- 1Department of Electrical and Computer Engineering, University of Missouri, Columbia, MO, United States
- 2Department of Physics and Astronomy, Texas Tech University, Lubbock, TX, United States
In this paper we discuss the advantages and challenges of utilizing Fission Fragment Rocket Engines (FFREs) to dramatically reduce transit time in space travel, for example, traveling to Mars. We discuss methods to decrease the size and weight of FFREs. These include utilizing metallic deuterides as moderators, driving the engines with electron beam bremsstrahlung, and operating the FFREs as subcritical assemblies, not as nuclear reactors. We discuss these and other new innovations based upon improved materials and technology that may be integrated into a revolutionary nuclear rocket technology.
1 Introduction
Since the 1950s, nuclear rockets were developed primarily at Los Alamos National Laboratory to produce faster methods for space travel. (Bussard and DeLauer, 1958; Dewar, 1974; Borowski, 1987; Dewar, 2007). These technologies utilize nuclear designs that transfer heat from a sealed core to liquid hydrogen expanders or thermionic converters in a conventional manner. Beginning in the 1980s, a more efficient nuclear energy transfer design emerged for rocketry, (Haslett, 1995; Lieberman, 1992), one which exposes the core for direct nuclear fragment thrust once the rocket is well outside the Earth’s atmosphere. From FY11 through FY14, the NASA Institute for Advanced Concepts studied the Fission Fragment Rocket Engine (FFRE). (Werka et al., 2012; Chapline, 1988; Chapline et al., 1988; Chapline and Matsuda, 1991). The FFRE would directly convert the momentum of fission fragments into spacecraft momentum at extremely high specific impulse (ISP). The ISP is a measure of how efficiently an engine uses fuel to create thrust. For rocketry, ISP is defined as the thrust integrated over time per unit weight (on Earth) of the propellant. (Benson, 2008; Sutton and Biblarz, 2016). The ISP is given by Eq. 1
where
The FFRE combined the existing technologies of low-density dust trapped electrostatically (dusty plasmas) with high field magnets for fission fragment control. By designing the nuclear core to permit sufficient mean free path for the escape of fission fragments, the study showed the FFRE could convert nuclear power to thrust directly and efficiently at a delivered ISP of 527,000 s. The study showed further that, without increasing the reactor power, adding a neutral gas to the fission fragment beam significantly increased the FFRE thrust. This frictional interaction of gas and beam resulted in an engine that would continuously produce nearly 4.5 kN of thrust at a delivered impulse of 32,000 s, thereby reducing the (then expected) round trip mission to Mars from 3 years to 260 days. These studies concluded that the engine and spacecraft were within current technological capabilities, could be built and launched, though the FFRE engines would be very large. New materials, and recent advances in technology, promise to make FFREs much more compact and reliable.
For manned transit to Mars, speed is of the essence to minimize radiation exposure and maximize human health, as discussed in other articles in this volume. Nuclear fuel, with several million times the energy density of any chemical fuel, holds the promise of fast transit to Mars. Typically, two types of nuclear propulsion are considered for space flight: thermal propulsion and electric propulsion.
In thermal propulsion, a coolant that prevents reactor melt-down doubles as a propellant. This gas is heated by direct interaction with the fuel, which limits the gas heating to a temperature below 3000 K, where typical fission fuel melts. A metric of the efficiency of a rocket engine is the specific impulse or ISP. The ISP is thrust (force) of the engine divided by the weight flow of the propellent. The higher the ISP, the more efficient the engine. In nuclear thermal propulsion ISP of approximately 900 s can be achieved, compared to perhaps half that for the best chemical engines. While certainly an improvement, with nuclear energy’s enormous energy density, conventional nuclear thermal propulsion could benefit from technological innovation.
In electric propulsion, the nuclear reactor is configured to produce electricity, and that electricity is used to drive a conventional ion or plasma source that provides propulsion. These sources tend to have high ISP, but low thrust. Thrust, approximately the velocity of ejected propellent times mass flow, is required to lift a rocket out of a gravity well. For a mission to Mars, a rocket engine would ideally have the capacity to produce high thrust (for leaving the Earth, Moon, or Mars) and then high ISP (high efficiency) for interplanetary transit. As an analogy, this is similar to an automobile starting with a low gear and high torque, finally traveling at high speed in a high gear. In a reactor producing electricity, Carnot efficiency requires a large difference in output temperature compared to input temperature. In a vacuum, space craft have difficulty rejecting heat, requiring large radiators. Increasing input temperature is again limited by the melting temperature of the nuclear fuel.
