- 1Carl Sagan Center, SETI Institute, Mountain View, CA, United States
- 2Department of Biology, University of Florida, Gainesville, FL, United States
- 3National Aeronautics and Space Administration (NASA), Kennedy Space Center, Merritt Island, FL, United States
- 4Jacobs Technology, COMET Contract, Kennedy Space Center, Merritt Island, FL, United States
- 5AETOS Systems Inc, Huntsville, AL, United States
Terrestrial plants from the very limits of life are likely to harbor genes that confer an advantage in human space exploration. These plants are seemingly capable of performing mission critical functions in spaceflight and on extraterrestrial farms while informing directed gene manipulation in target plant species. However, their adaptations to physiologically extreme habitats may hinder the efficacy of routine laboratory techniques for model plants. We here present the development of Antarctic moss Ceratodon purpureus payload and flight operations for the ANT1 Radiation Tolerance Experiment with Moss in Orbit on the Space Station (ARTEMOSS) experiment to the International Space Station (ISS) given limited physical space and crew time. We demonstrate that the hydrophobic surface of Antarctic moss impedes chemical tissue fixation and precludes the use of RNAlater coupled with payload hardware deployed in standard plant spaceflight experiments. We show that deep-freezing the moss tissue on Petri plates provides adequate tissue fixation and allows the extraction of high-quality RNA suitable for gene expression profiling. We replaced hardware with stacks of Petri plates housing Antarctic moss and chemical fixation with deep-freezing in a cryogenic GLACIER freezer. Our design can be translated to other plant species to expand current experimentation techniques with plants from extreme terrestrial environments in order to advance human space exploration.
1 Introduction
Plants form a critical component of long-term space exploration, providing a number of services beyond a sustainable food source. For instance, they can capture carbon dioxide, release oxygen, revitalize the atmosphere, and enrich extraterrestrial regolith. An important challenge for space exploration is identifying genetic variants that make plants capable of performing these mission-critical functions under prevailing deep space conditions. Such plant genotypes could then be directly incorporated into human space missions while the genes governing their resilience can be introduced into target plant species. The most extreme terrestrial habitats are the only environments on Earth that feature some conditions analogous to those present on the Moon or Mars. For instance, ferns, gymnosperms, and flowering plants in the high Atacama Desert experience near Martian aridity, and moss inhabiting the volcano Licancabur in the tropical Andes receives ultraviolet B (UVB) irradiance approaching that on the Martian surface (Cabrol et al., 2014; Häder and Cabrol, 2020). Hundreds of species of bryophytes, algae, and two flowering plant species dwelling in the Antarctic cold desert experience dryness, temperature ranges, and, during the seasonal ozone hole, elevated UVB levels quite similar to those on Mars (Colesie et al., 2023). Plants from these extreme environments have evolved adaptations to sustain life under such harsh conditions, thus are likely to harbor genes that confer an advantage in human space exploration. These plants, however, differ considerably from model plants prevalent in space biology research and are likely to require alternative experimental techniques.
The waxy cuticle coating aerial organs forms an effective barrier between plant cells and the external environment, and its occurrence some 400 million years ago in bryophyte ancestors has been considered one of the greatest adaptations of plants that allowed them to establish and conquer dry land (Riederer and Schreiber, 2001; Domínguez, Heredia-Guerrero, and Heredia, 2015; Hõrak, 2017; Renault et al., 2017; Xue et al., 2017; Ziv et al., 2018). At present, waxy cuticles alongside tiny leaves, spines, taproots, or water-storage tissues continue to be instrumental to plant survival in the harshest places on Earth. Cuticular wax is typically a complex mixture of dozens of compounds with diverse long hydrocarbon chains (C20–C37) or ring structures that consist of fully saturated fatty acid derivatives (Yeats and Rose, 2013). Some waxes are epicuticular and form an outermost coating at the tissue/air interface, while others are superimposed and integrated within a three-dimensional network of cutin biopolymer (Buschhaus and Jetter, 2011). A high content of cuticular waxes that are non-polar result in a plant surface that is hydrophobic or super-hydrophobic. Surface hydrophobicity influences the wettability, self-cleaning behavior, and light reflection at the cuticle interface (Koch and Ensikat, 2008). Hydrophobic surfaces help plants conserve water by reducing the amount lost through evaporation and thus are instrumental to the drought-resistance of plants inhabiting arid climates (Tomasi and Abdel-Haleem, 2023). Hydrophobic surfaces also protect plants from water-related damage such as fungal growth or ice formation on leaves, giving an advantage to plants in extremely cold environments.
Molecular analysis of plant responses to the spaceflight environment typically occurs post-flight when scientific payload is returned to Earth. To adequately preserve molecular processes relevant to plant physiological adaptation to spaceflight, plant tissue needs to be fixed in-flight. To be effective, intracellular fixation must rapidly halt enzymatic reactions without disturbing or modifying cell physiology in the spaceflight-adapted state. Immersing plant tissue in a concentrated aqueous solution of ammonium and cesium sulfates known as RNAlater is currently the most popular method of in-flight plant fixation (Ferl et al., 2011; Kruse et al., 2017; Manzano et al., 2020). To achieve proper fixation, RNAlater needs to be absorbed to the plant surface and translocated into the cells. However, two phenomena that occur at the tissue/air surface and tissue/liquid interface affect RNAlater interactions with plant surfaces that are hydrophobic. First, there is a tendency for plants to trap air and retain thin air layers known as gas films when air makes initial contact with hydrophobic plant surfaces that are then submersed in any polar liquid (Figure 1). Air bubbles are hydrophobic due to the physical properties of nitrogen and oxygen molecules, which are non-polar and have no charge and therefore do not form hydrogen bonds with water molecules but have high affinity for hydrophobic cuticular waxes. The air bubbles present a physical obstacle to tissue–RNAlater contact. Second, the asymmetric sharing of electrons between oxygen and hydrogen atoms in water molecules results in high surface tension and droplet formation (Figure 1). Consequently, aqueous solutions such as RNAlater on hydrophobic surfaces coalesce instead of evenly spreading, which impedes RNAlater adherence (Nairn et al., 2016). It has been shown that hydrophobic plant surfaces have low wettability reflected in water contact angles above 90° and limited ability to retain fluid droplets (Brewer and Nuñez, 2007; Aryal and Neuner, 2010; Aryal and Neuner, 2016). In addition to contact interactions at the surface, RNAlater absorption through the hydrophobic surface can be further limited by low cuticle permeability (Figure 1). Permeability is governed by diffusivity associated with the size of RNAlater molecules in relation to the plant cuticle structure; for cuticles with rich and tightly arranged waxy compounds, diffusivity remains low. Finally, cuticles rich in waxes exhibit low solubility resulting from low affinity of RNAlater molecules for the waxy compounds (Figure 1) (Khayet and Fernández, 2012).
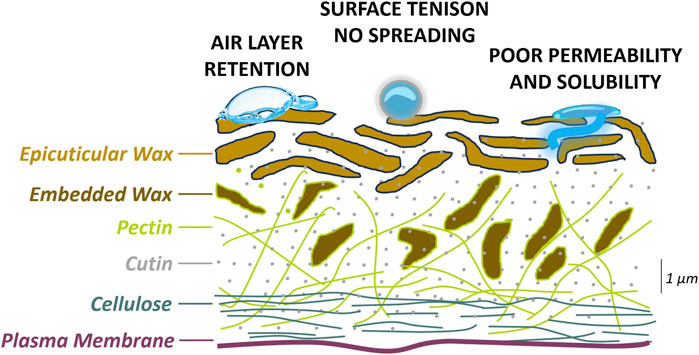
Figure 1. Graphical presentation of the cross section of the plant hydrophobic surface and the causes of poor cell fixation with a polar fixative..
Freezing is another effective method that can be considered for in-flight tissue fixation. Since freezing-based fixation is a physical and not a chemical process, it is suitable for any plant irrespective of its structure or chemical composition and thus can be employed for plants with hydrophobic surfaces. To avoid triggering cold responses and changes to gene expression, plant tissue needs to be frozen very quickly (Kidokoro et al., 2022). Snap-freezing in liquid nitrogen is the fastest tissue freezing technique that has proven successful in numerous biological investigations; however, it cannot be performed on the International Space Station (ISS). Additionally, rapid freezing causes intracellular water molecules to transition from amorphous fluid to a rigid lattice, while salts in cell fluids become concentrated at toxic levels. Ice crystals can tear cellular structures, and disruption of lysosomes leads to release of RNases. Thus, tissues intended for molecular analysis need to remain deep-frozen until processed.
