- 1MARBEC, Université de Montpellier, CNRS, IFREMER, IRD, France
- 2Université de Lorraine, SIMPA, Nancy, France
Food production and balanced nutrition will be a key challenge for residents of a future base on the Moon or Mars. As a complement to photosynthetic organisms, space aquaculture could provide the range of amino acids required to maintain health. This would rely on shipping fertilized aquaculture fish eggs to the Moon. To determine the feasibility of this, this study sought to test the influence of the conditions of a lunar mission—such as hypergravity during rocket launch and microgravity during the journey—on fish embryos and young larvae. To analyze the potential effects of these gravity changes on the early developmental stages of fish, we conducted two experiments to expose them to: i) 10 min of simulated hypergravity at 5 g (launch duration) and ii) 39 h of simulated microgravity using a random positioning machine. Both experiments used European sea bass (Dicentrarchus labrax) as a model. We analyzed egg oxygen consumption and hatching rates, as well as the expression of genes related to stress and immunity. The results indicated that neither of these altered gravity conditions affected the hatching rate. Simulated microgravity did not impact fish embryo oxygen consumption and appeared to induce faster embryonic development, as the eggs hatched earlier than expected. Levels of glucocorticoid receptors (GR1 and GR2) and heat shock proteins (Hsp90) were not impacted. Only the levels of complement component protein 3 (C3) were significantly higher in simulated microgravity, while interleukin-1β (IL-1β) transcripts were significantly lower in the hypergravity group, compared to controls. This shows that proteins involved in the innate immune system are expressed under altered gravity. Although further experiments are needed, these results suggest that the European sea bass is a promising candidate for space aquaculture.
Introduction
The presence of a human community on the Moon or on Mars for long-term residence would require a production unit allowing partial or total food autonomy in addition to cargo deliveries. One of the major objectives of a bioregenerative life-support system (BLSS) is to provide food sources for crewed missions using in situ resources and converting waste molecules into food biomass (Przybyla, 2021). The nutritive quality of aquatic organisms (Tacon et al., 2020) makes them prospective candidates to supplement the nutrients supplied by photosynthetic organisms, such as plants (Poulet et al., 2022) or microalgae (Fahrion et al., 2021), which have already been studied in the context of space missions. Resupplying a lunar base from Earth with fresh food on a weekly or monthly basis is neither economically nor technologically feasible. A short-term solution is to provide processed, prepackaged space food. However, lyophilized conservation is unstable, especially concerning essential nutrients such as potassium, calcium, vitamin D, and vitamin K, which are involved in muscle and bone maintenance. The micronutrients most sensitive to storage degradation are vitamins A, C, B1, and B6 after 1 year at ambient temperature (Cooper et al., 2017). A possible nutrition strategy for space bases could be to couple local production of fresh food with supplies brought by cargo spaceships.
Space aquaculture could be planned and integrated into a lunar base’s agricultural activities (Przybyla, 2021). Incorporating this activity in addition to greenhouse biomass production or an environmental control and life-support system (ECLS) would increase the level of sustainability over time (Irons and Irons, 2023). The Lunar Hatch program, supported by the French Space Agency (CNES) and the French Institute for Ocean Science (Ifremer), is investigating the feasibility of developing space aquaculture by studying the viability of shipping embryonated fish eggs from the Earth to the Moon. The main growth period of the fish would be on the Moon, where they would be cultivated in a closed circular system until they reached an edible size. This program is experimenting with the European seabass (Dicentrarchus labrax) as a model for space aquaculture. Before a real space mission, the feasibility of the concept has to be tested by exposing fish embryos to the environmental changes they would be subjected to during a Moon journey to determine if this affects their development. In the framework of the Lunar Hatch program, a first experiment was performed to study the impact of rocket vibrations on the embryogenesis of the European sea bass and the meagre (Argyrosomus regius). The results showed no significant difference on the egg hatching rate for either species after a Soyuz qualifying test, whatever the embryo development stage (Przybyla et al., 2020). Following this rocket vibration experiment, it was decided to perform a second experiment involving altered gravity.
Fish embryos in hypergravity conditions
During a space mission, fish embryos are exposed to two types of altered gravity. The first is hypergravity (∆g > 1 g) during launcher acceleration. During this 10-min take-off phase, gravity could be 3 g<∆g < 5 g depending on the thruster power of the launcher. The degree of acceleration varies according to the velocity of the launcher, the fuel burned by the engine, and the mass of the rocket. A study on the larvae of cichlid fish (Oreochromis mossambicus) and juveniles of the swordtail (Xiphophorus hellerii) looked at fish behavior, neuronal tissue and the otolith in these conditions, finding that fish have hypergravity perception (Brungs et al., 2011). In a permanent 3 g environment, developed fish showed a slight lateral tilt during swimming, probably due to asymmetric otolith growth (Hilbig and Anken, 2017). However, the influence of hypergravity on fish embryo development is poorly documented. In research investigating otolith formation, fertilized zebrafish (Danio rerio) eggs were reared at 3 g for 7 days. In the control group, by 16 h post fertilization (hpf) at 1g, precursors of the otoliths were observed. Zebrafish reared at 3 g from 1 to 7 days after fertilization exhibited significantly slower otolith growth than the controls at 1 g (Wiederhold et al., 2003). At 24 hpf, decreased pigmentation was observed when fertilized zebrafish eggs were under 3 g gravity. One day later, the difference with the control batch (1 g) disappeared (Aceto et al., 2015). A study with zebrafish egg and post-larvae under 7 days at 3 g exposure showed changes in their swimming and flotation patterns. Hatching rate showed a significant delay between 2- and 3-day post fertilization. Moreover, long-term hypergravity impacts the gene expression in genes implicated in epigenetic mechanisms (Salazar Moscoso et al., 2022). Medical research has applied long-term hypergravity (several days) to aquarium fish models to better understand vertebrate physiology. However, in a real space mission, this environment lasts only 10 minutes—the time to reach LEO. Therefore, this shorter duration of hypergravity was used for our study.
Fish embryos in microgravity
When ∆g < 1g, organisms are under microgravity. In a space mission, gravity is 10−3 g<∆g < 10−5 g (Black et al., 1995), whereas on the Moon it is ∆g = 0.166 g. In medical research, larval or juvenile fish have been used as animal models in LEO to investigate the development of the vestibular system and otolith in order to gain a better understanding of the human inner ear (Anken et al., 2016). In this context, ground-based experiments have been conducted to simulate microgravity using a drop tower (Anken and Hilbig, 2009), parabolic flight (Anken et al., 1998; Endo et al., 2002) or a clinostat/rotating wall vessel (Brungs et al., 2011; Edsall and Franz-Odendaal, 2014).
Multicellular life on Earth appeared around two billion years ago. The gravity on Earth is 1g, influencing the phylogenic development of living organisms. Compared to terrestrial vertebrates, aquatic organisms are subject to gravitational force and an opposing buoyancy force. This results in an aquatic organism feeling a reduced body weight compared to a terrestrial organism. This is the reason astronauts train in pools or simulate extravehicular activities in marine environments (Trout and Bruchey, 1969). The aquatic environment suggests that fish embryo development may be less impacted by microgravity than hypergravity in altered gravity conditions.
Determining the feasibility of space aquaculture requires switching from an aquarium fish model to an aquaculture fish model to conduct experiments on their biology in space (Przybyla, 2021). A journey to the Moon is the first step to verify before planning a potential aquaculture system for a future lunar base. This requires studying embryo/larvae survival rates, behavior and biological status in the conditions experienced during a lunar trip in order to optimize fish welfare.
Glucocorticoid receptors and chaperone heat shock protein Hsp90α
Cortisol, a steroid hormone belonging to the glucocorticoid family, is involved in the regulation of various physiological mechanisms, including inflammation, metabolism and stress response. In fish, as in many other vertebrates, cortisol is considered the main stress hormone (Sadoul and Geffroy, 2019). It binds to glucocorticoid receptors (GRs) and mineralocorticoid receptors to activate or repress a number of genes, contributing to physiological adaptation following stress. The European seabass possesses two glucocorticoid receptors: GR1 and GR2. Expression of genes encoding these proteins has been detected early in an individual’s development in the species and used as a stress marker in various studies (Di Bella et al., 2008; Pavlidis et al., 2011; Tsalafouta et al., 2014; Geffroy et al., 2021).
Heat shock proteins are involved in animal development (Rutherford and Lindquist, 1998), as well as in fish stress response (Palmisano et al., 2000). In European seabass, Hsp90α is a chaperone protein for both GR1 and GR2 and plays a key role in regulating the availability of GRs for cortisol. Hsp90α activity has mostly been described during stress experiments related to exposure to warm temperatures, including in the seabass (Goikoetxea et al., 2021) and the gilthead bream Sparus aurata (Madeira et al., 2016). Monitoring GR1, GR2 and Hsp90α during altered gravity experiments can give us useful information on the development and the stress level of larvae hatched in microgravity or after being subjected to a hypergravity environment during embryo development.