For both of these technologies, nuclear electric propulsion or nuclear thermal propulsion, performance enhancement can be achieved through dealing with the fuel heating problem. If we could heat the propellent to a higher temperature than the melting point of the fuel, then we could achieve higher thrust. If we could operate the nuclear reactor at a temperature well above the melting point of the nuclear fuel, the Carnot efficiency could also be improved.
Though not currently applied to propulsion, the NASA Kilopower space-reactor design uses a 6-inch diameter core of enriched uranium surrounded by a beryllium-oxide reflector. A boron-carbide rod is used as a reactor switch. The design should provide between 1 and 10 kW of power. These surface power technologies will also guide advancements in designing propulsion systems. (NASA, 2017; NASA, 2018; NASA, 2022). However, to achieve a sufficient ISP, any FFRE designs would require a much higher power output. FFRE designs would likely be operated as a subcritical assembly.
2 Fission fragment rocket engine
Fission fragment nuclear propulsion was proposed by George Chapline of Lawrence Livermore National Laboratory in the late 1980s. (Chapline, 1988; Chapline et al., 1988; Chapline and Matsuda, 1991). The propulsion method depended on the fission fragments of a nuclear reaction partially escaping the fissile fuel, mitigating the heating of the fuel. When a fissile material undergoes fission, two daughter atoms are produced as fragments of the event. These atoms carry a total energy of 160 MeV and can travel at velocities up to 5% of the speed of light. If the fission occurs in a very thin layer of nuclear fuel (a few microns thick), some of the fission fragments can escape without collision, minimizing fuel heating. With a magnetic field used to direct these fragments to the exhaust direction, extremely high ISP could be achieved, perhaps on the order of 106 s. This would be two orders of magnitude larger than an electric ion source and more than three orders greater than chemical or nuclear thermal propulsion. On long transit missions this increased ISP, and the decrease in mass of the rocket as its nuclear fragments are ejected, would result in an extremely high final rocket velocity. Chapline envisioned the thin fissile fuel coating being placed on carbon fibers, the fibers moving at high speed through a neutron moderator region with many other coated fibers, having sufficient fissile mass to sustain the reaction, obtaining reactor criticality. The fibers would then exit the neutron moderator to radiatively cool in the vacuum of space. Even though many fission fragments would escape the fuel and eject at high speed, the carbon fibers would still heat significantly. However, the carbon fibers would still contribute to enhancing the thrust.
To simulate a 14-MeV neutron source incident on a layer of uranium oxide, a nuclear transport code (MCNP6.2) was employed. (Werner et al., 2017). The simulation tracked any heavy ions generated to estimate the percentage that escaped as a function of the thickness of the uranium oxide layer. The total amount of fission fragments created increases with increasing UO2 thickness. This amount only begins to increase by orders of magnitude for layers thicker than 1 mm. The fission fragment population remaining in the UO2 increases with layer thickness. Since a larger fraction of the fission fragments remains in the UO2 for thicker layers, a smaller percentage of the total created fragments escapes the layer. The results of the simulation, illustrated in Figure 1, confirm that nearly 100% of heavy ions can escape for layer thicknesses below a few microns. Although thicker uranium oxide layers generate more fission fragments, almost all heavy ions remain trapped inside the fissile material. Therefore, using fissile layers thicker than approximately 10 microns does not yield any benefits to the ISP.
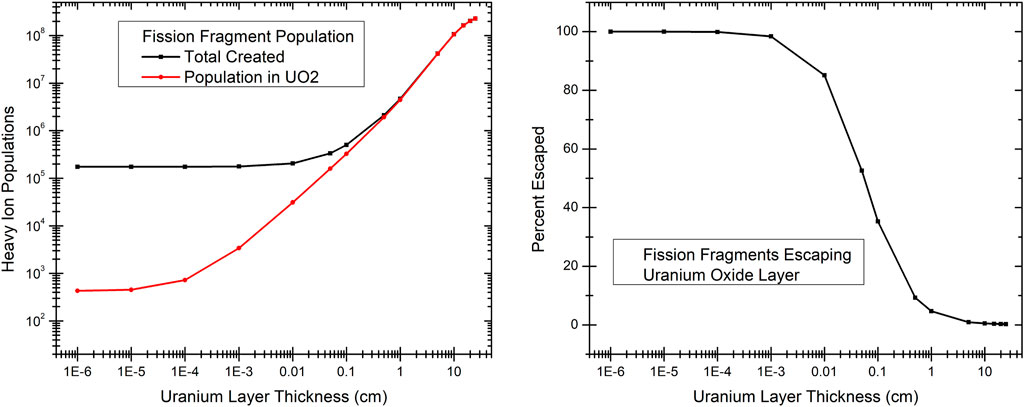
FIGURE 1. Simulation results for fission fragments in various zones around a uranium oxide layer. Left: The population of fission fragments either escaping or remaining inside the uranium oxide layer as a function of layer thickness. Both the total amount of fission fragments created, as well as the population remaining in the layer, increase with layer thickness. Right: The percentage of fission fragments escaping as a function of uranium oxide thickness. Though more fission fragments are created for thicker layers, a smaller percentage is able to escape the fissionable fuel layer.