The moss Ceratodon purpureus has a long history as a model for the study of gravitropism, and the species has been successfully deployed in multiple spaceflight experiments to the ISS (Sack, 1993; Sievers et al., 1996; Kern and Sack, 2001; Kern et al., 2005; Cove and Quatrano, 2006). The moss isolates used in these studies originated from central Europe. However, the species has a very broad distribution, spanning all seven continents (McDaniel and Shaw, 2005) and occupies polar and high mountain habitats characterized by extreme cold, drought, low atmospheric pressure, and considerable UVB radiation (McDaniel and Shaw, 2005; Clarke and Robinson, 2008a). Moreover, Ceratodon is found in regions with substantial background ionizing radiation of geological origin and is among one of the first plant colonizers of exclusion zones post nuclear fallout containing toxic levels of radionuclides (Kovalchuk et al., 2004; Mousseau and Møller, 2020). The species is tolerant of both chemical and UV mutagenesis, and the annotated genome of C. purpureus provides a critical resource for transcriptomic analyses of responses to environmental challenges (Thornton et al., 2005). Moreover, C. purpureus undergoes efficient gene-targeting knock-outs and knock-ins using CRISPR-based approaches (Tavernier et al., 2023).
Here, we present the design of plant payload and fixation procedures developed for the spaceflight investigation ARTEMOSS (ANT1 Radiation Tolerance Experiment with Moss in Orbit on the Space Station). ARTEMOSS deploys the moss C. purpureus isolate ANT1, which was collected in Antarctica—the most extreme of terrestrial environments, characterized by severe aridity, cold, and high seasonal UVB fluxes. ARTEMOSS addresses growth, development, and physiology, as reflected by gene expression, of ANT1 in the combined environments of ground-based simulated deep-space ionizing radiation and spaceflight microgravity and requires moss tissue fixation in-flight. We thus present approaches to improve fixation in RNAlater of Antarctic moss with hydrophobic surfaces, along with extensive testing of deep-freeze fixation tailored for the microgravity environment.
2 Materials and methods
2.1 Moss culture
C. purpureus isolate ANT1 was collected at Casey Base field station in Antarctica (a generous gift from Dr. Sharon Robinson, University of Wollongong, New South Wales, Australia), and genetically identical gametophyte laboratory cultures were established (Cove et al., 2009a). ANT1 gametophyte tissue was cultured on 90-mm-diameter round Petri plates with agar BCDA media, which is BCD media supplemented with 5 mM diammonium tartrate and containing 0.7% agar (A9799, plant cell culture agar; Sigma-Aldrich, St. Louis, Missouri, USA) following Cove et al. (2009b). Moss cultures were grown in a growth chamber at 23°C with a 18 hL:6 hD light regime and a white light intensity of 15 W. The 14-day-old ANT1 moss tissue was harvested from two 90-mm-diameter round Petri plates and homogenized in 5 mL of sterile distilled water using tissue blender (PowerGen 125, Fisher Scientific). Thirteen spot-inoculums of 15 µL volume were deposited per 60-mm-diameter round Petri plate with precisely 5.7 mL of agar BCDA supplemented with 0.5% sucrose (Fisher Scientific). Plates were sealed with two layers of micropore tape and placed on a tray in a growth chamber set at 23°C with an 18 hL:6 hD light regime and a white light intensity of 15 W. After 7–10 days of growth, the spot-inoculum Petri plates were used for fixation.
2.2 Chemical fixation of moss tissue
Moss tissue on the Petri plates was fixed by dispensing 18 mL of RNAlater (Invitrogen) or RNAlater mixed with surfactants or adjuvants per Petri plate (Table 1). In each case, the fixative was dispensed very slowly with a serological pipette placed at the edge of the Petri plate to partially recreate the fixative flow in the microgravity environment where hydrostatic pressure does not govern fluid behavior. Moss tissue on the Petri plate was fixed at room temperature for 24 h, and then the Petri plate was frozen at −80°C for 24 h.
2.3 Deep-freeze fixation of moss tissue
Moss tissue on the Petri plates was immersed in liquid nitrogen for 2 min or inserted into a −150°C or −80°C freezer for 24 h.
2.4 Thermocouple freezing test
A small diameter opening was poked through the side of a Petri plate, and a thermocouple sensor was inserted along media into the Petri plate center and secured in place with tape. The Petri plates with thermocouple sensors were inserted into a −150°C or −80°C freezer. Some Petri plates were distributed individually in a horizontal orientation, while others were arranged in five-plate stacks and placed on the side and either in direct contact with the metal freezer shelf or with a layer of Styrofoam to simulate no contact with the freezer metal shelf in microgravity. Measurements were taken every 10 s for 6,460 s (1 h 47 min 40 s).
2.5 RNA extractions
The initial step of RNA extraction differed depending on the fixation method of the moss tissue. On extraction day, the Petri plate with chemically fixed moss tissue was fully thawed, and four spot-inoculums were harvested with forceps, rinsed in RNAlater for 2–3 min, and then transferred to 470 µL of RLT (RNA lysis buffer) with 1% beta-mercaptoethanol (Fisher Scientific). On extraction day, the Petri plate with deep-frozen tissue was removed from the freezer and placed on dry ice. While kept fully frozen, four spot-inoculums were detached from the media with forceps and placed in a pre-chilled 2-mL Eppendorf tube with 470 µL of RLT with 1% beta-mercaptoethanol (Fisher Scientific). For all samples, the tissue was first homogenized using GenoGrinder (SPEX SamplePrep 2010; GenoGrinder) and metal beads at 1,700 rpm for 2 min, followed by homogenization using QIAshredder columns (Qiagen). All the RNA was extracted using a RNeasy Plant Mini kit (Qiagen) and eluted with 30 µL of RNase-free ddH2O. Of 30 µL of eluted RNA, 3 µL was used to measure RNA quantity using Qubit (Qubit 2.0 fluorometer, Invitrogen) and 4 µL was subjected to 2100 Bioanalyzer analysis (Agilent) using plant nano-chip. RIN (RNA integrity number) was calculated using software (Agilent). The electropherograms displayed a data plot of size/migration time versus fluorescence intensity and were also generated using software (Agilent). Total RNA obtained from a minimum of three biological replicas per condition was assayed on the bioanalzyer.
2.6 Imaging
Each Petri plate was photographed from above using a cell phone camera at 30 min and 24 h post-fixation. Moss tissue on Petri plates was also evaluated and photographed under the microscope (Olympus SZX12) at ×7, ×12.5, and ×50 magnification against the dark field (DF).
3 Results
3.1 RNAlater does not effectively fix Antarctic moss tissue
To test the efficacy of a standard chemical fixation method used in plant spaceflight experiments, we subjected Antarctic moss tissue on Petri plates to RNAlater fixation. Submersion of the moss tissue in RNAlater revealed the retention of large air bubbles on the tissue. The air bubbles were not spherical but were, rather, dome-shaped and were reminiscent of a gas film which fully encapsulated each moss colony (Figure 2A). This suggested that the bubbles contained the air adhered to the moss tissue before contact with RNAlater. We observed the retention of similar air bubbles when moss spot-inoculum colonies on a Petri plate were submersed in water (data not shown). Air bubbles did not detach from the moss tissue submersed in RNAlater when the Petri plates were vortexed, rotated, or shaken (data not shown). To assess the efficiency of RNAlater fixation, we extracted total RNA from the moss tissue fixed in RNAlater for 24 h at room temperature, frozen at −80°C for 24 h, and then fully thawed. Extracted total RNA was compared to the reference, total RNA extracted from fresh, never-fixed, or snap-frozen in liquid nitrogen moss tissue. In total RNA obtained from fresh tissue, the 25S rRNA peak dominated and was clearly separated from the 18S rRNA peak (Figure 2B, left). The 25-nt-long RNA corresponding to small RNA contributed a minor fraction of total RNA. The remainder clearly distinguished peaks corresponded to small rRNA. The RIN value was calculated at >7.0 across all biological replicas. In contrast, total RNA extracted from moss tissue fixed in RNAlater showed an accumulation of short RNA molecules and reversed ratios of rRNA peaks with 25S rRNA being the shortest (Figure 2B, right). The RIN value across biological replicas remained at <4.0, corroborating severe RNA degradation. This suggests inefficient fixation of Antarctic moss tissue in RNAlater.