Innate immune system: complement component protein C3 and interleukin-1β
Fish embryos and larvae have an efficient defense system to deal with aquatic pathogens, although they lack an adaptive immune system (with memory, and thus specific to the individual), which becomes functional at a later developmental stage. To recognize and efficiently destroy pathogens at an early stage of development, the innate immune system (without memory and non-specific) is activated via different pathways (Magnadóttir, 2006; Uribe et al., 2011). The key component of the innate immune system is the complement component 3 protein (C3), which plays a central role in the lytic and pro-inflammatory pathways (Yano et al., 1988; Najafpour et al., 2020). This protein was identified in the digestive system of Atlantic halibut larvae (Hippoglossus L.) as early as the onset of first feeding, 50 days after hatching (Hermannsdottir et al., 2009). Complement proteins have also been shown to be involved in tissue and organ regeneration. Distinct complement-triggered pathways have been shown to modulate critical responses that promote tissue reprogramming, pattern formation and regeneration across phylogenesis (Mastellos et al., 2013). Thus, monitoring C3 protein could provide information on the development of the non-specific immune system in altered conditions, assuming that environmental changes may have an impact on this.
Interleukin-1β (IL-1β) expression has been reported in several studies on vertebrates during spaceflight (Crucian et al., 2014; Buchheim et al., 2019; Krieger et al., 2021). It is an initiator of inflammation, and its expression in vertebrates can also be a response to stress (Brydon et al., 2005; Serradell et al., 2020). IL-1β has been studied in 13 teleost fish species, and it seems to function in a similar way as in other vertebrates including mammals (Uribe et al., 2011). Given that inflammation or stress can cause tissue damage affecting various organs and physiological systems of fish, in our study we quantified IL-1β transcripts to determine if simulated altered gravity impacts the expression of this innate immune gene in D. labrax embryo development.
Our study focused on the hatching rate and oxygen consumption of aquaculture fish eggs during a ground-based experiment simulating hypergravity using a centrifuge and simulating microgravity using a random positioning machine (RPM). The parameters studied after exposure to altered gravity were the RNA integrity number (RIN), the relative expression of glucocorticoid receptors (GR1, GR2), and Hsp90a in order to assess the stress level after conditions based on a real space mission. The C3 and IL-1β transcripts aimed to assess the early immune system status after exposure to both altered conditions just after hatching. The aim of this study was to lay the groundwork for a proof of concept of the feasibility of hatching aquaculture fish in LEO or on a future lunar base and to anticipate any countermeasures that may need to be implemented for fish welfare during such a mission.
Materials and methods
The exposure of D. labrax sibling embryos to simulated microgravity and hypergravity conditions was conducted at the European Space Agency (ESA) facility in Nancy, France, in separate experimental rooms, with a control group in each room. In order to evaluate the hatching rate and compare the viability of the fish, a triplicate was reared in industrial conditions that are optimal for fish production at the Ifremer aquaculture station (Palavas-les-Flots, France). The general outline of the experimental protocol is presented in Figure 1.
Experimental tanks
To conduct the experiment, a polyvinyl chloride experiment tank was built to sit inside a CubeSat (a miniaturized satellite), which is 10 cm × 10 cm x 10 cm (Figure 2A/Figure 2B). The tank diameter was 6.4 cm and it weighed 639 g when filled with water; the tank volume was 230 mL. The altered gravity experimental groups were then hermetically sealed into the CubeSats and the control groups were sealed in bottles without the possibility of renewing the water or injecting oxygen. While the experimental CubeSat can carry 200 European seabass eggs (the industrial protocol is 1 egg/mL), for this experiment on altered gravity, we decided to reduce the number of samples to 100 eggs to ensure a comfortable environment (0.43 eggs/mL), especially in terms of oxygen availability for the entire week of testing.
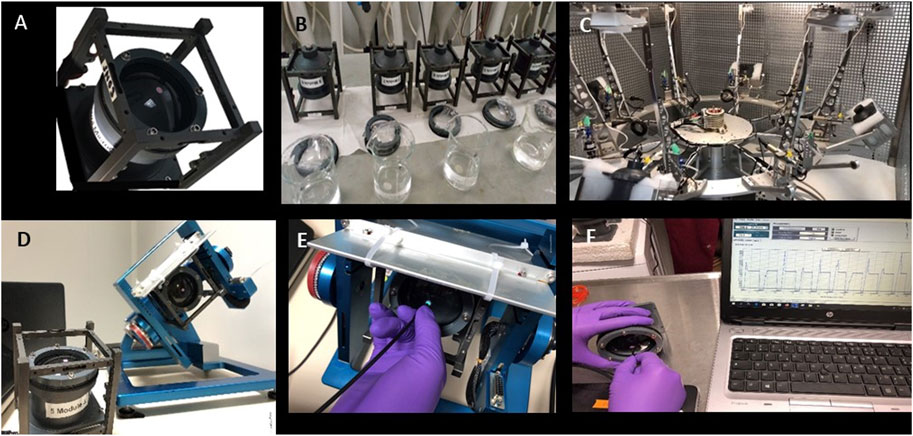
FIGURE 2. (A), (B) Experimental tank (230 mL) inside a CubeSat (n = 100 eggs) used in this experiment as well as in previous experiments to assess the impact of rocket launch vibrations on aquaculture fish hatching rate (C) Aquatic rotor (from 1 g to 5 g) with experimental tanks in triplicate containing European seabass embryos (n = 100 each) (D) Random positioning machine with a CubeSat containing European seabass embryos (n = 100) exposed for 39 h of simulated microgravity and the control CubeSat (n = 100) (E) PSt3 oxygen sensor and light transmitted by the optical fiber to the sensor spot for oxygen concentration measurement inside of the CubeSat window (F) Oxygen level concentration data transfer to the computer.
Aquaculture fish egg fertilization
A week before the experiment, four male and four female Dicentrarchus labrax were selected at the Ifremer aquaculture station (Palavas-les-Flots, France) based on sperm availability and ovulation stage. The selected fish were then isolated in a recirculating aquaculture system (RAS) regulated at 13.2 °C. Two days before the date of the scheduled fertilization, the females were injected with gonadotropin-releasing hormone (10 μg/mL). The day of fertilization, the females were ready for egg collection. Only one male and one female with the best oocyte quality were selected for controlled fertilization in order to produce a single family for the hypergravity experiment (HGE) and the simulated microgravity experiment (SMGE) to minimize parental influence on the results. A volume of 2 mL of oocytes (n ≈ 2000) was sampled and placed into a small 150-mL beaker, assessed under the microscope and sprayed with 1 mL of fresh sperm. The fertilized eggs were then placed into an aquatic incubator at 14.5 °C, pH ≈ 8.15 and salinity ≈38%. Viable eggs were collected to be sampled.
Aquaculture fish egg sample preparation
At 20 hpf, the fish eggs (size ≈1.1 mm) were transparent, and the embryonic dome was easily visible without a clarifying agent. Due to the possible presence of dead eggs (which are a brown color), all eggs were visually selected by the same operator using a binocular microscope (Motic-w10 × 20). A total of 1,025 European seabass eggs were assessed, counted and prepared to make a pool for the hypergravity and microgravity experiments, the controls, and the control group kept in the local industrial environment. The industrial environment (Ifremer research station) is considered to provide optimal rearing conditions according to the standard RAS protocol, which has been performed successfully for more than 30 years. This control group was not subjected to the altered gravity conditions. All CubeSats and bottles were filled with filtrated (0.2 µm) water oversaturated with oxygen (240% of air saturation).
Oxygen measurement inside the CubeSat
The rate of oxygen consumption of fish embryos is difficult to measure, due to the small size of eggs and low rates of oxygen uptake. The oxygen concentration inside the CubeSat modules was regularly measured using non-invasive optical fiber technology. This method avoided opening the sealed CubeSat with the risk of degassing, preserving the fish embryos from possible stress. By placing the edge of the fiber in front of the sensor (Figure 2E), the transmitter is able to analyze the received signal and translate it into an oxygen rate (in % of air saturation) readable directly by Presens FiBox software (Figure 2F). Calibration was required before the measurement, using Milli-Q water at 0% and 100% of oxygen saturation. The temperature of the module was recorded for each measurement because this influences the calculation of oxygen concentration. All modules were filled at 22 hpf with the same marine water with constant salinity (34.8 PSU). A measurement with filtrated water (0.2 µm to avoid bacteria presence and respiration) and without eggs showed that the CubeSat lost about 15% of oxygen, probably due to a porous gasket. This loss was considered in the calculation of oxygen consumption. Oxygen measurement was performed for all groups just after the CubeSat module was hermetically sealed at 22 hpf.
Embryo oxygen consumption was calculated for the SMGE and control following the formula below:
A: [Biological oxygen loss] (µgO2/Egg)
B: [Number of hours since CubeSat sealing]
Oxygen consumption during the microgravity experiment (µgO2/eggs/h) was calculated with A/B.
Transport of fish eggs from the Ifremer laboratory to the ESA’s Gravitational Experimental Platform for Animal Models (GEPAM)
At 22 hpf, all groups for the altered gravity experiments were transferred to the European Space Agency GEPAM platform (Bonnefoy et al., 2021), at a distance of around 700 km, in a specific thermoregulated vehicle (16 °C). A triplicate stayed at the Ifremer laboratory and was considered as the control group in industrial conditions. The experimental groups, experimental controls and scientific team reached the gravitational experimental platform after a trip of 6 h 45.