To avoid this heating, NASA initiated the study of the Fission Fragment Rocket Engine (FFRE) concept in 2010. (Clark and Sheldon, 2005; Werka et al., 2012; Clark et al., 2013). The FFRE would directly convert the momentum of fission fragments into spacecraft momentum at extremely high ISP. The FFRE combined the existing technologies of low-density dust trapped electrostatically (dusty plasmas) with high field magnets for fission fragment control. The fissile fuel fragments and dust would be micron-sized, allowing for the fission fragments to escape the fuel particle without depositing significant energy into the fuel particle or heating it. By designing the nuclear core’s uranium dust density to permit a sufficient mean free path for the escape of fission fragments, while simultaneously maintaining density sufficient to maintain criticality, the study showed the FFRE could convert nuclear power to thrust directly and efficiently at a delivered ISP of 527,000 s.
Without increasing the reactor power, adding a neutral gas to the fission fragment beam significantly increased the FFRE thrust. This frictional interaction of gas and beam resulted in an engine that would continuously produce nearly 4.5 kN of thrust at a delivered impulse of 32,000 s, thereby reducing the (then expected) round trip mission to Mars from 3 years to 260 days. It importantly would allow the heating of the gas propellent to a very high temperature, improving thermodynamic efficiency without melting the uranium oxide fuel. The fuel dust would still heat from the gamma radiation in the reactor, however the small size of the fuel dust would mean that the particles had a larger surface-area-to-volume ratio compared to large particles, enhancing radiative cooling of the fuel.
These studies concluded that the engine and spacecraft were within current technological capabilities, could be built and launched, however the FFREs would be very large. To sustain a nuclear reaction, a neutron produced by fission in the fuel should be moderated and available to initiate another fission event in order to “go critical.” There also needs to be a sufficient amount of fuel mass to continue the reaction. In the FFRE concept, the fuel density needs to be low enough for fission fragments to get out. The design constraint is to design a reactor that keeps neutrons in, and allows fission fragments out, and fundamentally this means a low-density fuel in a large volume.
In an early conceptual design, superconducting magnets were used adjacent to the nuclear reaction. With one magnet having a radius of over 5 m, the coils would have a combined mass of over 40 metric tons. Either a moderator or neutron reflector would be needed to keep neutrons in the reactor, maintaining criticality. This would add over 50 metric tons and the total engine mass would be over 113 metric tons. (Clark et al., 2013). In this design, the extra mass would not be expended as the rocket accelerated, substantially limiting the final velocity of the rocket.
Though there are a number of other very practical issues to consider for the successful deployment of a dusty plasma FFRE, perhaps most importantly, no one has ever built or tested a dusty plasma reactor on Earth, let alone in space.
3 Innovations on the fission fragment rocket engine
3.1 Aerogel core
Ryan Weed and others (Weed et al., 2022) have suggested a modification of the dusty plasma FFRE concept. By utilizing low density aerogel materials to contain micron-sized fuel particles, the significant complications of the dusty plasma confinement can be eliminated. The distribution of fissile particles in the aerogel can be engineered to facilitate criticality while allowing fission products to escape. The low density of the aerogel would minimize energy loss from the fission fragments while at the same time facilitating radiative cooling of the fissile particles. Spent, aged, or depleted aerogel could be simply replaced with the low mass of replacement aerogels, amounting to a trivial amount of total spacecraft mass. Recent advances in magnetic technology (MIT News, 2018) along with reactor design versatility made possible by the utilization of aerogel could dramatically reduce FFRE mass and make deployment on “conventional” space craft, such as SpaceX’s Falcon 9, possible.