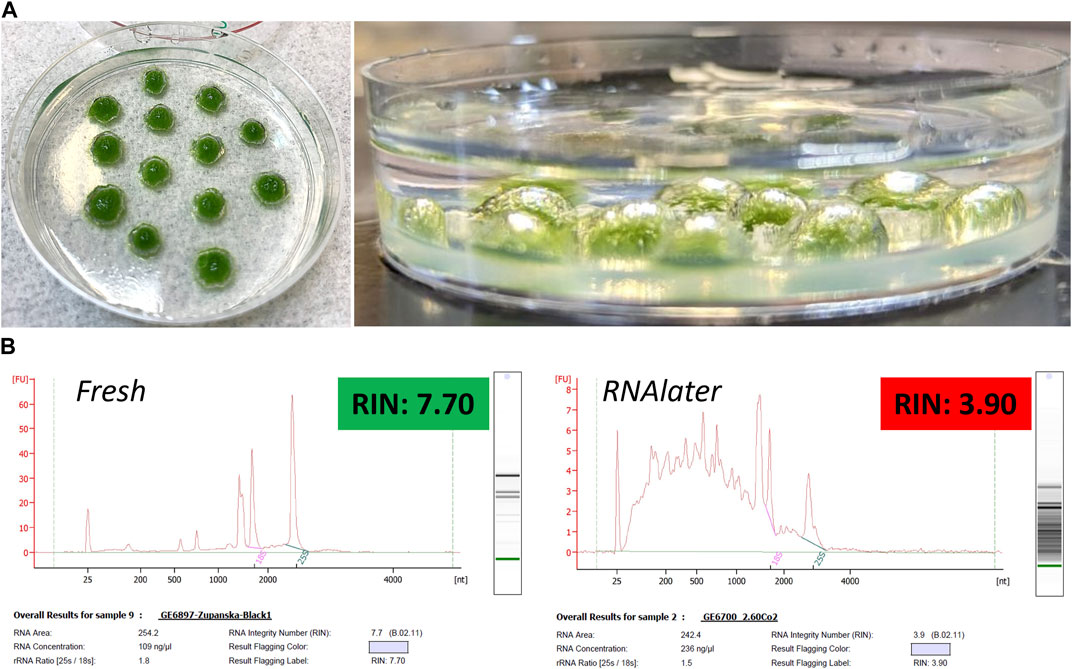
Figure 2. Top panel (A) Photographs of moss ANT1 tissue submersed in RNAlater (left) and of retention of airfilm on the moss tissue (right). Bottom panel (B) Electropherograms of total RNA extracted from fresh, never-fixed, or frozen moss tissue (left) and from moss tissue fixed in RNAlater for 24 h at room temperature, frozen at −80°C for 24 h, and then fully thawed (right).
3.2 Addition of surfactants to RNAlater did not improve fixation of Antarctic moss tissue
Since the moss tissue that retained air bubbles had not been properly fixed in RNAlater, we first focused on increasing the surface area of RNAlater–tissue contact by destabilizing the air bubbles. Air bubbles remain stable in polar liquids due to the force of surface tension; reducing the air bubble surface tension is the main approach to eliminating air bubbles that contact a liquid. Thus, we first deployed surfactants that are well known to affect surface tension between two immiscible phases such as at the boundary between a gaseous phase (air bubble) and condensed phase (RNAlater). Surfactants contain both a hydrophilic group, such as an acid anion, and a hydrophobic group, such as an alkyl chain with affinity to the hydrophobic plant surfaces, and thus the addition of surfactants to RNAlater should also reduce surface tension at the liquid/tissue interphase and improve RNAlater–tissue contact. We selected surfactants that: 1.) would dissolve or emulsify in RNAlater; 2.) could be added to RNAlater at small volumes that would not dilute its fixative properties; 3.) would not precipitate RNAlater salts. We tested four surfactants: 1) non-ionic Tween-20 that is nontoxic, stable, functions as a wetting agent, and is widely used as an emulsifier in several domestic, food production, and pharmacological applications; 2) non-ionic NP-40S that is very mild, non-denaturing, and acts as an emulsifier or mild lysis agent; 3) cationic CTAB that is a quaternary ammonium surfactant used in cell lysis during DNA extraction; 4) anionic Alconox that is mild and widely used in laboratories as a cleaning agent compatible with many material surfaces. NP-40S and Alconox were directly combined with RNAlater, while Tween-20 and CTAB caused RNAlater salts to precipitate and thus were dissolved in distilled water prior to mixing with RNAlater.
Dispensing RNAlater mixed with any of the surfactants on the Petri plates caused the air bubbles to round up (NP-40S and Tween-20), shrink (CTAB), or detach (Tween-20) from moss tissue within 24 h (Figure 3A). The quality of total RNA extracted from the moss tissue fixed in RNAlater mixed with any surfactant was low, and RNA showed moderate to high degradation (Figure 3B). Small rRNAs showed a high ratio relative to either 18S or 25S rRNA in all samples, including those with RIN values between 6.0 and 7.0. This implied that dislodging air bubbles, and therefore improving RNAlater adherence to the moss tissue, was not sufficient to achieve effective moss tissue fixation in RNAlater.
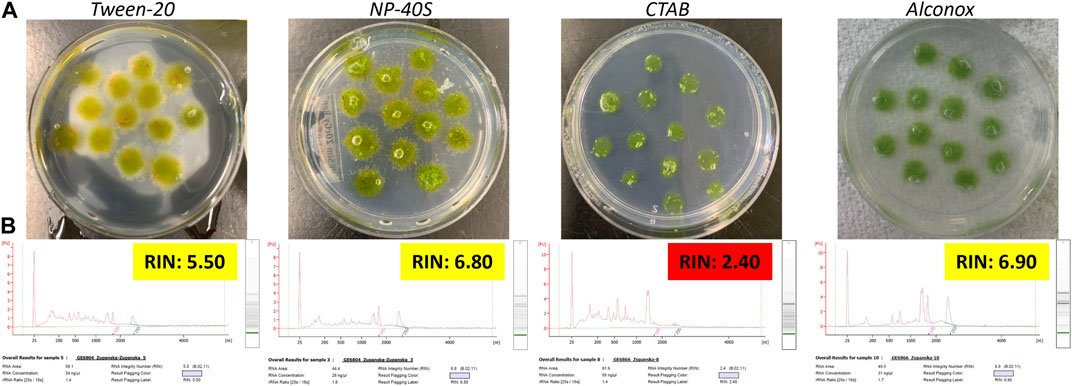
Figure 3. Top panel (A) Photographs of the ANT1 moss tissue submersed in RNAlater mixed with respective surfactants. Bottom panel (B) Electropherograms of total RNA extracted from moss tissue fixed in RNAlater mixed with respective surfactants for 24 h at room temperature, frozen at −80°C for 24 h, and then fully thawed. Final surfactant concentration in RNAlater was 0.003% Tween-20, 0.1% NP-40S, 0.01% CTAB, and 0.5% Alconox.
3.3 Adding surfactants in combination with oils to RNAlater slightly improves fixation of Antarctic moss tissue
We next focused on improving the permeation of RNAlater through the moss tissue. We deployed oil-based adjuvants that act on lipid solubilization that relaxes a plant cuticle structure (softening) and therefore, potentially aids penetration of low affinity polar solvents (Arand et al., 2018). We selected two adjuvant types: petroleum-based or plant-based oils mixed with surfactants that are widely used in agriculture. Each adjuvant formed an emulsion when added directly to RNAlater and did not cause any RNAlater salts to precipitate. Each adjuvant was used at three concentrations: 0.15, 0.25, and 0.5%.