Microgravity experiment
The simulated microgravity room was maintained at 18.1±0.8 °C using air conditioning. At 34 hpf, the spherical container (6 cm in diameter) of a CubeSat filled with 230 mL of seawater (density 1.026 Kg.m3, viscosity 1.13 mPa s) and 100 eggs (each measuring ≈1.1 mm) was attached centrally between the arms of a random positioning machine (RPM) made by Dutch Space BV (Figure 2D). An RPM is a 3D-clinostat commonly used to simulate microgravity; a study by Herranz et al. (2013) indicated that an RPM could be used with fish larval stages before they can swim freely, which was the case here. The RPM was set to rotate in “full random mode” at a random speed, direction and interval, with an angular rotation velocity between 55 and 65° per second for 39 h. The continuous random change in orientation generates effects similar to those of true microgravity in space by cancelling the terrestrial g force; this device is frequently used for ground-based experiments and is described as the best simulation of these conditions (Hemmersbach et al., 2018). During the microgravity experiment, the RPM was stopped 3 times for less than 5 min to measure oxygen levels. Oxygen measurements were carried out at 56 hpf, at the middle and at the end of microgravity exposure.
The CubeSat used as a control was prepared in an identical way to the one exposed to the RPM conditions: it contained 100 eggs at the same developmental stage and was placed in the same experimental room with controlled lighting and ventilation.
Once the eggs hatched, both the exposed and control modules (n = 100 each) were opened to count the larvae, which were sampled for biomarker analysis. Hatching rates from both CubeSats were assessed only on groups at 72 hpf.
It should be noted that random positioning machines have certain limitations compared to real exposure in space, particularly because the alteration in gravity is focused on the center of the rotation. However, in our experiment, the radius of the sphere used as the egg tank inside the CubeSat was small (3 cm) and well centered between the arms. The eggs themselves are spherical, and their movement inside the spherical tank during RPM exposure can be likened to a kind of lottery ball machine. While we cannot accurately calculate the amount of residual gravity, we can consider that all eggs had the same likelihood of spending residence time in the center of the RPM. The 3D movement promotes mixing to the center of the CubeSat, resulting in the embryos having the same exposure to altered gravity over the 39 h of the experiment.
Hypergravity experiment
The aquatic rotor of the GEPAM facility has eight gondolas (Figure 2C), each accommodating enough space for a CubeSat tank. At 49 hpf, three CubeSats (n = 100 embryos each) were placed in non-consecutive gondolas in order to balance the device. The acceleration chosen for this test was 5g, which is slightly more than SLS-Artemis 1 and all previous Apollo missions (Table 1, and Table 2).
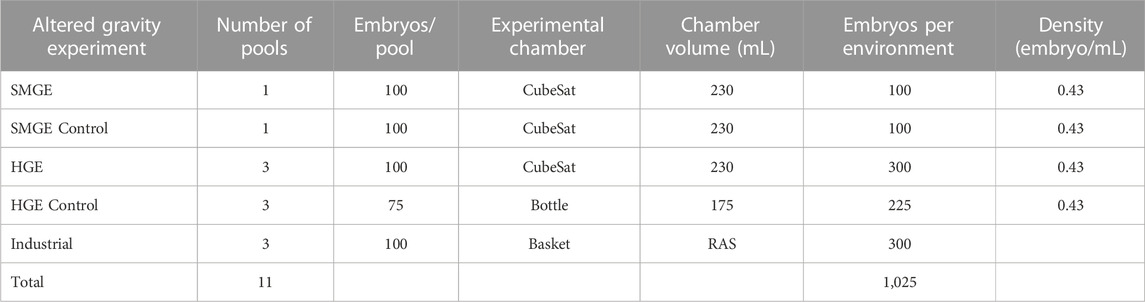
TABLE 1. European seabass egg preparation for hatching rate assessment in altered gravity and the control environment.

TABLE 2. Launcher acceleration (in g) recorded for lunar missions (data on Apollo missions sourced from Ascent Data, NASA, https://history.nasa.gov/SP-4029/Apollo_18-20_Ascent_Data.htm).
The experimental triplicate was exposed to 10 min in the centrifuge and the triplicate control group was placed in the same room. After exposure, all groups were put in a thermoregulated chamber (14.8±1.6 °C) until the hatching rate assessment.
Hatching rate evaluation and post-exposure sampling
Hatching in both gravity experiments occurred during the night. Counting to measure the hatching rate and biomarker sampling took place around 4 h after the peak of hatching in the simulated microgravity experiment (at 96 hpf) and in the hypergravity experiment (at 72 hpf). The difference between the two hatching periods was correlated to the ambient temperature difference inside the two experimental rooms and the temperature inside the CubeSat.
The hatching rate assessment protocol is described in Przybyla et al. (2020). Pre-larvae were sampled and fixed in order to analyze the RIN, as well as the relative expression of GR1, GR2, Hsp90a, C3 and IL-1β mRNA. Given the very early stage of development, the samples consisted of a pool of pre-larvae.
For the hypergravity experiment, one aliquot of 20 pre-larvae was sampled from each of the exposed and control CubeSats (n = 6). For the microgravity experiment, due to the fact that only one CubeSat could be attached to the RPM and that not all eggs hatched, the availability of eggs for an aliquot was more limited. As a result, three aliquots of 5, 10 and 15 pre-larvae were taken from the single CubeSat exposed to simulated microgravity and from the control CubeSat. All samples were flash-frozen in liquid nitrogen and stored at −80 °C for later RNA extraction. The sampling plan is described in Table 3.
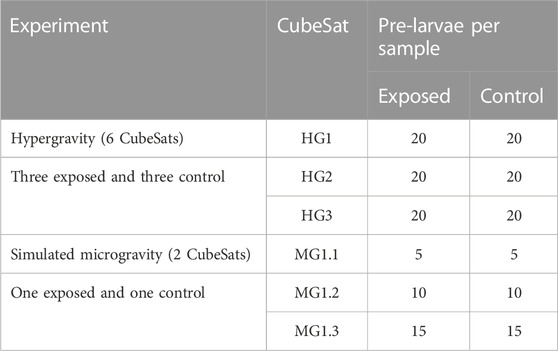
TABLE 3. Sampling plan for biomarker analysis. A single aliquot was sampled from each CubeSat exposed to hypergravity (HG1; HG2; HG3) and from each control CubeSat. For the simulated microgravity experiment, three aliquots were sampled from the single CubeSat (MG1) exposed to microgravity and from the control.
The available larvae for post-hatching analysis in the single SMGE group (n = 100) were more limited than in the HGE group in triplicate (n = 300), as the sampling size was different in the two independent experiments. However, the number of individual larvae sampled and the control were symmetric.
Extraction and reverse transcription of total RNA from fish samples
Total RNA was extracted from the larvae subjected to hypergravity conditions, simulated microgravity conditions and control conditions using the Nucleospin® RNA Plus XS kit (Macherey-Nagel, Düren, Germany). RNA integrity analysis was performed using RNA ScreenTapes and a TapeStation 4,150 device (Agilent, Santa Clara, California, United States). From each sample, 200 ng of total RNA was reverse transcribed using random primers, dNTP, RNaseOut and MML-V reverse transcriptase (all from Invitrogen, Cergy Pontoise, France) following the manufacturer’s instructions.
Quantitative PCR (qPCR)
Quantitative PCRs were performed in duplicate for a triplicate of samples (Table 3) using Takyon No ROX SYBR MasterMix dTTP Blue (Eurogentec, Liège, Belgium) and a Mastercycler Realplex2 Real-Time PCR system (Eppendorf, Hamburg, Germany). Primers (Eurogentec, Liège, Belgium) used to amplify transcripts were designed in different exons or in junctions between exons to preclude the amplification of potential traces of genomic DNA. Primer specificity was checked using the Basic Local Alignment Search Tool (BLAST) of the US National Center for Biotechnology Information (Bethesda, Maryland, United States). The qPCR cycling program was: 5 min at 95 °C followed by 40 cycles of 15 s at 95 °C and 45 s at the annealing temperature indicated in Table 4. Amplification efficiency was checked with standard curves and validated in the range of 89%–100%. The stability of housekeeping transcripts was checked using BestKeeper software (Pfaffl, 2001). Relative expression of glucocorticoid receptors 1 and 2 (GR1, GR2), Hsp90α, C3 and IL-1β was normalized using two housekeeping transcripts (Fau, which encodes a ubiquitin-like protein fused to the ribosomal S30 protein, and the ribosomal protein L13) using a method previously described (Fonte et al., 2019) (Table 4).
Statistics
As the hatching rate data was expressed in percentages and close to the extreme [0; 1], statistical analysis was performed with a converted value using
Ethical statement
The experiments at Ifremer and GEPAM were carried out in accordance with the recommendations of European Union Ethical Guidelines and directive 2010/63/EU on the protection of animals used for scientific purposes. The Ifremer Marine Experimental Platform of Palavas (PEMP) permit for animal experimentation E 34-192-006 and the site’s regional ethics committee (CEEA36 - Occitanie Méditerranée) guarantee the respect of handling rules by the scientific team.
Results and discussion
Embryogenesis duration and hatching rate
From egg fertilization to the end of the experiments, the water incubation temperature was kept at 14.5±1.2 °C in the hypergravity (HGE) conditions and 16.5±1.8 °C in the microgravity (SMGE) conditions. This temperature difference was due to the absence of thermoregulation in the SMGE room, and it is likely to explain why larvae were observed and counted at different times for HGE (at 96 hpf) and SMGE (at 72 hpf). Embryogenesis time is directly related to incubation temperature. Devauchelle and Coves (1988) put forward a robust model to predict European seabass egg hatching date based on incubation temperature, and this model has been regularly confirmed (Saka et al., 2001; Cucchi et al., 2012; Przybyla et al., 2020). The model accurately predicted the HGE hatching time within 1 hour. In the SMGE conditions, the European seabass eggs hatched 6 h before the expected time, which is in line with previous observations of killifish in microgravity (Scheld et al., 1976; Lychakov, 2016).