3.2 Subcritical assembly
The mean free path of thermal neutrons inside low enriched uranium is about 4.4 mm, 1.4 mm in highly enriched uranium, and 2.5 mm in high assay low-enriched uranium. (Reilly, 1991; Rinard, 1997). The mean free path for thermal neutrons in uranium dioxide is approximately 1.65 cm when using natural uranium. Please see the Supplemental Materials for additional information on these calculations. Additionally, the US Department of Energy has explored the tradeoffs and impacts of using various concentrations of enriched uranium on the masses of reactors for space applications. (US Department of Energy, 1994). Reactors have sufficient fissile fuel and sufficient moderation and neutron reflection to sustain a nuclear chain reaction. If any of these requirements for criticality are removed, an accelerator can be used to produce neutrons to facilitate continued nuclear reactions. A reactor that cannot sustain a nuclear chain reaction is not critical and is not, by definition, a reactor. It is a subcritical nuclear assembly. A subcritical assembly is not at criticality. Since it is driven by an accelerator that can vary its energy, the subcritical assembly permits direct control to throttle the thrust of the engine based on the neutron flux provided from the external neutron source and converter.
Of many examples, Gahl and Flagg (2014) proposed the use of an aqueous solution of uranium salts in heavy water as a subcritical assembly. Driven by a high energy electron beam, producing x-rays upon hitting a high-Z converter, the accelerator would induce photoneutron production in the deuterium of the heavy water. The photoneutrons would induce fission in the uranium, (Lakosi et al., 2006), producing fission fragments for use in radio pharmacy. With more uranium and better reflection, this assembly would have been a reactor. But if kept subcritical, the device would be much easier and safer to develop, operate, and license. In a subcritical FFRE assembly, an electron accelerator could produce x-rays and subsequently photoneutrons through the irradiation of metallic deuterides. (Steinetz et al., 2020; Gillespie et al., 2023a). The light-element metallic deuterides would also be used as neutron moderators in the subcritical FFRE assembly, since they very effectively reduce the fission neutrons’ energy through elastic collisions. A simplified example of the FFRE assembly is shown in Figure 2.
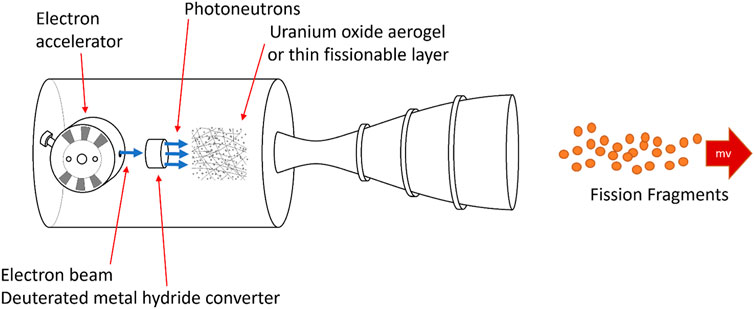
FIGURE 2. A simplified depiction of an electron beam incident on deuterated metal hydride converter. The generated bremsstrahlung x-rays create photoneutrons that drive fission in thin layers of a fissionable material.
There are many types of neutron sources available to drive fission reactions, from simple, commercially available DD fusion systems, spontaneous neutron emitting isotopes, to bremsstrahlung x-ray sources driving photoneutron reactions. Applied to FFRE technology, accelerator driven systems would be simpler to develop, much easier to control, and could facilitate the development of very small FFREs, whose geometry would never allow for a system to achieve criticality. Some of the fission fragments, such as the ones propagating on the thrust axis along the direction of travel, may be used to produce electricity, preferably by direct conversion, to power the accelerator and to meet other spacecraft needs. In addition, the small amount of waste heat generated by the subcritical assembly may be cooled through a thermoelectric converter to produce spacecraft power. Table 1 lists neutron sources that can be used in the fission reaction. Cf-252, with a half-life of 2.65 years and 2.31 × 106 n/s per µg, is the only californium isotope that emits neutrons spontaneously. D-D and D-T reactions generate monoenergetic neutrons of 2.45 and 14 MeV respectively when D+ and/or T+ ions have low kinetic energy. In recent decades, quite a few compact neutron generators using radio-frequency (RF) driven plasma discharges have been developed. (Reijonen et al., 2007; Verbeke et al., 1999; Wu, 2009). These compact neutron generators are composed of three main parts: the plasma source, the acceleration column, and the target. The source is a deuterium plasma formed by ionizing deuterium gas through RF coupling. The deuterium ions present in the plasma are then extracted from an aperture in the plasma facing electrode and accelerated up to over 100 keV onto a metal target. The target is typically either a pre-loaded metal hydride target, or a plain metal target that can adsorb deuterium and tritium atoms very efficiently and form metal hydrides. The incoming ions can then collide with the implanted deuterium through D-D or D-T fusion reactions and produce neutrons of 2.45 and 14.1 MeV respectively. These neutrons can then be moderated and collimated to the necessary energies and direction by using various shielding materials such as polyethylene, heavy water, or light water. (Sharma et al., 2014; Gillespie et al., 2023b).
