:10.1242/jeb.229542
Kales, A., Bixler, E. O., and Kales, J. D. Effects of hypnotic drugs on performance before and after sleep. NTRS - NASA technical Reports server; 1975. Report No.: NASA-CR-143311.
Kamibayashi, T., Maze, M., Weiskopf, R., Weiskopf, R., and Todd, M. (2000). Clinical uses of a2-adrenergic agonists. Anesthesiology 93 (5), 1345–1349. doi:10.1097/00000542-200011000-00030
Koller, D. P., Kasanin, V., Flynn-Evans, E. E., Sullivan, J. P., Dijk, D.-J., Czeisler, C. A., et al. (2021). Altered sleep spindles and slow waves during space shuttle missions. NPJ Microgravity 7 (1), 48. doi:10.1038/s41526-021-00177-1
Kurz, A., Sessler, D. I., Annadata, R., Dechert, M., Christensen, R., and Bjorksten, A. R. (1995). Midazolam minimally impairs thermoregulatory control. Anesth. Analg. 81 (2), 393–398. doi:10.1097/00000539-199508000-00032
Marquis, K., Hohlfelder, B., and Szumita, P. M. (2017). Stability of dexmedetomidine in 0.9% sodium chloride in two types of intravenous infusion bags. Int. J. Pharm. Compd. 21 (5), 436–439.
Matsumoto, A., Storch, K. J., Stolfi, A., Mohler, S. R., Frey, M. A., and Stein, T. P. (2011). Weight loss in humans in space. Aviat. Space Environ. Med. 82 (6), 615–621. doi:10.3357/asem.2792.2011
McManus, C., Mifflin, N., Rivera, R., Vause, S., Tran, T., Ostroff, M., et al. (2024). Comparison of outcomes from tunnelled femorally inserted central catheters and peripherally inserted central catheters: a propensity score-matched cohort study. BMJ Open 14 (5), e081749. doi:10.1136/bmjopen-2023-081749
Michenfelder, J. D., and Milde, J. H. (1991). The relationship among canine brain temperature, metabolism, and function during hypothermia. Anesthesiology 75, 130–136. doi:10.1097/00000542-199107000-00021
Monk, T. H., Buysse, D. J., Billy, B. D., Kennedy, K. S., and Willrich, L. M. (1998). Sleep and circadian rhythms in four orbiting astronauts. J. Biol. Rhythms 13 (3), 188–201. doi:10.1177/074873098129000039
Monti, J. M., and Monti, D. (2015). “Non-benzodiazepine hypnotics,” in Encyclopedia of psychopharmacology. Editors I. P. Stolerman,, and L. H. Price, 2nd ed. (Heidelberg: Springer Berlin), 1134–1138. doi:10.1007/978-3-642-36172-2
Moore, T. M., Callaway, C. W., and Hostler, D. (2008). Core temperature cooling in healthy volunteers after rapid intravenous infusion of cold and room temperature saline solution. Ann. Emerg. Med. 51 (2), 153–159. doi:10.1016/j.annemergmed.2007.07.012
Mortreux, M., Ko, F. C., Riveros, D., Bouxsein, M. L., and Rutkove, S. B., Longitudinal time course of muscle impairments during partial weight-bearing in rats, NPJ Microgravity, 5:20, doi:10.1038/s41526-019-0080-5
Mortreux, M., Nagy, J. A., Ko, F. C., Bouxsein, M. L., and Rutkove, S. B. (2018). A novel partial gravity ground-based analog for rats via quadrupedal unloading. J. Appl. Physiol. 125 (1), 175–182. doi:10.1152/japplphysiol.01083.2017
Mortreux, M., Riveros, D., Bouxsein, M. L., and Rutkove, S. B. (2019a). Mimicking a space mission to mars using hindlimb unloading and partial weight bearing in rats. J. Vis. Exp., 146. doi:10.3791/59327
Mortreux, M., Semple, C., Riveros, D., Nagy, J. A., and Rutkove, S. B. (2019b). Electrical impedance myography for the detection of muscle inflammation induced by λ-carrageenan. PLoS ONE 14 (10), e0223265. doi:10.1371/journal.pone.0223265
Mugahid, D. A., Sengul, T. G., You, X., Wang, Y., Steil, L., Bergmann, N., et al. (2019). Proteomic and transcriptomic changes in hibernating grizzly bears reveal metabolic and signaling pathways that protect against muscle atrophy. Sci. Rep. 9 (1), 19976. doi:10.1038/s41598-019-56007-8
NASA Mars transportation. White papers; NRTS document ID: 20240000449. 2022. Available at: https://www.nasa.gov/wp-content/uploads/2023/10/acr22-wp-mars-transportation.pdf?emrc=1a6c54.