Hatching rates in other species have been previously evaluated during LEO missions: for example, with aquarium fish (n = 50) as part of the Skylab3 mission the hatching rate was 96%, and with killifish (n = 50) on the Apollo-Soyuz mission it was 22% (Hoffman et al., 1977; von Baumgarten et al., 1975). In later missions, the hatching rates recorded for medaka were 88% (n = 48) and 25% (n = 4) (Ijiri, 1998; Niihori et al., 2004). Results from our 10-min 5 g hypergravity experiment with European seabass eggs showed no significant difference compared with a control reared under optimal industrial conditions (Figure 3). The group exposed to simulated microgravity for 39 h recorded the same range of hatching performance as the industrial control group. Because we had access to only one RPM, only two hatching rates were assessed (exposed and control) for the simulated microgravity experiment, so this result should be considered to indicate a tendency rather than as robust data. For an unexplained reason, the simulated microgravity control group in the RPM room had a bad hatching percentage, which cannot be related to parental genetic inheritance because all the embryos were siblings.
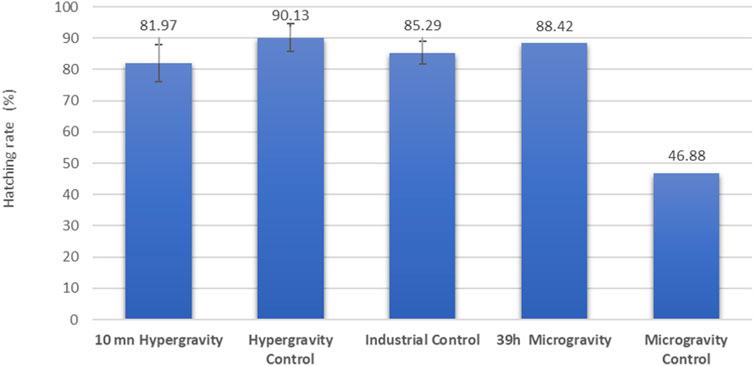
FIGURE 3. European seabass (Dicentrarchus labrax) hatching rate assessment of a family of siblings under simulated altered gravity and industrial control conditions. In the hypergravity experiment, three CubeSats were exposed for 10 min and three controls were unexposed (n = 100 fish embryos each). In the microgravity experiment, a single CubeSat (n = 100 embryos) was exposed to simulated microgravity for 39 h, and the control group (n = 100 embryos) was unexposed. The industrial control was raised in optimal ground-based aquaculture conditions. Results are expressed as mean ± SD for the hypergravity experiment (n = 3).
Oxygen consumption in microgravity
The initial water used to fill the CubeSat containing the fish eggs was over-oxygenated to ensure a sufficient oxygen level for the entire experiment (50 h) in total confinement in the CubeSat. Despite the precautions taken, the initial saturation was slightly different between the exposed group (248.7%) and the control group (240.2%) at the beginning of the experiment. Nonetheless, oxygen concentration inside the two sealed tanks was never under 146% of air saturation, and the cause of mortality of any unhatched eggs in the experiment cannot be attributed to lack of oxygen. Oxygen concentration kinetics during confinement before exposure and during the microgravity experiment are presented in Figure 4.
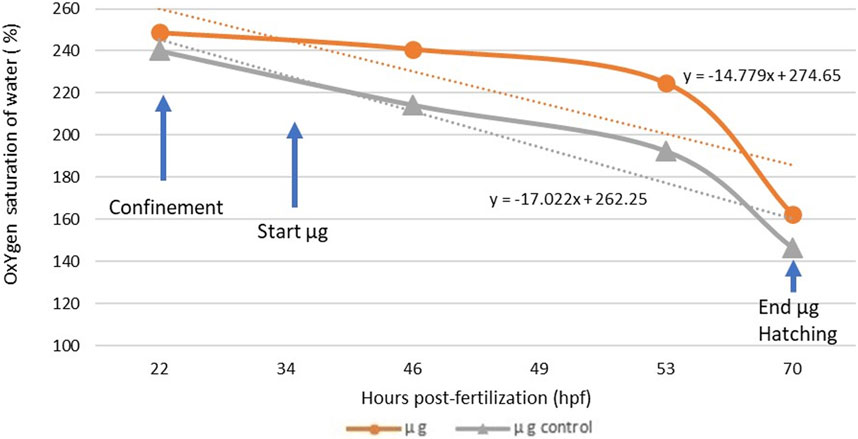
FIGURE 4. Oxygen concentration inside the CubeSat for the exposed group (n = 100 embryos) and the control group (n = 100 embryos) and trend curves during the simulated microgravity experiment. The difference between the slope of the microgravity and control group is not significant (p = 0.8074).
The slopes of the trend curves of the exposed group and the control group were not significantly different (p = 0.8074), suggesting that the microgravity environment did not generate an additional biological demand in oxygen during embryonic development. Furthermore, a calculation of individual oxygen consumption based on the oxygen volume consumed during the experiment and the surviving fish (live embryos and hatched larvae) in the two groups revealed that the embryos in microgravity did not consume more oxygen than the control group. The number of dead eggs (thus unhatched) was higher in the control group (52.08%) than in the exposed group (11.58%). We were unable to check when exactly the mortality occurred, before or during the microgravity exposure. Oxygen consumption values in the SMGE were consistent with those observed in a previous study with Dicentrarchus labrax (Pionetti, 1983) and other marine species (Table 5). Statistically, individual egg oxygen consumption did not differ between exposed and control groups in simulated microgravity.
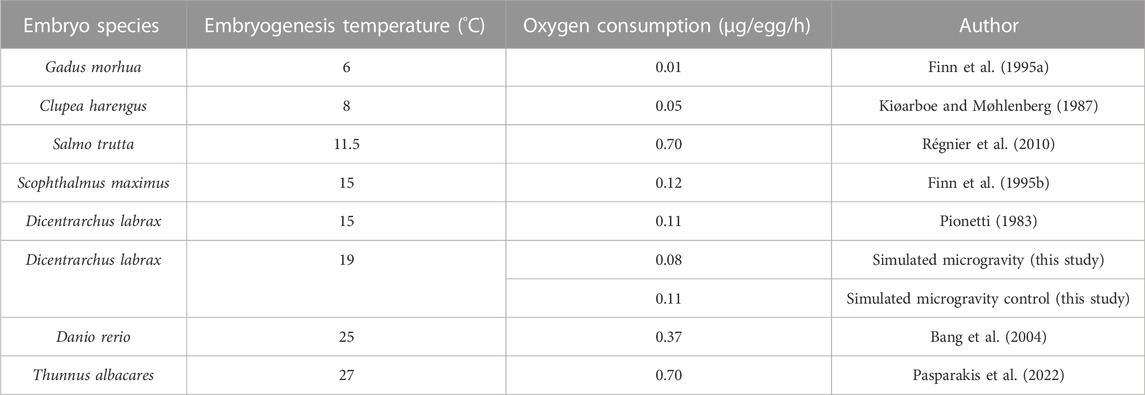
TABLE 5. Individual oxygen consumption calculation (µg/egg/h) in the simulated microgravity experiment compared to other species according to water temperature.
RNA integrity number
In order to assess possible degradation during altered gravity exposure, the RNA integrity number (RIN) was estimated. RNA plays a central role in reading the information encoded in the genome and transferring it into proteins. We used the RNA integrity number (RIN) as a diagnostic tool to estimate the quality of the samples, as it has been successfully used as an indicator of good RNA transcription and larvae development at hatching in other studies (Schroeder et al., 2006). RIN values range from 10 (intact) to 1 (totally degraded). Figure 5 shows that 10 min spent at a hypergravity of 5 g slightly but significantly decreased the RNA integrity number (p-value = 0.009), while 39 h of simulated microgravity did not significantly affect it.
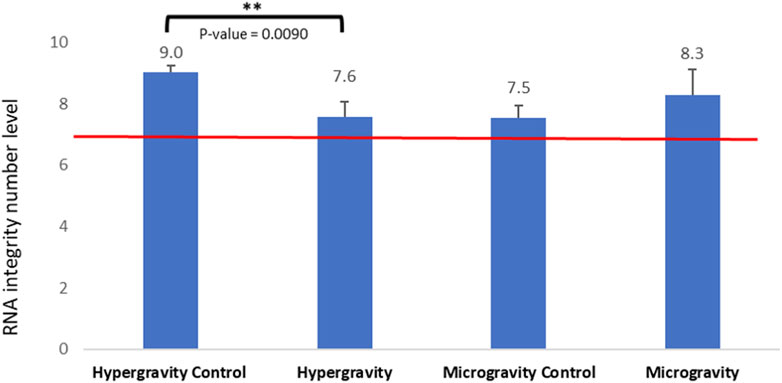
FIGURE 5. Effects of exposure to hypergravity (10 min at 5g) and simulated microgravity (39 h on the RPM) on the mean values of the RNA integrity number (RIN) of Dicentrarchus labrax embryos. The size of the replicates per condition is detailed in Table 3. Above the red line, the level of RNA integrity is considered good. Results are expressed as mean value ± SD. The statistically significant difference was found using a Student’s t-test. **p < 0.01.