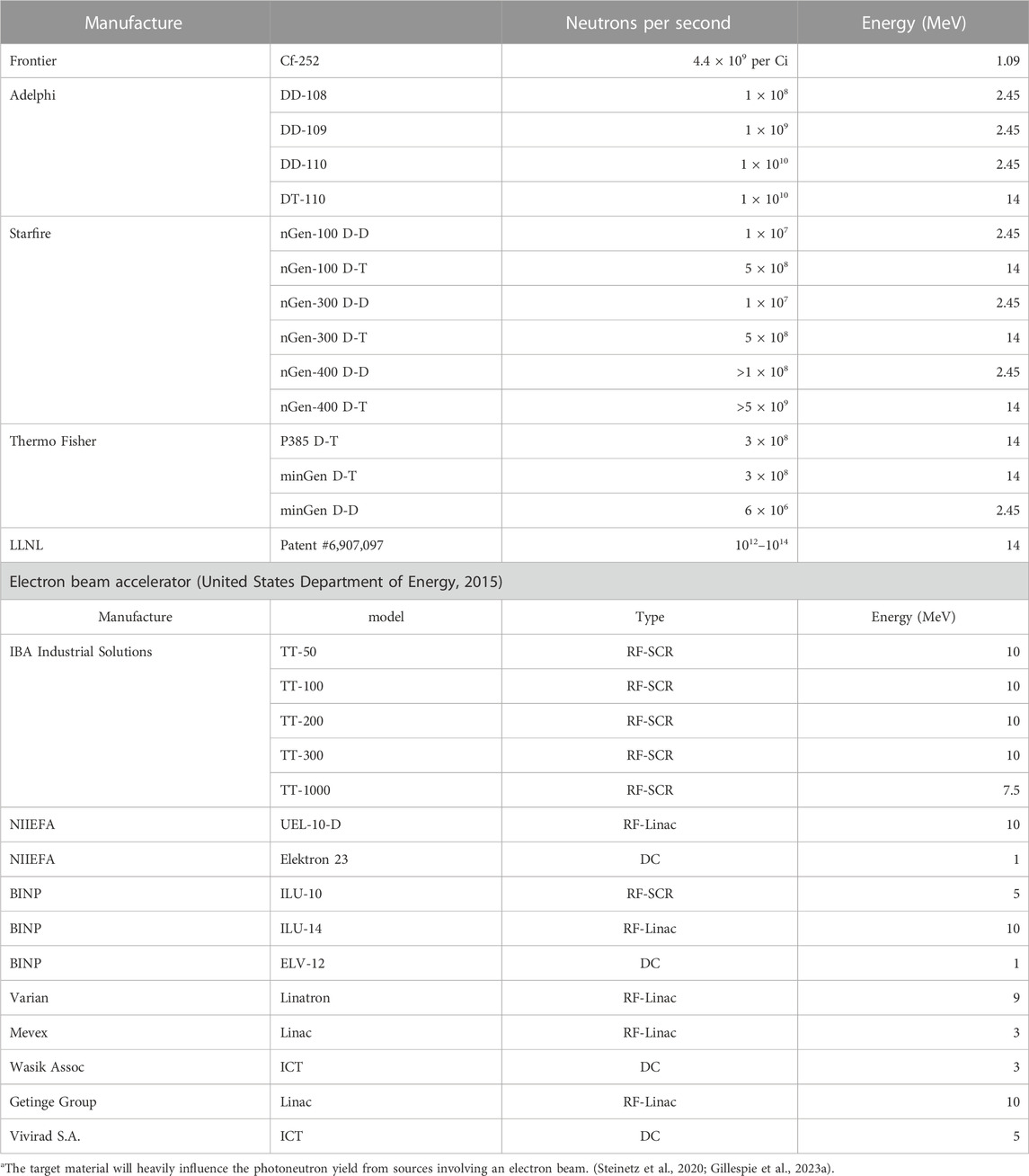
TABLE 1. List of commercially available neutron and electron sources for use in driving fission reactions.
From the beginning of US nuclear reactors in space, metal hydrides were utilized to moderate high energy neutrons. They provide excellent energy transfer because the protons are the same mass as the neutrons. Thermalizing the high energy neutrons will be advantageous for driving the reaction. The exterior target is in a heavily deuterated moderator for fast neutrons. Project Rover Kiwi, the Orion Program, and NERVA utilized heavily deuterated materials to sustain and moderate neutrons to enhance fission yield. (Pedersen, 1964; Dewar, 1974; Dyson, 2003; NASA, 2021). The heavily deuterated metals that are acting as a moderator and will also create secondary neutrons to help drive the reaction.