NASA. NASA’s Moon to mars architecture: a summary of the 2022 architecture concept review process and results - april 2023. NRTS Doc. ID 20230002706. 2023. Available at: https://www.nasa.gov/sites/default/files/atoms/files/m2m-architecture-executive-summary.pdf.
NASA Human Research Roadmap NASA human research Roadmap. Available at: https://humanresearchroadmap.nasa.gov/Risks/risk.aspx?i=176#.
NASA, (2020). NASA-STD-3001 technical brief food and nutrition. NASA office of the chief health and medical officer, 1–8. Report No.: V2 6029-6031, 6035, 6039-6043, 7001-7015.
Ploutz-Snyder, L. L., Downs, M., Goetchius, E., Crowell, B., English, K. L., Ploutz-Snyder, R., et al. (2018). Exercise training mitigates multisystem deconditioning during bed rest. Med. Sci. Sports Exerc 50 (9), 1920–1928. doi:10.1249/mss.0000000000001618
Rittenberger, J. C., Flickinger, K. L., Weissman, A., Repine, M., Elmer, J., Guyette, F. X., et al. (2019). Cooling to facilitate metabolic suppression in healthy individuals. Aerosp. Med. Hum. Perform. 90 (5), 475–479. doi:10.3357/amhp.5284.2019
Rittenberger, J. C., Weissman, A., Flickinger, K. L., Guyette, F. X., Hopkins, D., Repine, M. J., et al. (2021). Glycopyrrolate does not ameliorate hypothermia associated bradycardia in healthy individuals: a randomized crossover trial. Resuscitation 164, 79–83. doi:10.1016/j.resuscitation.2021.05.020
Rivas, E., Strock, N., Dillon, E. L., and Frisco, D. (2023). Risk of impaired performance due to reduced muscle mass, strength, and endurance and risk of reduced physical performance capabilities due to reduced aerobic capacity. Available at: https://ntrs.nasa.gov/citations/20220019338.
Rolle, A., De Jong, A., Vidal, E., Molinari, N., Azoulay, E., and Jaber, S. (2022). Cardiac arrest and complications during non-invasive ventilation: a systematic review and meta-analysis with meta-regression. Intensive Care Med. 48 (11), 1513–1524. doi:10.1007/s00134-022-06821-y
Ruf, T., and Geiser, F. (2015). Daily torpor and hibernation in birds and mammals. Biol. Rev. Camb Philos. Soc. 90 (3), 891–926. doi:10.1111/brv.12137
Rutkove, S. B., and Sanchez, B. (2019). Electrical impedance methods in neuromuscular assessment: an overview. Cold Spring Harb. Perspect. Med. 9 (10), a034405. doi:10.1101/cshperspect.a034405
Sanchez, B., and Rutkove, S. B. (2017). Present uses, future applications, and technical underpinnings of electrical impedance myography. Curr. Neurol. Neurosci. Rep. 17 (11), 86. doi:10.1007/s11910-017-0793-3
Scammell, T. E., and Winrow, C. J. (2011). Orexin receptors: pharmacology and therapeutic opportunities. Annu. Rev. Pharmacol. Toxicol. 51, 243–266. doi:10.1146/annurev-pharmtox-010510-100528
Semple, C., Riveros, D., Sung, D.-M., Nagy, J. A., Rutkove, S. B., and Mortreux, M. (2020). Using electrical impedance myography as a biomarker of muscle deconditioning in rats exposed to micro- and partial-gravity analogs. Front. Physiol. 11, 557796. doi:10.3389/fphys.2020.557796
Silvani, A., Cerri, M., Zoccoli, G., and Swoap, S. J. (2018). Is adenosine action common ground for NREM sleep, torpor, and other hypometabolic states? Physiol. (Bethesda) 33 (3), 182–196. doi:10.1152/physiol.00007.2018
Skibiski, J., Patel, P., and Abdijadid, S. (2024). Barbiturates. NCBI Bookshelf Stat. Pearls. Available at: https://www.ncbi.nlm.nih.gov/books/NBK539731/.