In this study, all RIN values obtained were above 7.0, which is considered to be RNA of high quality in the zebrafish Danio rerio (Mazzolini et al., 2018), red seabream Pagrus major (Nakatsuji et al., 2019) and gilthead seabream Sparus aurata (Vallejos-Vidal et al., 2022). While the hypergravity experiment showed a significant influence on RNA integrity, the RIN mean value (7.6 ±0.4) stayed in the high-quality range and was not different from the control of the microgravity experiment. This observation correlates, from a physiological point of view, to the good hatching rate in the HGE group (exposed 81.97%± 5.96 and control group 90.13%± 4.36), suggesting that the environmental change from a hypergravity of 1g–5 g in 10 min for embryos at 49 hpf had no deleterious impact on hatched larvae of European seabass. In the microgravity experiment, the RIN mean value was not significantly different from the control, suggesting that mRNA integrity and the transcriptomic mechanism in European seabass embryos were not altered by 39 h of simulated microgravity from 34 hpf to hatching time at 96 hpf. This observation correlates to the good hatching rate in the SMGE group (88.42%).
Fish stress assessment in simulated altered gravity
Glucocorticoid receptors and chaperone protein
Previous studies on S. aurata juveniles (body weight 150 g) showed that GR1 expression can be significantly different 6 h post-stress (Vallejos-Vidal et al., 2022). In our experimental session, the sampling of HGE larvae was done 47 h after centrifuge exposure, so it is not surprising that glucocorticoid receptors had recovered to the control level (Figure 6). This indicates that the regulation of glucocorticoid receptor transcripts during European seabass embryogenesis was not impaired by the hypergravity simulation of a lunar launch at an early development stage.
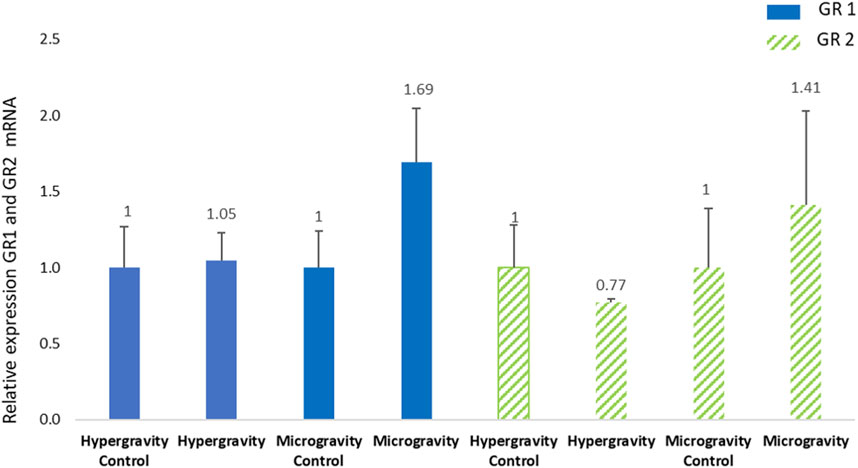
FIGURE 6. Effects of exposure of European seabass (Dicentrarchus labrax) to hypergravity (10 min at 5g) or simulated microgravity (39 h on an RPM) on glucocorticoid receptors 1 and 2 (GR1, GR2) mRNA levels. The size of the replicates per condition is detailed in Table 3. Relative values obtained with control embryos were set to 1. Results are expressed as mean ± SD.
The relative expression of GR1 and GR2 in Dicentrarchus labrax larvae after 39 h of exposure to simulated microgravity (sampled around 4 h after hatching) revealed no significant difference in the receptor involved in cortisol regulation. Hatching occurred at 96 hpf and the fish embryos (n = 100) spent 39 h inside a sealed CubeSat on the RPM, which represents 41% of the total embryo development. We did not observe a significant difference between the expression of GR1 and GR2 at the post-hatching stage, in contrast to a study on European seabass ontogeny without stress (Pavlidis et al., 2011) or a study in which a higher sensitivity response of GR2 than GR1 to pathogenic stress was observed at a juvenile stage (Reyes-López et al., 2018). Our results showed the same level of expression of the cortisol receptors in the exposure and control conditions, suggesting that the simulated altered gravity did not influence European seabass embryogenesis.
Hsp90α chaperone protein activity is directly related to the function of GR1 and GR2. No statistically significant difference in Hsp90α mRNA expression was observed in either experiment (Figure 7).
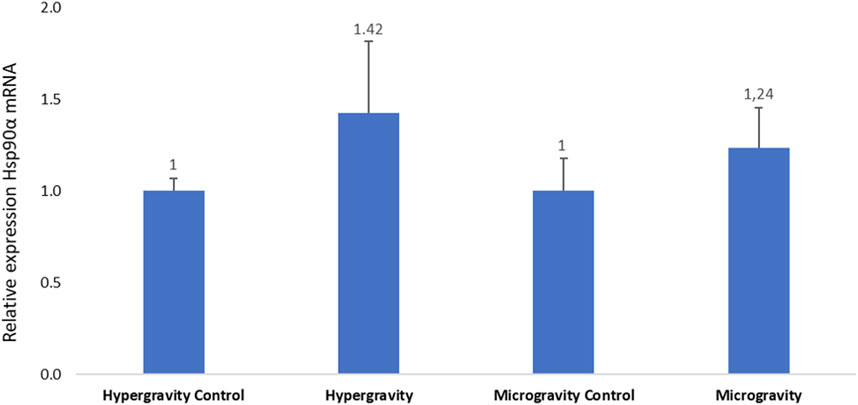
FIGURE 7. Effects of exposure to hypergravity (10 min at 5g) or simulated microgravity (39 h on an RPM) on Dicentrarchus labrax Hsp90a mRNA levels. The size of the replicates per condition is detailed in Table 3. Relative values obtained with control embryos were set to 1. Results are expressed as mean ± SD.
Madeira et al. (2016) exposed 35-day post-hatch Sparus aurata larvae to abiotic stress based on temperature variation and detected an increase in Hsp90α expression. In the European seabass, a downregulation of GR1 and GR2 was observed in the hypothalamus of fish submitted to relatively low rearing density (Geffroy et al., 2021). In our study, we did not observe a significant variation in GR1, GR2 and Hsp90α relative to control groups. The same level of transcription between the glucocorticoid receptors and its chaperone protein was observed, which indicates that our measurements were robust and allows us to conclude that our experimental altered gravity conditions did not affect this physiological route of stress in European seabass embryos.
Innate immune system: Proteins C3 and IL-1β
In vertebrates, innate immunity is a non-specific defense system. This mechanism is involved in identifying and fighting external aggressions. Our study investigated the function of this immune system under microgravity and extreme hypergravity conditions. The results showed variable relative C3 expression, which was highly significantly different from the 1 g control (Figure 8). Studies on C3 in gilthead seabream and European seabass juveniles (weighing around 30 g) suggest that a sampling plan 6 < n < 10 is relevant to obtain good C3 analysis sensitivity (Mauri et al., 2011; Vaz et al., 2022). In our study, tissue samples were smaller, but the number of larvae per pool was 5 < n < 15 for the microgravity experiment and n = 20 for the hypergravity experiment. We therefore assume that threshold detection is enough to validate our measurements.
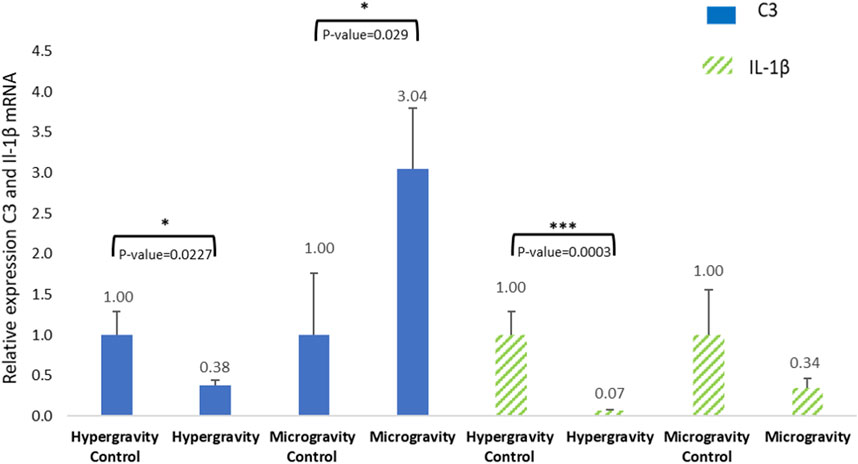
FIGURE 8. Effects of exposure to hypergravity (10 min at 5 g) or microgravity (39 h on an RPM) on C3 and IL-1β mRNA levels in Dicentrarchus labrax. The size of the replicates per condition is detailed in Table 3. Relative values obtained with control embryos were set to 1. Results are expressed as mean ± SD. Statistically significant differences were found using Student’s t-tests. *p < 0.05, ***p < 0.001.
In experimental conditions, Lange et al. (2004) detected the presence of C3 in cod larvae (Gadus morhua L.) in the yolk sac membrane and in muscle at 1-day post-hatching. Olive flounder (Paralichthys olivaceus) expressed significant C3 protein at hatching compared to the day before (embryo stage) and the day after (Lee et al., 2013). C3 expression was also monitored on rainbow trout (Oncorhynchus mykiss) and Atlantic salmon (Salmo salar) during the embryo to larval stage. All C3 isoform expression drastically increased just after hatching (Løvoll et al., 2006; Løvoll et al., 2007). These observations are in line with our microgravity group result, but not with the hypergravity group.
The literature on C3 protein expression in European seabass hatchlings is poor, so we cannot make a confident conclusion about the significant C3 inhibition observed in the HGE group. However, this difference in C3 relative expression had no consequence on the good hatching rate recorded in the HGE group (81.97%±5.96) and the SMGE group (88.42%). The study found that the complement protein C3 is expressed in Dicentrarchus labrax at the hatchling stage 4 h after leaving the chorion, suggesting that part of the innate system is functional in both altered gravity contexts.