The photoneutron can serve as another potential neutron source. Chadwick and Goldhabber discovered the nuclear photoeffect on the deuteron. (Chadwick and Goldhaber, 1934). Table 2 outlines the materials and their corresponding low threshold reactions, with D and 9Be being the only nuclides possessing low binding energies, namely, 2.23 and 1.66 MeV, respectively. Though other low-threshold materials such as 6Li, 7Li, 13C, 233U, 235U, and 238U could be used as a target for photo-fission, making use of the photoeffect on deuterium is attractive due to its low threshold and relatively large cross section. Electron accelerators can also act as neutron generators with certain advantages, including higher intensity, lower cost, and smaller size than nuclear reactors, albeit with lower flux in thermal spectra. Prior research has successfully generated neutrons using high-energy electron beams. (Kim, 2000; Chen, 2006; Moreh et al., 2006; Petwal et al., 2007). Tabbakh reported achieving 1012 n/cm2/s, with average energies of 0.9, 0.4, and 0.9 MeV for the Pb, Ta and W targets, respectively using RHODOTRON TT200 (IBA). (Tabbakh et al., 2012). Thus such equipment displays great potential as a neutron source. Further, the TT200 is a 10-MeV source. The neutron yield could also be improved for more efficiency if higher energy accelerator is used. We have determined from our MCNP6.2 simulations in a typical geometry that ∼104 electrons are required to generate adequate gamma radiation to produce one neutron through the photodisintegration of a deuteron. In this configuration, each photoneutron will need to create about 104 235U fissions, so this system must be operated very close to criticality. In addition, a neutron amplifier can also be considered, comprising a cascaded series of stages consisting of fissionable fuel cells separated from each other by neutron moderator walls. (Borst, 1996; Magill, 2004). By integrating these innovations into existing designs, it may become feasible to construct FFREs with much lower masses while improving upon the ISP for reduced transit times.
4 Conclusion
Fast transit to Mars poses several challenges to rocket design due to overall mass, specific impulse, thrust, and thermal management. It may be possible to circumvent several of these obstacles by using subcritical assemblies, energy efficient neutron generation, and compact, high-field magnets to generate and steer fission fragments. Integrating these innovations into FFRE designs may provide increased specific impulse and controlled thrust while operating as a subcritical assembly. These new technologies and improvements may be integrated into a revolutionary nuclear rocket technology and dramatically reduce transit time to Mars.
Data availability statement
The datasets presented in this study can be found in online repositories. The names of the repository/repositories and accession number(s) can be found below: The data and methods recorded and utilized in this study can be found at https://www.depts.ttu.edu/phas/cees/, and through the Information Technology Division of Texas Tech University.
Author contributions
All authors contributed to the conception and design of the research reported here. AG and CL conducted the MCNP6.2 simulations, and all authors calculated the relevant cross-sections as a function of energy using ENDF. JG wrote the first draft of the manuscript. All authors contributed to the article and approved the submitted version.
Funding
This work was funded by Texas Tech University, by the University of Missouri, and in part by the Texas Research Incentive Program.
Acknowledgments
We are grateful to Matthew Looney (Texas Tech University) his suggestions and efforts as the Radiation Safety Officer involved in this effort. We thank Prof. John Brockman from MURR for his advice and recommendations associated with the radiations reported herein.
Conflict of interest
The authors declare that the research was conducted in the absence of any commercial or financial relationships that could be construed as a potential conflict of interest.
Publisher’s note
All claims expressed in this article are solely those of the authors and do not necessarily represent those of their affiliated organizations, or those of the publisher, the editors and the reviewers. Any product that may be evaluated in this article, or claim that may be made by its manufacturer, is not guaranteed or endorsed by the publisher.
Supplementary material
The Supplementary Material for this article can be found online at: https://www.frontiersin.org/articles/10.3389/frspt.2023.1191300/full#supplementary-material
References
Borowski, S. K. (1987). Nuclear propulsion – a vital technology for the exploration of mars and the planets beyond. Case for Mars III. 1988.
Borst, L. (1996). Neutron amplifier (U.S. Patent No. 3291694A). United States: U.S. Patent and Trademark Office.