The addition of each adjuvant mixed with RNAlater at any concentration caused air bubbles to round up and shrink within just 30 min of contact compared to 24 h in the case of surfactants alone (Figure 4A). Microscopic observations confirmed that air bubbles were only loosely attached to the moss filament periphery (data not shown). Tapping the Petri plates resulted in separation of all air bubbles from the tissue, further corroborating weakened air bubble adherence to the moss tissue with the presence of an adjuvant in RNAlater. Except for the sample fixed in RNAlater with the petroleum oil-based adjuvant at 0.25%, the quality of total RNA extracted from the fixed, frozen, and fully thawed moss tissue was low and showed moderate to high degradation. Although the sample fixed in RNAlater with 0.25% of the petroleum oil-based adjuvant showed lower RNA degradation, 25-nt-long RNA and small rRNAs were at high abundance, and the abundance of 25S rRNA was equal to that of 18S rRNA (Figure 4B). In neither case did RIN exceed 6.6 value. This implied that using adjuvants only slightly improved RNAlater permeation through the Antarctic moss tissue.
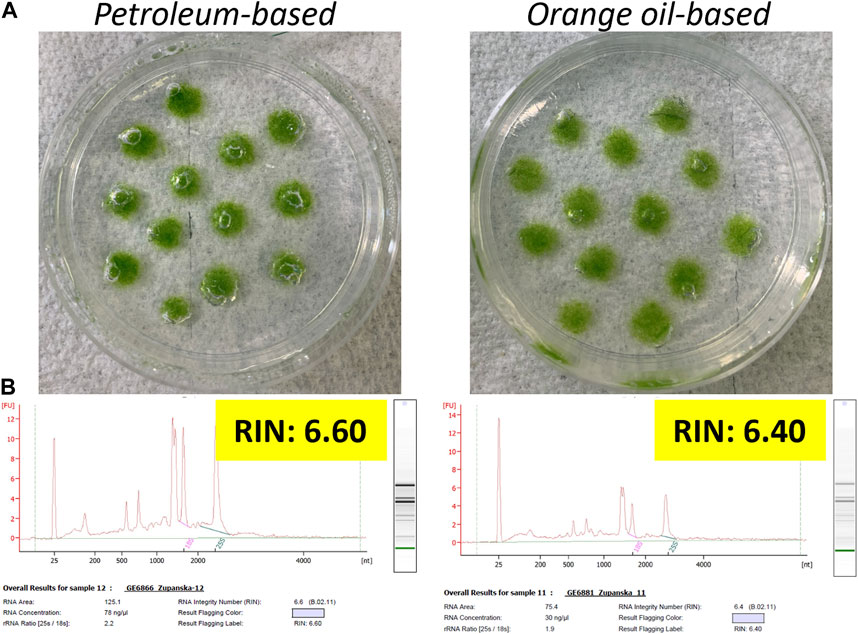
Figure 4. Top panel (A) Photographs of ANT1 moss tissue submersed in RNAlater mixed with the petroleum oil-based or orange oil-based adjuvants. Bottom panel (B) Electropherograms of total RNA extracted from the moss tissue fixed in RNAlater mixed with respective adjuvants for 24 h at room temperature, frozen at −80°C for 24 h, and then fully thawed. The final adjuvant concentration in RNAlater was 0.25%.
3.4 Deep-freeze results in proper Antarctic moss tissue fixation
We next evaluated the quality of total RNA extracted from the moss tissue that was deep-frozen without any chemical fixation. We froze the moss tissue by submersing Petri plates in liquid nitrogen at −196°C or inserting it into a −150°C or −80°C freezer. We extracted total RNA from the frozen moss tissue without allowing the moss tissue to thaw.
Total RNA extracted from the moss tissue frozen in the −150°C or −80°C freezer did not differ in quality from that extracted from the moss tissue snap-frozen in liquid nitrogen. Total RNA obtained from each sample was of very high quality, with the RIN values consistently exceeding 7.0. Across all samples, the abundance of 25S rRNA was the highest, and the contribution of the small RNA molecules to the total RNA was very low (Figure 5). The size distribution of total RNA obtained from any frozen tissue matched that of total RNA obtained from fresh, never-fixed tissue (see Figure 2B right). This demonstrated that rapid freeze is an effective fixation method for Antarctic moss tissue. Since the higher freezing temperature does not adversely affect total RNA quality, either the −150°C or −80°C freezer was a viable alternative to snap-freezing in liquid nitrogen.

Figure 5. Electropherograms of total RNA extracted from the frozen moss tissue that had never been thawed.
3.5 Freezing time of moss tissue at −150°C is considerably shorter than that at −80°C
Available freezers on the ISS to house a spaceflight payload and at the National Aeronautics and Space Administration (NASA) Kennedy Space Center to house a ground control provide −150°C or −80°C. Since deep-freezing fixation should occur very rapidly to capture an accurate spaceflight gene expression profile, we first measured the time required for the moss tissue on Petri plates to freeze in both temperatures. We placed the individual Petri plates with thermocouple sensors horizontally in direct contact with the metal shelf in the −150°C or −80°C freezer and registered temperature recordings over time. It took 290 s at −150°C for the moss tissue to achieve −5°C, while at −80°C it took 100 s longer (Figure 6). It took 330 s at −150°C for the moss tissue to achieve −20°C, while at −80°C, it took 130 s longer. It took only 410 s at −150°C for the moss tissue to achieve −70°C, while at −80°C, it took 1,180 s longer. This demonstrated that the use of a −150°C freezer would result in nearly 75% more rapid moss tissue fixation through the deep-freeze compared to −80°C.
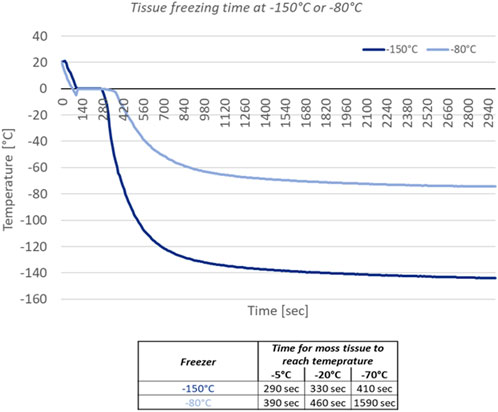
Figure 6. Plot of temperature over time based on thermocouple measurements collected from Petri plates inserted into the −150°C or −80°C freezer. Data table shows time in seconds needed for moss tissue to achieve the respective temperature in either freezer.
3.6 Freezing time of moss tissue depends on the Petri plate location in the plate stack and orientation
Minimizing the use of physical space in the freezer and reducing crew time dedicated to the payload operations is the priority while designing any spaceflight experiment. Therefore, we assessed whether the time for the moss tissue to freeze varied among Petri plates that were arranged in stacks. Petri plates in microgravity will not remain in contact with the metal freezer shelf but they will float; chilling will thus occur through cold air and not through heat exchange upon contact with a cold conductor. Therefore, to simulate the freezing scenario in microgravity, we separated the Petri plates from the metal surface with a layer of Styrofoam (Figure 7A). We inserted thermocouple sensors inside three Petri plates located at the top, middle, and bottom of a five-plate stack. The plate stacks were next laid on the side directly on the freezer shelf or on a layer of Styrofoam (Figure 7A). In each five-plate stack, each Petri plate from the side had a direct contact with a metal surface or Styrofoam, the top and bottom Petri plates in each stack had direct exposure to chilled air, and the middle Petri plate in a stack was insulated by two Petri plates on each side (Figure 7A).