Interleukin-1β is produced by active macrophages. It is involved in infection and inflammation, but can also play a role in the acute stress response, as has been shown in the common carp Cyprinus carpio (Metz et al., 2006). Its expression was observed in European seabass 12 h following an infection by Vibrio anguillarum 5 days after hatching (Reyes-López et al., 2018). The overexpression of IL-1β in European seabass juveniles can appear 1.5 h–8 h after a V. anguillarum infection (Meloni et al., 2015). Figure 8 shows that 10 min spent at a hypergravity of 5 g significantly decreased the expression level of IL-1β mRNA. IL-1β was detected at 4 hpf, indicating the activation of the inflammatory innate immune system in both altered gravities. Yet the low level of IL-1β confirms that altered gravity does not induce inflammation or stress. The innate immune system has been studied in several fish species, but ours is the first analysis of C3 and IL-1β in just-hatched European seabass. Our findings indicate that C3 protein and IL-1β can be measured 4 hours after this species hatches. As there was a good hatching rate and given the vitality of the young larvae, the significant inhibition of complement C3 and IL-1β in the hypergravity groups cannot be linked to abnormal development or a deleterious innate immune system. The underexpression of C3 and IL-1β confirm an absence of inflammatory or physiological damage after altered gravity exposure, but may also reveal a delay in the activation of the pro-inflammatory defense mechanism. It would be valuable to study the expression of these proteins several days after hatching to verify the development of the immune system in conditions of altered gravity.
Conclusion
This prospective study indicates that the mechanism of embryogenesis in an aquaculture fish can follow normal development under simulated hyper- and microgravity conditions. Further investigation of D. labrax’s innate immune system at 1 g and hyper- and microgravity conditions at larval stage could confirm our hypothesis that this system is intact after altered gravity exposure. We carried out our experiments separately to allow results, analysis and conclusions in mono-parametric situations for each gravity context. A further ground-based study could involve a larger number of embryos (hence more tissue to sample) for simulated microgravity in order to consolidate our hypothesis about the absence of effect on fish stress and the innate immune system. Successive exposure to 10-min hypergravity (launch duration) and microgravity (for as long as possible) should also be tested in a future experiment to understand the cumulative effect of these conditions on aquaculture fish embryos. The shortest manned mission to reach the Moon was Apollo 8 (69 h), but the mean time this journey takes is around 72 h; this means our 39-h microgravity experiment corresponds to only half (54%) of a standard trip to the Moon. Following a previous study on European seabass and meagre (Argyrosomus regius) fertilized egg resistance to spacecraft-launcher vibration (Przybyla et al., 2020), this study strengthens the idea of the feasibility of shipping aquaculture fish eggs to a lunar base in order to develop space aquaculture integrated in an agricultural greenhouse or a water treatment system (Pickett et al., 2020). Cultivated aquatic organisms could provide astronauts with amino acids to complement plant sources and offer a more balanced diet. Beyond the nutritional aspect, the variety, texture and flavor of aquaculture species could help to avoid “menu fatigue” in space due to repeated consumption of the same foods and the resulting body mass loss (Douglas et al., 2020). The results of this study allow optimism and warrant envisaging a real proof of concept to validate the findings by sending aquaculture fish on a space mission in low Earth orbit or, even more valuably, on a lunar mission.
Data availability statement
The raw data supporting the conclusion of this article will be made available by the authors, without undue reservation.
Author contributions
CP Writer and manager GRAVITY Project JB GEPAM facility management experimental hyper and micro gravity support–Biomarker paragrph author. RP Student experiment management and paragraph writer AD Student pre-experiment management and paragraph writer GD Fish egg expert and protocol management EM Fish egg expert, logistic and experimental biologist SL Broodstock manager, eggs supplier and maintenance FC Biomarkers analysis BG Fish stress expert and sampling protocol manager. JF Senior expert in gravity experiment. All authors contributed to the article and approved the submitted version.
Funding
The “GRAVITY project” was funded by the French Space Agency (APR-CNES ID Action 5994 - contract 4800001083) and the French National Institute for Ocean Science (IFREMER scientific directorate R120-06-DF-01). The experiment’s access to the GEPAM facility was funded by the European Space Agency through the Continuously Open Research Announcement Opportunity for Ground Based Facilities program (Contract 4000137191/21/NL/PA/pt).
Acknowledgments
The first author would like to sincerely thank Véronique Ribière for the video production detailing the stages of the study and her support for the Lunar Hatch project, and Benoit Rollin for the design and construction of the nine CubeSat modules. All co-authors are grateful to the administrative and logistics staff from the Ifremer and GEPAM laboratories involved in this project, and to the European Space Agency (ESA), the French Space Agency (CNES) and Ifremer, which made this experiment possible.
Conflict of interest
The authors declare that the research was conducted in the absence of any commercial or financial relationships that could be construed as a potential conflict of interest.
Publisher’s note
All claims expressed in this article are solely those of the authors and do not necessarily represent those of their affiliated organizations, or those of the publisher, the editors and the reviewers. Any product that may be evaluated in this article, or claim that may be made by its manufacturer, is not guaranteed or endorsed by the publisher.
Supplementary material
The video of the GRAVITY experiment is available on the following site: https://www.youtube.com/channel/UCRAUiMnmFkpyDmw9IIWwiWA.
Abbreviations
hpf, Hours post fertilization; HGE, Hypergravity experiment; SMGE, Simulated microgravity experiment; RPM, Random positioning machine; LEO, Low Earth orbit; RIN, RNA integrity number; 1g, Conventional unit of gravitational acceleration on Earth; BLSS, Bioregenerative life-support system; ECLS, Environmental control and life-support system; GR, Glucocorticoid receptor; GEPAM, Gravitational Experimental Platform for Animal Models; RAS, Recirculating aquaculture system; PSU, Practical salinity unit.
References
Aceto, J., Nourizadeh-Lillabadi, R., Maree, R., Dardenne, N., Jeanray, N., Wehenkel, L., et al. (2015). Zebrafish bone and general physiology are differently affected by hormones or changes in gravity. Plos One 10, e0126928. doi:10.1371/journal.pone.0126928
Anken, R., Brungs, S., Grimm, D., Knie, M., and Hilbig, R. (2016). Fish inner ear otolith growth under real microgravity (spaceflight) and clinorotation. Microgravity Sci. Technol. 28, 351–356. doi:10.1007/s12217-015-9459-4
Anken, R. H., Ibsch, M., and Rahmann, H. (1998). Neurobiology of fish under altered gravity conditions. Brain Res. Rev. 28, 9–18. doi:10.1016/s0165-0173(98)00021-6
Anken, R., and Hilbig, R. (2009). Swimming behaviour of the upside-down swimming catfish (Synodontis nigriventris) at high-quality microgravity - a drop-tower experiment. Adv. Space Res. 44, 217–220. doi:10.1016/j.asr.2009.02.009
Bang, A., Gronkjaer, P., and Malte, H. (2004). Individual variation in the rate of oxygen consumption by zebrafish embryos. J. Fish Biol. 64, 1285–1296. doi:10.1111/j.0022-1112.2004.00391.x
Black, S., Larkin, K., Jacqmotte, N., Wassersug, R., Pronych, S., and Souza, K. (1995). “Regulative development of Xenopus laevis in microgravity,” in Life and gravity: Physiological and morphological responses. Editors P. Todd, H. J. Anton, P. W. Barlow, R. Gerzer, J. M. Heim, R. HemmersbachKrauseet al. 209–217.