Brown, D., Chadwick, M., Capote, R., Kahler, A., Trkov, A., Herman, M., et al. (2018). ENDF/B-VIII.0: the 8th major release of the nuclear reaction data library with CIELO-project cross sections, new standards and thermal scattering data. Nucl. Data Sheets 148, 1–142. doi:10.1016/j.nds.2018.02.001
Chapline, G., and Matsuda, Y. (1991). Energy production using fission fragment rockets. Fusion Technol. 20 (4), 719–722. doi:10.13182/fst91-a11946925
Caldwell, J. T., Dowdy, E. J., Berman, B. L., Alvarez, R. A., and Meyer, P. (1980). Giant resonance for the actinide nuclei: photoneutron and photofission cross sections forU235,U236,U238, andTh232. Phys. Rev. C 21, 1215–1231. doi:10.1103/physrevc.21.1215
Chadwick, J., and Goldhaber, M. (1934). A nuclear photo-effect: disintegration of the diplon by -rays. Nature 134, 237–238. doi:10.1038/134237a0
Chapline, G., Dickson, P., and Schnitzler, B. (1988). Fission fragment rockets: a potential breakthrough. No. EGG-M-88285; CONF-880911-18. Idaho Falls (USA): EG and G Idaho, Inc.
Chapline, G. (1988). Fission fragment rocket concept. Nucl. Instrum. Methods Phys. Res. Sect. A Accel. Spectrom. Detect. Assoc. Equip. 271 (1), 207–208. doi:10.1016/0168-9002(88)91148-5
Clark, R., and Sheldon, R. (2005). “Dusty plasma based fission fragment nuclear reactor,” in 41st AIAA/ASME/SAE/ASEE Joint Propulsion Conference and Exhibit, 4460.
Clark, R., Sheldon, R., and Werka, R. (2013). “Dusty plasma based fission fragment nuclear rocket,” in Proceedings of Nuclear and Emerging Technologies for Space 2013, 6790.
Dewar, J. (2007). To the end of the solar system: the story of the nuclear rocket. 2nd ed. Burlington: Apogee.
Dewar, J. (1974). Project Rover: a study of the nuclear rocket development program, 1953–1963. Washington, DC: US Department of Energy.
Gahl, J., and Flagg, M. (2014). Radioisotope production and treatment of solution of target material. US Patent # 8644442, Date of Patent February 4.
Gillespie, A. K., Lin, C., Looney, M., and Duncan, R. V. (2023b). Simulating radiation shielding effectiveness for a 10-mCi californium source, 2.45-MeV neutron source, and a thermofisher P385 neutron generator. arXiv preprint: arXiv:2309.15125, Available at: https://doi.org/10.48550/arXiv.2309.15125.
Gillespie, A. K., Lin, C., and Duncan, R. V. (2023a). Photoneutron yield for an electron beam on tantalum and erbium deuteride. arXiv preprint arXiv:2308.02629, Available at: https://doi.org/10.48550/arXiv.2308.02629.
Haslett (1995). Space nuclear thermal propulsion program final report (PDF) (report). Kirtland Air Force Base. New Mexico: Phillips Laboratory.
Kim, G. N. (2000). Pulsed neutron source using 100 MeV electron linac at Pohang accelerator laboratory, in Proc. XX International Linac Conference, U.S.A.
Koning, A., Rochman, D., Sublet, J., Dzysiuk, N., Fleming, M., and Van der Marck, S. (2019). TENDL: complete nuclear data library for innovative nuclear science and technology. Nucl. Data Sheets 155, 1–55. doi:10.1016/j.nds.2019.01.002
Lakosi, L., Nguyen, C. T., and Bagi, J. (2006). Photoneutron interrogation of uranium samples by a 4 MeV LINAC. A feasibility study. Vienna: International Atomic Energy Agency.
Lieberman, R. J., (1992). Audit report on the timber wind special access program (PDF) (report). Arlington, Virginia: United States Department of Defense, 93–033.
Magill, J. (2004). Neutron amplifier assembly” (U.S. Patent No. US20040228433A1). United States: U.S. Patent and Trademark Office.
MIT News (2018). Major advance toward fusion energy. Available at: https://news.mit.edu/2021/MIT-CFS-major-advance-toward-fusion-energy-0908 (Accessed March 15, 2023).
Moreh, R., Block, R., and Danon, Y. (2006). Generating a multi-line neutron beam using an electron Linac and a U-filter. Nucl. Instrum. Methods Phys. Res. A562, 401–406. doi:10.1016/j.nima.2006.02.160
NASA (2018). Demonstration proves nuclear fission system can provide space exploration power. Available at: https://www.nasa.gov/press-release/demonstration-proves-nuclear-fission-system-can-provide-space-exploration-power.