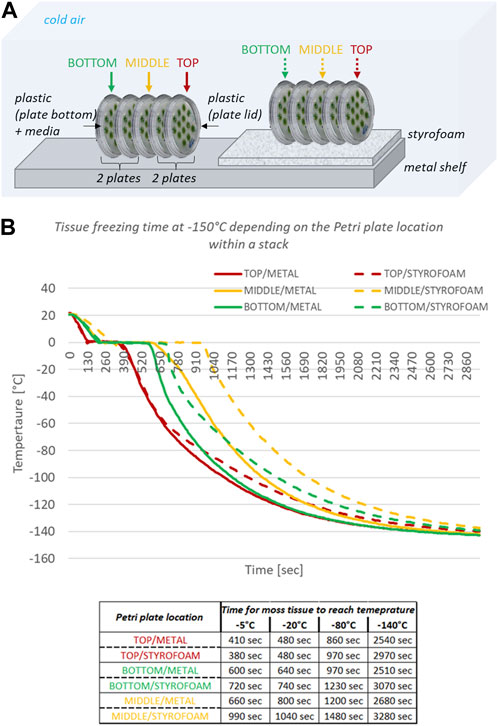
Figure 7. Top panel (A) Graphical presentation of deep-freeze test performed on Petri plates arranged in five-plate stacks and inserted into the −150°C freezer; in italics, factors affecting deep-freeze of the moss tissue. Bottom panel (B) Plot of temperature over time based on thermocouple measurements from top, middle, and bottom Petri plates in the five-plate stack. Data table shows time in seconds needed for moss tissue on designated Petri plates to achieve the respective temperature. Metal denotes a five-plate stack laid on the side directly on the metal shelf in the freezer; Styrofoam denotes a five-plate stack laid on the side and separated from the metal shelf by a layer of Styrofoam; top, middle, and bottom denote the position of a Petri plate within a five-plate stack.
The moss tissue on the Petri plate located on top of the stack froze fastest, followed by the moss tissue on the Petri plate located at the bottom of the stack, and the moss tissue on the Petri plate in the middle of the stack froze the slowest (Figure 7B). On the metal surface, the moss tissue on the top Petri plate achieved −80°C 110 s sooner than the moss tissue on the bottom Petri plate and 340 s sooner than the moss tissue on the middle Petri plate. On Styrofoam, the moss tissue on the top Petri plate achieved −80°C 260 s sooner than the moss tissue on the bottom Petri plate and 510 s sooner than the moss tissue on the middle Petri plate. The moss tissue on Petri plates within a five-plate stack in direct contact with the metal surface froze faster than the moss tissue on the corresponding Petri plates within a five-plate stack placed on Styrofoam (Figure 7B). The moss tissue on the top Petri plate placed on Styrofoam achieved −80°C 110 s later than the moss tissue on the top Petri plate placed directly on the metal surface. The moss tissue on the bottom Petri plate placed on Styrofoam achieved −80°C 260 s later than the moss tissue on the bottom Petri plate placed directly on a metal surface. The moss tissue on the middle Petri plate placed on Styrofoam achieved −80°C 280 s later than the moss tissue on the middle Petri plate placed directly on a metal surface. These data indicated that Petri plate contact with a metal surface is not critical to fast freezing of moss tissue and that the Petri plate position in the middle of the five-plate stack is least favorable for fast deep-freezing fixation.
3.7 Deep-freeze of Petri plates arranged in stacks results in proper Antarctic moss tissue fixation
We evaluated the quality of total RNA extracted from the moss tissue deep-frozen at −150°C on the Petri plates arranged in five-plate or three-plate stacks. The plate stacks were laid horizontally on top of a layer of Styrofoam at −150°C for 24 h and then transferred to −80°C. We extracted total RNA from the frozen moss tissue without allowing the tissue to thaw.
Total RNA extracted from the moss tissue frozen on the Petri plates arranged in the five- or three-plate stack was of very high quality, with RIN values consistently exceeding 7.0 (Figure 8). Across all samples, the abundance of 25S rRNA was the highest, and the contribution of the small RNA molecules to the total RNA was very low. The quality of RNA did not depend on the tissue freezing time and was similar for RNA extracted from the moss tissue located at different Petri plate locations within a plate stack.
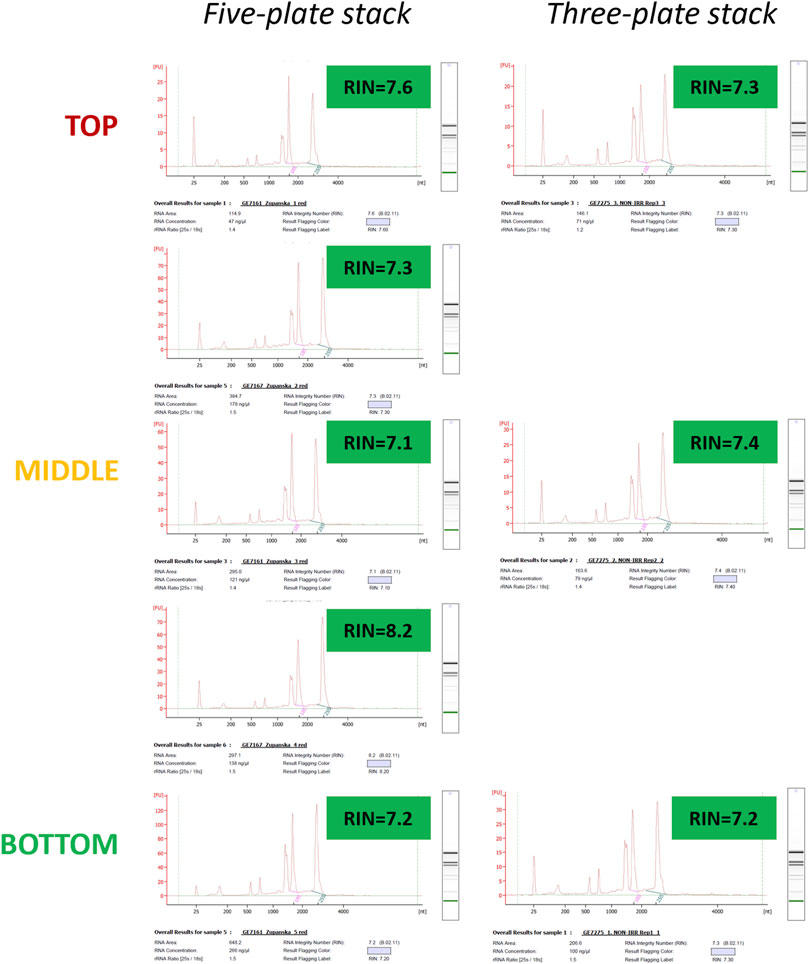
Figure 8. Electropherograms of total RNA extracted from the moss tissue deep-frozen at −150°C, while Petri plates were arranged in five-plate or three-plate stacks. Data obtained from the three-plate stacks have been collected during the ARTEMOSS experiment verification test (EVT).
4 Discussion
We here demonstrate that total RNA obtained from Antarctic moss tissue fixed in RNAlater is highly degraded and inadequate for downstream transcriptomic analysis. Antarctic moss eludes efficient fixation in RNAlater, despite the integration of various surfactants that modify the adherence of RNAlater to the moss tissue and combinations of surfactants with various types of oils that facilitate RNAlater permeation. We demonstrated that deep-freezing moss tissue on Petri plates provides adequate tissue fixation and allows the extraction of high-quality RNA suitable for gene expression profiling. After evaluating availability and tissue fixation time for various deep-freezing conditions, we designed the ARTEMOSS payload with considerations of constrained space and crew time.
Dispensing RNAlater on the moss tissue on a Petri plate revealed the presence of air bubbles adhered to moss filaments. The non-spherical air bubbles forming airfilm is indicative of the hydrophobic or super-hydrophobic surface rich in cuticular waxes (Krasowska et al., 2009). Moss C. purpureus isolate ANT1 was collected at Casey Bay in Antarctica—the driest and coldest of all terrestrial environments. Although the chemical characterization of the external surface of ANT1 is not available, plants from very cold or arid environments exhibit particularly high cuticular wax content, which is presumably instrumental in averting water loss and desiccation and is unlike plants from tropical and subtropical regions with abundant rainfall (Busta et al., 2016; Goldsmith et al., 2017; Hõrak, 2017; Waterman et al., 2017; Xue et al., 2017; Waterman et al., 2018). Additionally, other traits adaptive to survival in Antarctica have been identified in ANT1. For instance, the alkali extraction of cell-wall-bound phenolics, combined with methanol extraction of soluble phenolics, determined that ANT1 had six times higher concentration of cell-wall-bound UV-screening compounds than the concentration of intracellular UV screens (Clarke and Robinson, 2008a; Waterman et al., 2018). ANT1 also showed an exceptionally high content of biflavonoids and unknown phenolic compounds that are strongly bound to cell walls as well as being located inside the cells; they play dual roles as both UV-screening and antioxidant compounds that give this species advantage under damaging UV conditions during seasonal stratospheric ozone depletion (Waterman et al., 2017).