Bonnefoy, J., Ghislin, S., Beyrend, J., Coste, F., Calcagno, G., Lartaud, I., et al. (2021). Gravitational experimental platform for animal models, a new platform at ESA’s terrestrial facilities to study the effects of micro- and hypergravity on aquatic and rodent animal models. Int. J. Mol. Sci. 22, 2961. doi:10.3390/ijms22062961
Brungs, S., Hauslage, J., Hilbig, R., Hemmersbach, R., and Anken, R. (2011). Effects of simulated weightlessness on fish otolith growth: clinostat versus rotating-wall vessel. Adv. Space Res. 48, 792–798. doi:10.1016/j.asr.2011.04.014
Brydon, L., Edwards, S., Jia, H., Mohamed-Ali, V., Zachary, I., Martin, J. F., et al. (2005). Psychological stress activates interleukin-1β gene expression in human mononuclear cells. Brain Behav. Immun. 19, 540–546. doi:10.1016/j.bbi.2004.12.003
Buchheim, J. I., Matzel, S., Rykova, M., Vassilieva, G., Ponomarev, S., Nichiporuk, I., et al. (2019). Stress related shift toward inflammaging in cosmonauts after long-duration space flight. Front. Physiology 10, 85. doi:10.3389/fphys.2019.00085
Cooper, M., Perchonok, M., and Douglas, G. L. (2017). Initial assessment of the nutritional quality of the space food system over three years of ambient storage. Npj Microgravity 3, 17. doi:10.1038/s41526-017-0022-z
Crucian, B. E., Zwart, S. R., Mehta, S., Uchakin, P., Quiriarte, H. D., Pierson, D., et al. (2014). Plasma cytokine concentrations indicate that in vivo hormonal regulation of immunity is altered during long-duration spaceflight. J. Interferon Cytokine Res. 34, 778–786. doi:10.1089/jir.2013.0129
Cucchi, P., Sucre, E., Santos, R., Leclere, J., Charmantier, G., and Castille, R. (2012). Embryonic development of the sea bass Dicentrarchus labrax. Helgol. Mar. Res. 66, 199–209. doi:10.1007/s10152-011-0262-3
Devauchelle, N., and Coves, D. (1988). The characteristics of sea bass (Dicentrarchus labrax) eggs: description, biochemical composition and hatching performances. Aquat. Living Resour. 1, 223–230. doi:10.1051/alr:1988022
Di Bella, M. L., Vazzana, M., Vizzini, A., and Parrinello, N. (2008). Glucocorticoid receptor (DlGR1) is expressed in pre-larval and larval stages of the teleost fish Dicentrarchus labrax. Cell Tissue Res. 333, 39–47. doi:10.1007/s00441-008-0605-9
Douglas, G. L., Zwart, S. R., and Smith, S. M. (2020). Space food for thought: challenges and considerations for food and nutrition on exploration missions. J. Nutr. 150, 2242–2244. doi:10.1093/jn/nxaa188
Edsall, S. C., and Franz-Odendaal, T. A. (2014). An assessment of the long-term effects of simulated microgravity on cranial neural crest cells in zebrafish embryos with a focus on the adult skeleton. Plos One 9, e89296. doi:10.1371/journal.pone.0089296
Endo, M., Kobayashi, R., Ariga, K., Yoshizaki, G., and Takeuchi, T. (2002). Postural control in tilapia under microgravity and the near infrared irradiated conditions. Nippon. Suisan Gakkaishi 68, 887–892. doi:10.2331/suisan.68.887
Fahrion, J., Mastroleo, F., Dussap, C.-G., and Leys, N. (2021). Use of photobioreactors in regenerative life support systems for human space exploration. Front. Microbiol. 12, 699525. doi:10.3389/fmicb.2021.699525
Finn, R. N., Fyhn, H. J., and Evjen, M. S. (1995a). Physiological energetics of developing embryos and yolk-sac larvae of Atlantic cod (Gadus morhua). I. Respiration and nitrogen metabolism. Mar. Biol. 124, 355–369. doi:10.1007/bf00363909
Finn, R. N., Widdows, J., and Fyhn, H. J. (1995b). Calorespirometry of developping embryos and yolk-sac larvae of turbot (Scophtalmus maximus). Mar. Biol. 122, 157–163. doi:10.1007/bf00349289
Fonte, C., Kaminski, S., Vanet, A., Lanfumey, L., Cohen-Salmon, C., Ghislin, S., et al. (2019). Socioenvironmental stressors encountered during spaceflight partially affect the murine TCR-β repertoire and increase its self-reactivity. Faseb J. 33, 896–908. doi:10.1096/fj.201800969r
Geffroy, B., Gesto, M., Clota, F., Aerts, J., Darias, M. J., Blanc, M. O., et al. (2021). Parental selection for growth and early-life low stocking density increase the female-to-male ratio in European sea bass. Sci. Rep. 11, 13620. doi:10.1038/s41598-021-93116-9
Goikoetxea, A., Sadoul, B., Blondeau-Bidet, E., Aerts, J., Blanc, M.-O., Parrinello, H., et al. (2021). Genetic pathways underpinning hormonal stress responses in fish exposed to short- and long-term warm ocean temperatures. Ecol. Indic. 120, 106937. doi:10.1016/j.ecolind.2020.106937
Hemmersbach, R., Häder, D.-P., and Braun, M. (2018). “Methods for gravitational biology research,” in Gravitational biology I: Gravity sensing and graviorientation in microorganisms and plants. Editors M. Braun, M. Böhmer, D.-P. Häder, R. Hemmersbach, and K. Palme (Cham: Springer International Publishing), 13–26.
Hermannsdottir, R., Johannsdottir, J., Smaradottir, H., Sigurgisladottir, S., Gudmundsdottir, B. K., and Bjornsdottir, R. (2009). Analysis of effects induced by a pollock protein hydrolysate on early development, innate immunity and the bacterial community structure of first feeding of Atlantic halibut (Hippoglossus hippoglossus L.) larvae. Fish Shellfish Immunol. 27, 595–602. doi:10.1016/j.fsi.2009.05.007
Herranz, R., Anken, R., Boonstra, J., Braun, M., Christianen, P. C., de Geest, M., et al. (2013). Ground-based facilities for simulation of microgravity: organism-specific recommendations for their use, and recommended terminology. Astrobiology 13, 1–17. doi:10.1089/ast.2012.0876
Hilbig, R. W., and Anken, R. H. (2017). Impact of micro- and hypergravity on neurovestibular issues of fish. Sensory Motor and Behavioral Research in Space, 59–86.
Hoffman, R. B., Salinas, G. A., and Baky, A. A. (1977). Behavioral analyses of killifish exposed to weightlessness in the Apollo-Soyuz test project. Space Environ. Med. 48, 712–717.
Ijiri, K. (1998). Development of space-fertilized eggs and formation of primordial germ cells in the embryos of Medaka fish. Adv. Space Res. 21, 1155–1158. doi:10.1016/s0273-1177(97)00205-6
Irons, L. G., and Irons, M. A. (2023). Pancosmorio (world limit) theory of the sustainability of human migration and settlement in space. Front. Astronomy Space Sci. 10. doi:10.3389/fspas.2023.1081340
Kiøarboe, T., and Møhlenberg, F. (1987). Partitioning of oxygen consumption between “maintenance” and “growth” in developing herring Clupea harengus (L.) embryos. J. Exp. Mar. Biol. Ecol. 111, 99–108. doi:10.1016/0022-0981(87)90048-7
Krieger, S. S., Zwart, S. R., Mehta, S., Wu, H. L., Simpson, R. J., Smith, S. M., et al. (2021). Alterations in saliva and plasma cytokine concentrations during long-duration spaceflight. Front. Immunol. 12, 725748. doi:10.3389/fimmu.2021.725748
Lange, S., Bambir, S., Dodds, A. W., and Magnadóttir, B. (2004). The ontogeny of complement component C3 in atlantic cod (Gadus morhua L.)—An immunohistochemical study. Fish Shellfish Immunol. 16, 359–367. doi:10.1016/j.fsi.2003.06.001
Lee, J. W., Lee, Y. M., Lee, J. H., Noh, J. K., Kim, H. C., Park, C. J., et al. (2013). The expression analysis of complement component C3 during early developmental stages in olive flounder (Paralichthys olivaceus). Dev. Reprod. 17, 311–319. doi:10.12717/dr.2013.17.4.311
Løvoll, M., Johnsen, H., Boshra, H., Bogwald, J., Sunyer, J. O., and Dalmo, R. A. (2007). The ontogeny and extrahepatic expression of complement factor C3 in Atlantic salmon (Salmo salar). Fish Shellfish Immunol. 23, 542–552. doi:10.1016/j.fsi.2007.01.002
Løvoll, M., Kilvik, T., Boshra, H., Bogwald, J., Sunyer, J. O., and Dalmo, R. A. (2006). Maternal transfer of complement components C3-1, C3-3, C3-4, C4, C5, C7, Bf, and Df to offspring in rainbow trout (Oncorhynchus mykiss). Immunogenetics 58, 168–179. doi:10.1007/s00251-006-0096-3
Lychakov, D. V. (2016). Behavioural and functional vestibular disorders after space flight: 2. Fish, amphibians and birds. J. Evol. Biochem. Physiology 52, 1–16. doi:10.1134/s0022093016010014
Madeira, D., Araujo, J. E., Vitorino, R., Capelo, J. L., Vinagre, C., and Diniz, M. S. (2016). Ocean warming alters cellular metabolism and induces mortality in fish early life stages: A proteomic approach. Environ. Res. 148, 164–176. doi:10.1016/j.envres.2016.03.030
Magnadóttir, B. (2006). Innate immunity of fish (overview). Fish Shellfish Immunol. 20, 137–151. doi:10.1016/j.fsi.2004.09.006
Mastellos, D. C., DeAngelis, R. A., and Lambris, J. D. (2013). Complement-triggered pathways orchestrate regenerative responses throughout phylogenesis. Seminars Immunol. 25, 29–38. doi:10.1016/j.smim.2013.04.002
Mauri, I., Roher, N., MacKenzie, S., Romero, A., Manchado, M., Balasch, J. C., et al. (2011). Molecular cloning and characterization of European seabass (Dicentrarchus labrax) and Gilthead seabream (Sparus aurata) complement component C3. Fish Shellfish Immunol. 30, 1310–1322. doi:10.1016/j.fsi.2011.03.013
Mazzolini, J., Chia, K., and Sieger, D. (2018). Isolation and RNA extraction of neurons, macrophages and microglia from larval zebrafish brains. Jove-Journal Vis. Exp., 57431. doi:10.3791/57431
Meloni, M., Candusso, S., Galeotti, M., and Volpatti, D. (2015). Preliminary study on expression of antimicrobial peptides in European sea bass (Dicentrarchus labrax) following in vivo infection with Vibrio anguillarum. A time course experiment. Fish Shellfish Immunol. 43, 82–90. doi:10.1016/j.fsi.2014.12.016
Metz, J. R., Huising, M. O., Leon, K., Verburg-van Kemenade, B. M. L., and Flik, G. (2006). Central and peripheral interleukin-1β and interleukin-1 receptor I expression and their role in the acute stress response of common carp, Cyprinus carpio L. J. Endocrinol. 191, 25–35. doi:10.1677/joe.1.06640
Nakatsuji, N., Adachi, K., and Morioka, K. (2019). Long-term stability of RNA isolated from muscle of red seabream (Pagrus major) during ice storage. Fish Physiology Biochem. 45, 819–828. doi:10.1007/s10695-018-0588-8
Najafpour, B., Cardoso, J. C. R., Canario, A. V. M., and Power, D. M. (2020). Specific evolution and gene family expansion of complement 3 and regulatory factor H in fish. Front. Immunol. 11, 568631. doi:10.3389/fimmu.2020.568631
Niihori, M., Mogami, Y., Naruse, K., and Baba, S. A. (2004). Development and swimming behavior of medaka fry in a spaceflight aboard the space shuttle Columbia (STS-107). Zoological Sci. 21, 923–931. doi:10.2108/zsj.21.923
Palmisano, A. N., Winton, J. R., and Dickhoff, W. W. (2000). Tissue-specific induction of Hsp90 mRNA and plasma cortisol response in chinook salmon following heat shock, seawater challenge, and handling challenge. Mar. Biotechnol. 2, 329–338. doi:10.1007/s101260000005
Pasparakis, C., Wang, Y., Heuer, R. M., Zhang, W., Stieglitz, J. D., McGuigan, C. J., et al. (2022). Ultraviolet avoidance by embryonic buoyancy control in three species of marine fish. Sci. Total Environ. 806, 150542. doi:10.1016/j.scitotenv.2021.150542
Pavlidis, M., Karantzali, E., Fanouraki, E., Barsakis, C., Kollias, S., and Papandroulakis, N. (2011). Onset of the primary stress in European sea bass Dicentrarhus labrax, as indicated by whole body cortisol in relation to glucocorticoid receptor during early development. Aquaculture 315, 125–130. doi:10.1016/j.aquaculture.2010.09.013
Pfaffl, M. W. (2001). A new mathematical model for relative quantification in real-time RT-PCR. Nucleic Acids Res. 29, e45–e45. doi:10.1093/nar/29.9.e45
Pickett, M. T., Roberson, L. B., Calabria, J. L., Bullard, T. J., Turner, G., and Yeh, D. H. (2020). Regenerative water purification for space applications: needs, challenges, and technologies towards 'closing the loop. Life Sci. Space Res. 24, 64–82. doi:10.1016/j.lssr.2019.10.002
Pionetti, J.-M. (1983). The oxygen consumptionof sea bass Dicentrarchus labrax in relation to development. Oceanis 9, 5.