Smith, S. M. Nutritional requirements for exploration missions up to 365 days. NASA NTRS Tech. Rep. Serv. 2020 Apr. Rep. No. JSC-67378, 1–10. Available at: https://ntrs.nasa.gov/citations/20205008306.
Smith, S. M., and Zwart, S. R. (2021). Nutrition as fuel for human spaceflight. Physiol. (Bethesda) 36 (5), 324–330. doi:10.1152/physiol.00011.2021
Smith, S. M., Zwart, S. R., Block, G., Rice, B. L., and Davis-Street, J. E. (2005). The nutritional status of astronauts is altered after long-term space flight aboard the International Space Station. J. Nutr. 135 (3), 437–443. doi:10.1093/jn/135.3.437
Smith, S. M., Zwart, S. R., Gl, D., and M, H. (2021). Human adaptation toSpaceflight: the role of food and nutrition. NP-2021-03-003-JSC 2nd. Houston, TX: National Aeronautics and Space Administration Lyndon B. Johnson Space Center.
Sonder, P., Janssens, G. N., Beishuizen, A., Henry, C. L., Rittenberger, J. C., Callaway, C. W., et al. (2018). Efficacy of different cooling technologies for therapeutic temperature management: a prospective intervention study. Resuscitation 124, 14–20. doi:10.1016/j.resuscitation.2017.12.026
Stampi, C. (1994). Sleep and circadian rhythms in space. J. Clin. Pharmacol. 34, 518–534. doi:10.1002/j.1552-4604.1994.tb04996.x
Stein, T. P., and Blanc, S. (2011). Does protein supplementation prevent muscle disuse atrophy and loss of strength? Crit. Rev. Food Sci. Nutr. 51 (9), 828–834. doi:10.1080/10408398.2010.482679
Stein, T. P., Leskiw, M. J., Schluter, M. D., Hoyt, R. W., Lane, H. W., Gretebeck, R. E., et al. (1999). Energy expenditure and balance during spaceflight on the space shuttle. Am. J. Physiol. 276 (6 Pt 2), R1739–R1748. doi:10.1152/ajpregu.1999.276.6.r1739
Stremel, R. W., Convertino, V. A., Bernauer, E. M., and Greenleaf, J. E. (1976). Cardiorespiratory deconditioning with static and dynamic leg exercise during bed rest. J. Appl. Physiol. 41 (6), 905–909. doi:10.1152/jappl.1976.41.6.905
Takahashi, H., Nishikawa, T., Mizutani, T., and Handa, F. (1997). Oral clonidine premedication decreases energy expenditure in human volunteers. Can. J. Anaesth. 44 (3), 268–272. doi:10.1007/bf03015364
Takahashi, T., Murayama, R., Abe-Doi, M., Miyahara-Kaneko, M., Kanno, C., Nakamura, M., et al. (2020). Preventing peripheral intravenous catheter failure by reducing mechanical irritation. Sci. Rep. 10 (1), 1550. doi:10.1038/s41598-019-56873-2
Tang, H., Rising, H. H., Majji, M., and Brown, R. D. (2021). Long-term space nutrition: a scoping review. Nutrients 14 (1), 194. doi:10.3390/nu14010194
Tarulli, A. W., Duggal, N., Esper, G. J., Garmirian, L. P., Fogerson, P. M., Lin, C. H., et al. (2009). Electrical impedance myography in the assessment of disuse atrophy. Arch. Phys. Med. Rehabil. 90 (10), 1806–1810. doi:10.1016/j.apmr.2009.04.007
Tøien, Ø., Blake, J., Edgar, D. M., Grahn, D. A., Heller, H. C., and Barnes, B. M. (2011). Hibernation in black bears: independence of metabolic suppression from body temperature. Science 331 (6019), 906–909. doi:10.1126/science.1199435