Total RNA extracted from the moss tissue submersed in RNAlater and retaining airfilm was of very poor quality, indicating inefficient fixation of Antarctic moss tissue in RNAlater. The presence of encapsulating air bubbles may limit the surface interaction between tissue and RNAlater. The addition of surfactants of different chemical composition to RNAlater reduced air bubble retention on the moss tissue and exposed most of the tissue surface to contact with RNAlater. However, surfactants did not satisfactorily improve the quality of total RNA extracted from the fixed moss tissue. This was unexpected, since the performance of surfactants in dispersing, spreading, sticking, and wetting has been successfully deployed in agriculture to improve the absorption of various active agents (Castro et al., 2013). In molecular biology, surfactants are successfully used as enhancements for the “floral dip” transformation of plants (Huynh et al., 2022). This suggested that poor fixation in RNAlater did not result solely from the ineffective fixative-tissue surface interaction and that dislodging air bubbles improves RNAlater adherence to the moss tissue but was still not sufficient to achieve effective ANT1 tissue fixation in RNAlater. Although the addition of surfactants to RNAlater did not improve ANT1 fixation, this strategy may be effective with other plants, particularly as the variety of plant species used in spaceflight investigations expands. Even highly hydrophilic plant surfaces when exposed to air trap air bubbles; however, in the presence of the buoyancy force on the ground, the submersion of plant tissue in a liquid causes air bubbles to detach and travel upward to the liquid surface. In microgravity, however, the lack of gravity-driven buoyancy and sedimentation within fluids causes non-departing small bubbles to coalesce and form sizable bubbles or even dry patches on a surface of biological or solid materials, irrespective of their hydrophobicity. Thus, the inability of air bubbles to naturally dislodge or burst likely compromises any liquid chemical delivery to a plant. Hence, targeting air bubble surface tension by using surfactants, like those tested in this investigation, could destabilize air bubbles, prevent coalescence, and ultimately remove bubbles from the system, thus improving biological experimentations in microgravity.
Modifying the permeation of RNAlater through the Antarctic moss tissue by adding oils in combination with surfactants resulted in a slightly better quality of extracted RNA. The minor improvement was unexpected since it has been demonstrated that the cuticular waxes form a permeation barrier to polar solvents, and their solubilization opens pathways that enable transport across the cuticle (Staiger et al., 2019). Hence, the symptoms observed of a change in cuticle texture are referred to as “softening” or even “dewaxing,” which dramatically improve the movement of polar solvents through the cuticle (Rourke and Gibbs, 1999). In agriculture, the addition of various types of emulsifiable oils increases the permeability of plant cuticles to foliage-applied polar pesticides (Nicetic et al., 2011). In our study, RNAlater in the presence of the petroleum oil-based adjuvant preserved RNA better than when mixed with orange oil-based adjuvant, suggesting that paraffin oil but not orange oil improved RNAlater permeation through the cuticular waxes. Since oil efficacy on modifying plant waxes depends on wax composition, structure, and chemical properties, it can be speculated that paraffin oil had higher affinity for waxes present in Antarctic moss cuticle than orange oil and thus solubilized waxes more effectively. The possibility exists that a carefully selected combination of an appropriate oil with detergents added to RNAlater would render adequate Antarctic moss tissue fixation and preserve good quality RNA. We also cannot rule out a scenario in which the inability of RNAlater to permeate the moss cells results from the presence of compounds other than, or in addition to, cuticular waxes.
As an alternative to RNAlater, we evaluated the efficacy of ethanol for Antarctic moss tissue fixation (Supplementary Material). Fixation in 70% ethanol resulted in high-quality RNA extracted from the moss tissue, but not at lower 30% and 50% concentrations, even though air bubbles never formed on moss tissue submersed at any concentration. Although ethanol seemed to be an effective fixative for plants with hydrophobic surfaces, it is not suitable for use in spaceflight due to its high flammability.
We demonstrated that deep-freezing Antarctic moss tissue is an effective fixation method that results in the preservation of very high-quality total RNA. The time required for the moss tissue on a Petri plate to achieve −5°C differed by 100 s between −80°C and −150°C freezers; however, the time for the moss tissue to achieve a deep-frozen state was substantially longer, by nearly 1,000 s (16 min 40 s) in the −80°C freezer than in the −150°C freezer, establishing a strong preference for using the GLACIER freezer over MELFI to fix our plant payload in-flight. We recreated a freezing scenario in microgravity by separating the Petri plates from the metal freezer surface with a layer of Styrofoam. Although the moss tissue on Petri plates placed on Styrofoam froze later than the moss tissue on Petri plates in direct contact with metal surface, the difference depended on the Petri plate configuration from 110 s (1 min 50 s) to 280 s (4 min 40 s) at −80°C. We demonstrated, however, that the substantial difference in the moss tissue freezing time resulted from a Petri plate being directly exposed to chilling air or being insulated by other Petri plates in a stack. Petri plates on both edges of the five-plate stacks achieved a deep-frozen state much faster than those in the middle of the stack, insulated by two Petri plates on each side. That difference was particularly large when the five-plate stack was placed on Styrofoam and reached 510 s (8 min 30 s) between the moss tissue on the top and middle Petri plate. The moss tissue on the top Petri plate froze slightly faster than that on the bottom Petri plate, although the five-plate stack was placed in the freezer horizontally and not vertically. This suggested that the moss tissue froze faster when separated from the cold air only by a plastic Petri plate lid as on the top Petri plate than when the tissue was separated from the cold air by a plastic Petri plate bottom and a layer of the media, as on the bottom of the Petri plate (Figure 7B). Thus, the least insulation of plant tissue from the cold air would shorten the deep-freeze fixation time. Therefore, for ARTEMOSS, we decided to construct stacks from just three instead of five Petri plates. Although the time to deep-freeze moss tissue on the Petri plates varied depending on the plate’s location within a stack, the difference in time did not affect the quality of RNA obtained from the moss tissue assessed by RIN. RNA extracted from the moss tissue harvested from Petri plates at each location within the plate stacks had RIN above 7.0.
Neither the effects of the sudden temperature drop below freezing or the differences in brief exposures to temperature falling from 0°C to −150°C on gene expression have been investigated in plants. However, even if some genes were activated during the deep-freezing process, they will appear in spaceflight but also in ground control gene expression profiles, and therefore such genes will not be wrongly asserted as spaceflight unique. Furthermore, numerous previous spaceflight experiments on Arabidopsis used the freezing method, predominantly in the −80°C MELFI freezer, to successfully preserve protein or transcript profiles; none reported enrichment in the cold-activated genes in the spaceflight samples (Kiss et al., 2011; Mazars et al., 2014; Barbero Barcenilla et al., 2023; Land et al., 2023).
Terrestrial plants from the edges of the limits of life have evolved adaptive traits that are seemingly favorable to thriving in the harsh environments inherent in human space exploration. However, these very adaptations may present challenges to routine experimental procedures, and working with plants from extreme environments will frequently require employing unconventional technological solutions. Based on the findings of this study, we designed in-flight fixation operations for the ARTEMOSS payload consisting of Antarctic moss on Petri plates that would best secure the transcript profile expressed in orbit. We will substitute the standard method of fixation in RNAlater with deep-freezing in GLACIER at −150°C. We will arrange the Petri plates in three-plate stacks to preserve the physical space and crew time at the fixation step, yet limit variations in the moss tissue freezing time on respective Petri plates. Three biological replicas per treatment will occupy each Petri plate position, top, middle, or bottom across a total of five three-plate stacks to correct for minor discrepancies in the moss tissue freezing time. The three-plate stacks at ground control will be placed on Styrofoam in the −150°C freezer to recreate fixing conditions in microgravity more accurately, where no contact with metal surface is maintained.
As we are poised to return to the Moon and take our terrestrial plants with us, chemical on-site fixation of plant tissue designated for sample return may not be an ideal approach. To achieve proper chemical fixation on the lunar surface, plants would need to grow in hardware preloaded with a fixative brought from Earth which would considerably increase upmass. Moreover, with time plants on the Moon will likely be grown in on site regolith in open containers rather than on nutrient media in hardware confinement. Therefore, the deep-freezing method, as evaluated in this investigation, which does not require special hardware or additional upmass, can be a viable alternative to preserve plant samples on the lunar surface for return to Earth. The necessity of returning ultracold geological samples has been long advocated. Here, we further demonstrate the value of incorporating long-term cryogenic containment systems on return missions for preserving biological investigations.