Poulet, L., Engeling, K., Hatch, T., Stahl-Rommel, S., Velez Justiniano, Y.-A., Castro-Wallace, S., et al. (2022). Large-scale crop production for the Moon and Mars: current gaps and future perspectives. Front. Astronomy Space Sci. 8. doi:10.3389/fspas.2021.733944
Przybyla, C., Dutto, G., Bernard, M., Rollin, B., Laurand, X., Averseng, J., et al. (2020). European sea bass (Dicentrarchus labrax) and meagre (Argyrosomus regius) fertilized egg resistance to a spacecraft launcher vibration qualifying test. Aquac. Int. 28, 2465–2479. doi:10.1007/s10499-020-00601-5
Przybyla, C. (2021). Space aquaculture: prospects for raising aquatic vertebrates in a bioregenerative life-support system on a lunar base. Front. Astronomy Space Sci. 8. doi:10.3389/fspas.2021.699097
Régnier, T., Bolliet, V., Labonne, J., and Gaudin, P. (2010). Assessing maternal effects on metabolic rate dynamics along early development in brown trout (Salmo trutta): an individual-based approach. J. Comp. Physiology B 180, 25–31. doi:10.1007/s00360-009-0385-x
Reyes-López, F. E., Aerts, J., Vallejos-Vidal, E., Ampe, B., Dierckens, K., Tort, L., et al. (2018). Modulation of innate immune-related genes and glucocorticoid synthesis in gnotobiotic full-sibling European sea bass (Dicentrarchus labrax) larvae challenged with Vibrio anguillarum. Front. Immunol. 9, 914. doi:10.3389/fimmu.2018.00914
Rutherford, S. L., and Lindquist, S. (1998). Hsp90 as a capacitor for morphological evolution. Nature 396, 336–342. doi:10.1038/24550
Sadoul, B., and Geffroy, B. (2019). Measuring cortisol, the major stress hormone in fishes. J. Fish Biol. 94, 540–555. doi:10.1111/jfb.13904
Saka, Ş., Fırat, M. K., and Kamaci, H. O. (2001). The development of European sea bass (Dicentrarchus labrax L., 1758) eggs in relation to temperature. Turkish J. Veterinary Animal Sci. 25, 139–147.
Salazar Moscoso, M., Joly Ruiz Castellanos, S., Anglada Escudé, G., and Ribas Cabezas, L. (2022). Hypergravity induces changes in physiology, gene expression and epigenetics in zebrafish, 4th Symposium on Space Educational Activities. Universitat Politècnica de Catalunya.
Scheld, H. W., Boyd, J. F., Bozarth, G. A., Conner, J., Eichler, V. B., Fuller, P. M., et al. (1976). Killifish hatching and orientation experiment MA-161.
Schroeder, A., Mueller, O., Stocker, S., Salowsky, R., Leiber, M., Gassmann, M., et al. (2006). The RIN: an RNA integrity number for assigning integrity values to RNA measurements. BMC Mol. Biol. 7, 3. doi:10.1186/1471-2199-7-3
Serradell, A., Torrecillas, S., Makol, A., Valdenegro, V., Fernández-Montero, A., Acosta, F., et al. (2020). Prebiotics and phytogenics functional additives in low fish meal and fish oil based diets for European sea bass (Dicentrarchus labrax): effects on stress and immune responses. Fish Shellfish Immunol. 100, 219–229. doi:10.1016/j.fsi.2020.03.016
Tacon, A. G. J., Lemos, D., and Metian, M. (2020). Fish for health: improved nutritional Quality of cultured fish for human consumption. Rev. Fish. Sci. Aquac. 28, 449–458. doi:10.1080/23308249.2020.1762163
Trout, O. F., and Bruchey, W. J. (1969). Water immersion reduced-gravity simulation. Hum. Factors 11, 473–487. doi:10.1177/001872086901100507
Tsalafouta, A., Papandroulakis, N., Gorissen, M., Katharios, P., Flik, G., and Pavlidis, M. (2014). Ontogenesis of the HPI axis and molecular regulation of the cortisol stress response during early development in Dicentrarchus labrax. Sci. Rep. 4, 5525. doi:10.1038/srep05525
Uribe, C., Folch, H., Enriquez, R., and Moran, G. (2011). Innate and adaptive immunity in teleost fish: a review. Veterinarni Med. 56, 486–503. doi:10.17221/3294-vetmed
Vallejos-Vidal, E., Sanz-Milián, B., Teles, M., Reyes-Cerpa, S., Mancera, J. M., Tort, L., et al. (2022). The gene expression profile of the glucocorticoid receptor 1 (gr1) but not gr2 is modulated in mucosal tissues of gilthead sea bream (Sparus aurata) exposed to acute air-exposure stress. Front. Mar. Sci. 9. doi:10.3389/fmars.2022.977719
Vaz, M., Pires, D., Pires, P., Simões, M., Pombo, A., Santos, P., et al. (2022). Early immune modulation in European seabass (Dicentrarchus labrax) juveniles in response to betanodavirus infection. Fishes 7, 63. doi:10.3390/fishes7020063
von Baumgarten, R. J., Simmonds, R. C., Boyd, J. F., and Garriott, O. K. (1975). Effects of prolonged weightlessness on the swimming pattern of fish aboard Skylab 3. Aviat. Space Environ. Med. 46, 902–906.
Wiederhold, M. L., Harrison, J. L., and Gao, W. Y. (2003). A critical period for gravitational effects on otolith formation. J. Vestib. Research-Equilibrium Orientat. 13, 205–214. doi:10.3233/ves-2003-134-605
Keywords: Complement component 3, European seabass, glucocorticoid receptors, heat shock protein 90, hypergravity, interleukin-1 beta, microgravity, space aquaculture
Citation: Przybyla C, Bonnefoy J, Paounov R, Debiol A, Dutto G, Mansuy E, Lallement S, Coste F, Geffroy B and Frippiat JP (2023) Embryogenesis of an aquaculture fish (Dicentrarchus labrax) under simulated altered gravity. Front. Space Technol. 4:1240251. doi: 10.3389/frspt.2023.1240251
Received: 14 June 2023; Accepted: 18 September 2023;
Published: 09 October 2023.
Edited by:
Parag Vaishampayan, National Aeronautics and Space Administration, United StatesReviewed by:
Holly Hyde Birdsall, Baylor College of Medicine, United StatesKhaled Y. Kamal, Texas A&M University, United States
Copyright © 2023 Przybyla, Bonnefoy, Paounov, Debiol, Dutto, Mansuy, Lallement, Coste, Geffroy and Frippiat. This is an open-access article distributed under the terms of the Creative Commons Attribution License (CC BY). The use, distribution or reproduction in other forums is permitted, provided the original author(s) and the copyright owner(s) are credited and that the original publication in this journal is cited, in accordance with accepted academic practice. No use, distribution or reproduction is permitted which does not comply with these terms.
*Correspondence: Cyrille Przybyla, Q3lyaWxsZS5wcnp5YnlsYUBpZnJlbWVyLmZy