Tooze, J. A., Schoeller, D. A., Subar, A. F., Kipnis, V., Schatzkin, A., and Troiano, R. P. (2007). Total daily energy expenditure among middle-aged men and women: the OPEN study. Am. J. Clin. Nutr. 86, 382–387. doi:10.1093/ajcn/86.2.382
Trabulsi, J., Troiano, R. P., Subar, A. F., Sharbaugh, C., Kipnis, V., Schatzkin, A., et al. (2003). Precision of the doubly labeled water method in a large-scale application: evaluation of a streamlined-dosing protocol in the Observing Protein and Energy Nutrition (OPEN) study. Eur. J. Clin. Nutr. 57 (11), 1370–1377. doi:10.1038/sj.ejcn.1601698
Will, P. M., and Walter, J. D. (1999). Exercise testing: improving performance with a ramped Bruce protocol. Am. Heart J. 138 (6 Pt 1), 1033–1037. doi:10.1016/s0002-8703(99)70067-0
Wilson, M. E., Dobler, C. C., Morrow, A. S., Beuschel, B., Alsawas, M., Benkhadra, R., et al. (2020). Association of home noninvasive positive pressure ventilation with clinical outcomes in chronic obstructive pulmonary disease: a systematic review and meta-analysis. JAMA 323 (5), 455–465. doi:10.1001/jama.2019.22343
Keywords: long-duration space travel, metabolism, exercise, sedation, cooling
Citation: Weissman AJ, Flickinger KL, Wu V, DeMaio R, Jonsson A, Prescott P, Monteleone J, Zurowski E, Guyette FX, Gordon BDH, Mortreux M, Melanson K, Buysse DJ, Empey PE and Callaway CW (2024) Quasi-torpor for long-duration space missions. Front. Space Technol. 5:1457487. doi: 10.3389/frspt.2024.1457487
Received: 30 June 2024; Accepted: 05 September 2024;
Published: 11 October 2024.
Edited by:
Saswati Das, Atal Bihari Vajpayee Institute of Medical Sciences and Dr. Ram Manohar Lohia Hospital, IndiaReviewed by:
Timo Frett, German Aerospace Center (DLR), GermanyCopyright © 2024 Weissman, Flickinger, Wu, DeMaio, Jonsson, Prescott, Monteleone, Zurowski, Guyette, Gordon, Mortreux, Melanson, Buysse, Empey and Callaway. This is an open-access article distributed under the terms of the Creative Commons Attribution License (CC BY). The use, distribution or reproduction in other forums is permitted, provided the original author(s) and the copyright owner(s) are credited and that the original publication in this journal is cited, in accordance with accepted academic practice. No use, distribution or reproduction is permitted which does not comply with these terms.
*Correspondence: Alexandra J. Weissman, d2Vpc3NtYW5hakB1cG1jLmVkdQ==
Disclaimer: All claims expressed in this article are solely those of the authors and do not necessarily represent those of their affiliated organizations, or those of the publisher, the editors and the reviewers. Any product that may be evaluated in this article or claim that may be made by its manufacturer is not guaranteed or endorsed by the publisher.
Research integrity at Frontiers
Learn more about the work of our research integrity team to safeguard the quality of each article we publish.