Data availability statement
The original contributions presented in the study are included in the article/Supplementary Material; further inquiries can be directed to the corresponding author.
Author contributions
AZ: conceptualization, investigation, methodology, and writing–original draft. EL: conceptualization, investigation, methodology, and writing–review and editing. YZ: conceptualization, methodology, and writing–review and editing. NH: conceptualization, methodology, and writing–review and editing. JC: conceptualization, investigation, methodology, and writing–review and editing. CS: conceptualization, investigation, methodology, and writing–review and editing. ES: project administration and writing–review and editing. JR: investigation, methodology, and writing–review and editing. LK: investigation, methodology, and writing–review and editing. DD: project administration, supervision, and writing–review and editing. SM: writing–review and editing.
Funding
The author(s) declare that financial support was received for the research, authorship, and/or publication of this article. This work was supported by the National Aeronautics and Space Administration—Biological and Physical Sciences (80NSSC22K0208 awarded to AZ and SM). Authors YZ, NH, JC, CS, ES, JR, LK and DD were supported by NASA's Biological and Physical Sciences Division and by NASA's ISS Program Office.
Acknowledgments
The authors would like to recognize the contributions of Steve Thorne (Berkley Alumni, The Copernican Project), Jean Jacques Royer, and Lev Filippov (University of Lorraine) for helpful discussions on air bubble behavior on plant tissue and thank them for their suggestions on air bubble destabilization.
Conflict of interest
Authors JR and LK were employed by AETOS Systems Inc.
The remaining authors declare that the research was conducted in the absence of any commercial or financial relationships that could be construed as a potential conflict of interest.
Publisher’s note
All claims expressed in this article are solely those of the authors and do not necessarily represent those of their affiliated organizations, or those of the publisher, the editors, and the reviewers. Any product that may be evaluated in this article, or claim that may be made by its manufacturer, is not guaranteed or endorsed by the publisher.
Supplementary material
The Supplementary Material for this article can be found online at: https://www.frontiersin.org/articles/10.3389/frspt.2024.1376163/full#supplementary-material
References
Arand, K., Asmus, E., Popp, C., Schneider, D., and Riederer, M. (2018). The mode of action of adjuvants-relevance of physicochemical properties for effects on the foliar application, cuticular permeability, and greenhouse performance of pinoxaden. J. Agric. Food Chem. 66 (23), 5770–5777. doi:10.1021/acs.jafc.8b01102
Aryal, B., and Neuner, G. (2010). Leaf wettability decreases along an extreme altitudinal gradient. Oecologia 162 (1), 1–9. doi:10.1007/s00442-009-1437-3
Aryal, B., and Neuner, G. (2016). Variability and extremes in leaf wettability and run-off properties in plants from various habitats. Res. Rev. J. Botanical Sci. 5, 23–30.
Barbero Barcenilla, B., Meyers, A., Castillo-Gonzalez, C., Young, P., Min, J.-H., Song, J., et al. (2023). Arabidopsis telomerase takes off by uncoupling enzyme activity from telomere length maintenance in space. Nat. Commun. 14, 7854. doi:10.1038/s41467-023-41510-4
Brewer, C. A., and Nuñez, C. I. (2007). Patterns of leaf wettability along an extreme moisture gradient in western patagonia, Argentina. Int. J. Plant Sci. 168 (5), 555–562. doi:10.1086/513468
Buschhaus, C., and Jetter, R. (2011). Composition differences between epicuticular and intracuticular wax substructures: how do plants seal their epidermal surfaces? J. Exp. Bot. 62 (3), 841–853. doi:10.1093/jxb/erq366
Busta, L., Budke, J. M., and Jetter, R. (2016). The moss Funaria hygrometrica has cuticular wax similar to vascular plants, with distinct composition on leafy gametophyte, calyptra and sporophyte capsule surfaces. Ann. Bot. 118 (3), 511–522. doi:10.1093/aob/mcw131
Cabrol, N. A., Feister, U., Häder, D.-P., Piazena, H., Grin, E. A., and Klein, A. (2014). Record solar UV irradiance in the tropical Andes. Front. Environ. Sci. 2. doi:10.3389/fenvs.2014.00019
Castro, M. J. L., Ojeda, C., and Cirelli, A. F. (2013). Surfactants in agriculture. Green Mater. Energy, Prod. Depollution 3, 287–334. doi:10.1007/978-94-007-6836-9_7
Clarke, L. J., and Robinson, S. A. (2008a). Cell wall-bound ultraviolet-screening compounds explain the high ultraviolet tolerance of the Antarctic moss, Ceratodon purpureus. New Phytol. 179 (3), 776–783. doi:10.1111/j.1469-8137.2008.02499.x
Colesie, C., Walshaw, C. V., Sancho, L. G., Davey, M. P., and Gray, A. (2023). Antarctica's vegetation in a changing climate. WIREs Clim. Change 14 (1), e810. doi:10.1002/wcc.810
Cove, D. J., Perroud, P.-F., Charron, A. J., McDaniel, S. F., Khandelwal, A., and Quatrano, R. S. (2009a). Culturing the moss Physcomitrella patens: figure 1. Cold Spring Harb. Protoc. 2009 (2), pdb.prot5136. pdb.prot5136. doi:10.1101/pdb.prot5136
Cove, D. J., Perroud, P.-F., Charron, A. J., McDaniel, S. F., Khandelwal, A., and Quatrano, R. S. 2009b. "Isolation and regeneration of protoplasts of the moss Physcomitrella patens: figure 1." Cold Spring Harb. Protoc. 2009 (2): pdb.prot5140. doi:10.1101/pdb.prot5140
Cove, D. J., and Quatrano, R. S. (2006). Agravitropic mutants of the moss Ceratodon purpureus do not complement mutants having a reversed gravitropic response. Plant Cell Environ. 29 (7), 1379–1387. doi:10.1111/j.1365-3040.2006.01519.x
Domínguez, E., Heredia-Guerrero, J. A., and Heredia, A. (2015). Plant cutin genesis: unanswered questions. Trends Plant Sci. 20 (9), 551–558. doi:10.1016/j.tplants.2015.05.009
Ferl, R., Zupanska, A., Spinale, A., Reed, D., Manning-Roach, S., Guerra, G., et al. (2011). The performance of KSC Fixation Tubes with RNALater for orbital experiments: a case study in ISS operations for molecular biology. Adv. Space Res. 48, 199–206. doi:10.1016/j.asr.2011.03.002
Goldsmith, G. R., Bentley, L. P., Shenkin, A., Salinas, N., Blonder, B., Martin, R. E., et al. (2017). Variation in leaf wettability traits along a tropical montane elevation gradient. New Phytol. 214 (3), 989–1001. doi:10.1111/nph.14121
Häder, D.-P., and Cabrol, N. A. (2020). Monitoring of solar irradiance in the high Andes. Photochem. Photobiol. 96 (5), 1133–1139. doi:10.1111/php.13276
Hõrak, H. (2017). Learning from the experts: drought resistance in desert plants. New Phytol. 216 (1), 5–7. doi:10.1111/nph.14753
Huynh, J., Hotton, S. K., Chan, R., Syed, Y., and Thomson, J. (2022). Evaluation of novel surfactants for plant transformation. BMC Res. Notes 15 (1), 360. doi:10.1186/s13104-022-06251-5
Kern, V. D., and Sack, F. D. (2001). Effects of spaceflight (STS-87) on tropisms and plastid positioning in protonemata of the moss Ceratodon purpureus. Adv. Space Res. 27 (5), 941–949. doi:10.1016/s0273-1177(01)00158-2
Kern, V. D., Schwuchow, J. M., Reed, D. W., Nadeau, J. A., Lucas, J., Skripnikov, A., et al. (2005). Gravitropic moss cells default to spiral growth on the clinostat and in microgravity during spaceflight. Planta 221 (1), 149–157. doi:10.1007/s00425-004-1467-3