NASA (2022). NASA announces artemis concept awards for nuclear power on moon. Available at: https://www.nasa.gov/press-release/nasa-announces-artemis-concept-awards-for-nuclear-power-on-moon.
NASA (2021). Nuclear rockets. Available at: https://www1.grc.nasa.gov/historic-facilities/rockets-systems-area/7911-2/(Accessed September 15, 2023).
NASA (2017). Space technology mission directorate. Available at: https://www.nasa.gov/directorates/spacetech/kilopower.
Petwal, V., Senecha, V., Subbaiah, K., Soni, H. C., and Kotaiah, S. (2007). Optimization studies of photo-neutron production in high-Z metallic targets using high energy electron beam for ADS and transmutation. Pramana – J. Phys. 68 (2), 235–241. doi:10.1007/s12043-007-0027-3
Reijonen, J., Andresen, N., Gicquel, F., Gough, R., King, M., Kalvas, T., et al. (2007). Development of advanced neutron/gamma generators for imaging and active interrogation applications. Orlando, FL, USA: Optics and Photonics in Global Homeland Security III.
Reilly, D., Ensslin, N., and Smith, H. Jr. (1991). Passive nondestructive assay of nuclear materials, Editor S. Kreiner (United States), 363–367.
Sharma, M., Alajo, A. B., and Liu, X. (2014). MCNP modeling of a neutron generator and its shielding at Missouri University of Science and Technology. Nucl. Instrum. A767, 126–134. doi:10.1016/j.nima.2014.08.011
Steinetz, B. M., Benyo, T. L., Chait, A., Hendricks, R. C., Forsley, L. P., Baramsai, B., et al. (2020). Novel nuclear reactions observed in bremsstrahlung-irradiated deuterated metals. Phys. Rev. C 101, 044610. doi:10.1103/physrevc.101.044610
Sutton, G. P., and Biblarz, O. (2016). Rocket propulsion elements. New York: John Wiley and Sons, 27. ISBN 978-1-118-75388-0.
Tabbakh, F., Aldaavati, M., Hoseyni, M., Rezaee, K., and Saraee, E. (2012). Induced photonuclear interaction by Rhodotron-TT200 10 MeV electron beam. Pramana - J. Phys. 78, 257–264. doi:10.1007/s12043-011-0232-y
United States Department of Energy (2015). Workshop on energy and environmental applications of accelerators. United States Department of Energy.
US Department of Energy (1994). Impact of the use of low or medium enriched uranium on the masses of space nuclear reactor power systems. Washington, DC: USDOE Assistant Secretary for Nuclear Energy.
Verbeke, J., Lee, Y., Leung, K. N., Vujic, J., Williams, M. D., Wu, L. K., et al. (1999). Neutron tube design study for boron neutron capture therapy application. United States: Lawrence Berkeley National Laboratory.
Weed, R., Horsley, M., and Chapline, B. (2022). Cleveland, OH: Nuclear Emerging Technologies Symposium, Lightning Talks, American Nuclear Society.Aerogel supported fission fragment rocket engine core
Werka, R., Clark, R., Sheldon, R., and Percy, T. (2012). Concept assessment of a fission fragment rocket engine (FFRE) propelled spacecraft. No. HQ-E-DAA-TN33885.
Werner, C., Armstrong, J., Brown, F., Bull, J., Casswell, L., Cox, L., et al. (2017). MCNP user's manual code version 6.2. Rep. LA-UR-17-29981. Los Alamos, NM, USA: Los Alamos National Laboratory Tech.
Keywords: fast transit, fragment, nuclear, rocket, FAST, transit, engine, Mars
Citation: Gahl J, Gillespie AK, Duncan RV and Lin C (2023) The fission fragment rocket engine for Mars fast transit. Front. Space Technol. 4:1191300. doi: 10.3389/frspt.2023.1191300
Received: 26 June 2023; Accepted: 25 September 2023;
Published: 13 October 2023.
Edited by:
James Polk, NASA Jet Propulsion Laboratory (JPL), United StatesReviewed by:
Lawrence Forsley, GEC, United StatesCopyright © 2023 Gahl, Gillespie, Duncan and Lin. This is an open-access article distributed under the terms of the Creative Commons Attribution License (CC BY). The use, distribution or reproduction in other forums is permitted, provided the original author(s) and the copyright owner(s) are credited and that the original publication in this journal is cited, in accordance with accepted academic practice. No use, distribution or reproduction is permitted which does not comply with these terms.
*Correspondence: J. Gahl, Z2FobGpAbWlzc291cmkuZWR1