Khayet, M., and Fernández, V. (2012). Estimation of the solubility parameters of model plant surfaces and agrochemicals: a valuable tool for understanding plant surface interactions. Theor. Biol. Med. Model 9, 45. doi:10.1186/1742-4682-9-45
Kidokoro, S., Shinozaki, K., and Yamaguchi-Shinozaki, K. (2022). Transcriptional regulatory network of plant cold-stress responses. Trends Plant Sci. 27 (9), 922–935. doi:10.1016/j.tplants.2022.01.008
Kiss, J. Z., Millar, K. D. L., Kumar, P., Edelmann, R. E., and Correll, M. J. (2011). Improvements in the re-flight of spaceflight experiments on plant tropisms. Adv. Space Res. 47 (3), 545–552. doi:10.1016/j.asr.2010.09.024
Koch, K., and Ensikat, H. J. (2008). The hydrophobic coatings of plant surfaces: epicuticular wax crystals and their morphologies, crystallinity and molecular self-assembly. Micron 39 (7), 759–772. doi:10.1016/j.micron.2007.11.010
Kovalchuk, I., Abramov, V., Pogribny, I., and Kovalchuk, O. (2004). Molecular aspects of plant adaptation to life in the Chernobyl zone. Plant Physiol. 135 (1), 357–363. doi:10.1104/pp.104.040477
Krasowska, M., Zawala, J., and Malysa, K. (2009). Air at hydrophobic surfaces and kinetics of three phase contact formation. Adv. Colloid Interface Sci. 147-148, 155–169. doi:10.1016/j.cis.2008.10.003
Kruse, C. P. S., Basu, P., Luesse, D. R., and Wyatt, S. E. (2017). Transcriptome and proteome responses in RNAlater preserved tissue of Arabidopsis thaliana. PLOS ONE 12 (4), e0175943. doi:10.1371/journal.pone.0175943
Land, E. S., Sheppard, J., Doherty, C. J., and Perera, I. Y. (2023). Conserved plant transcriptional responses to microgravity from two consecutive spaceflight experiments. Front. Plant Sci. 14, 1308713. doi:10.3389/fpls.2023.1308713
Manzano, A., Creus, E., Tomas, A., Valbuena, M. A., Villacampa, A., Ciska, M., et al. (2020). The FixBox: hardware to provide on-orbit fixation capabilities to the EMCS on the ISS. Microgravity Sci. Technol. 32, 1105–1120. doi:10.1007/s12217-020-09837-5
Mazars, C., Brière, C., Grat, S., Pichereaux, C., Rossignol, M., Pereda-Loth, V., et al. (2014). Microgravity induces changes in microsome-associated proteins of Arabidopsis seedlings grown on board the international space station. PLOS ONE 9 (3), e91814. doi:10.1371/journal.pone.0091814
McDaniel, S. F., and Shaw, A. J. (2005). Selective sweeps and intercontinental migration in the cosmopolitan moss Ceratodon purpureus (Hedw.) Brid. Mol. Ecol. 14 (4), 1121–1132. doi:10.1111/j.1365-294X.2005.02484.x
Mousseau, T. A., and Møller, A. P. (2020). Plants in the light of ionizing radiation: what have we learned from chernobyl, fukushima, and other "hot" places? Front. Plant Sci. 11, 552. doi:10.3389/fpls.2020.00552
Nairn, J. J., Forster, W. A., and van Leeuwen, R. M. (2016). Effect of solution and leaf surface polarity on droplet spread area and contact angle. Pest Manag. Sci. 72 (3), 551–557. doi:10.1002/ps.4022
Nicetic, O., Cho, Y. R., and Rae, D. J. (2011). Impact of physical characteristics of some mineral and plant oils on efficacy against selected pests. J. Appl. Entomology 135 (3), 204–213. doi:10.1111/j.1439-0418.2010.01553.x
Renault, H., Alber, A., Horst, N. A., Basilio Lopes, A., Fich, E. A., Kriegshauser, L., et al. (2017). A phenol-enriched cuticle is ancestral to lignin evolution in land plants. Nat. Commun. 8, 14713. doi:10.1038/ncomms14713
Riederer, M., and Schreiber, L. (2001). Protecting against water loss: analysis of the barrier properties of plant cuticles. J. Exp. Bot. 52 (363), 2023–2032. doi:10.1093/jexbot/52.363.2023
Rourke, B. C., and Gibbs, A. G. (1999). Effects of lipid phase transitions on cuticular permeability: model membrane and in situ studies. J. Exp. Biol. 202, 3255–3262. doi:10.1242/jeb.202.22.3255
Sack, F. D. (1993). Gravitropism in protonemata of the moss Ceratodon. Memoirs Torrey Botanical Club 25 (1), 36–44.
Sievers, A., Buchen, B., and Hodick, D. (1996). Gravity sensing in tip-growing cells. Trends Plant Sci. 1 (8), 249–250. doi:10.1016/1360-1385(96)10028-5
Staiger, S., Seufert, P., Arand, K., Burghardt, M., Popp, C., and Riederer, M. (2019). The permeation barrier of plant cuticles: uptake of active ingredients is limited by very long-chain aliphatic rather than cyclic wax compounds. Pest Manag. Sci. 75 (12), 3405–3412. doi:10.1002/ps.5589
Tavernier, E.-K., Lockwood, E., Perroud, P.-F., Nogué, F., and McDaniel, S. F. (2023). Establishing CRISPR-Cas9 in the sexually dimorphic moss. Ceratodon purpureus 2023, 562971. doi:10.1101/2023.10.25.562971
Thornton, L. E., Keren, N., Ohad, I., and Pakrasi, H. B. (2005). Physcomitrella patens and Ceratodon purpureus, mosses as model organisms in photosynthesis studies. Photosynth Res. 83 (1), 87–96. doi:10.1007/s11120-004-5577-3
Tomasi, P., and Abdel-Haleem, H. (2023). Phenotypic diversity in leaf cuticular waxes in Brassica carinata accessions. Plants (Basel) 12 (21), 3716. doi:10.3390/plants12213716
Waterman, M. J., Bramley-Alves, J., Miller, R. E., Keller, P. A., and Robinson, S. A. (2018). Photoprotection enhanced by red cell wall pigments in three East Antarctic mosses. Biol. Res. 51 (1), 49. doi:10.1186/s40659-018-0196-1
Waterman, M. J., Nugraha, A. S., Hendra, R., Ball, G. E., Robinson, S. A., and Keller, P. A. (2017). Antarctic moss biflavonoids show high antioxidant and ultraviolet-screening activity. J. Nat. Prod. 80 (8), 2224–2231. doi:10.1021/acs.jnatprod.7b00085
Xue, D., Zhang, X., Lu, X., Chen, G., and Chen, Z.-H. (2017). Molecular and evolutionary mechanisms of cuticular wax for plant drought tolerance. Front. Plant Sci. 8, 621. doi:10.3389/fpls.2017.00621
Yeats, T. H., and Rose, J. K. (2013). The formation and function of plant cuticles. Plant Physiol. 163 (1), 5–20. doi:10.1104/pp.113.222737
Keywords: extreme environments, plants, Ceratodon, spaceflight, microgravity, ISS, chemical fixation, deep-freeze
Citation: Zupanska AK, Lockwood E, Zhang Y, Haveman NJ, Carver JA, Spern CW, Senyk E, Richards JT, Koss LL, Dimapilis DI and McDaniel SF (2024) Designing payload and spaceflight operations for plants from extreme terrestrial environments. Front. Space Technol. 5:1376163. doi: 10.3389/frspt.2024.1376163
Received: 25 January 2024; Accepted: 27 February 2024;
Published: 03 April 2024.
Edited by:
Michael Lebert, University of Erlangen Nuremberg, GermanyReviewed by:
Raul Herranz, Spanish National Research Council (CSIC), SpainJack J. W. A. van Loon, VU Amsterdam, Netherlands
Copyright © 2024 Zupanska, Lockwood, Zhang, Haveman, Carver, Spern, Senyk, Richards, Koss, Dimapilis and McDaniel. This is an open-access article distributed under the terms of the Creative Commons Attribution License (CC BY). The use, distribution or reproduction in other forums is permitted, provided the original author(s) and the copyright owner(s) are credited and that the original publication in this journal is cited, in accordance with accepted academic practice. No use, distribution or reproduction is permitted which does not comply with these terms.
*Correspondence: Agata K. Zupanska, azupanska@seti.org