- 1Université de Bordeaux, CNRS, Bordeaux INP, ISM, UMR 5255, Talence, France
- 2European Commission—Joint Research Centre, Sustainable Resources Directorate, Ispra, Italy
- 3ITAP, Université de Montpellier, INRAE, Institut Agro, Montpellier, France
By analogy to conventional environmental impacts, the potential release of debris or generation of fragments can be considered as the emission of an environmental stressor damaging the orbital ‘natural’ resource which supports space activities. Hence, it appears relevant to integrate systematically the impact of the emission of debris on the orbital resource within the life cycle impact assessment (LCIA) step to broaden the scope of life cycle assessment (LCA) for space systems. The main objective of this article is to propose a set of characterization factors to compute the impact caused by the generation of debris within the orbital environment. To do so, the proposed approach follows the methodology of emission-related characterization models in LCIA. the characterization model enables to link the emission of debris and final economic damages to space activities through a complete impact pathway including the fate of debris in downstream orbital compartments, the exposure of targeted space objects to this debris, and the economic damage in case of collision between the debris and the space object. The model is computed for different compartments of the low earth orbit (LEO) region thanks to a discretization of the orbital environment. Results show that the potential damages are the highest for orbital compartments located in the orbital bands of altitude/inclination: 550–2000 km/52–54°, 1,200–2000 km/86–88°, 400–2000 km/96–100°, because of the downstream location of Starlink constellation, OneWeb constellation, and earth observation satellites, respectively. The proposed set of CFs can be used in the LCA of different space systems in order to include impacts and damages related to space debris, along with other environmental impacts. This original development fully in line with the standardized LCIA framework would have potential for further integration into harmonised sector-specific rules for the European space sector such as product environmental footprint category rules (PEFCR).
1 Introduction
Context. The United Nations’ treaties consider outer space as a ‘Global Common’ of humankind such as oceans, the atmosphere, or the Antarctic. The topic of space sustainability has gained increasing recognition among States and intergovernmental organisations (UN COPUOS, 2017). The use of outer space provides a unique application and essential data collection service dealing with Earth observation and climate monitoring as well as telecommunication, navigation, and human space exploration (Yang et al., 2013). However, 60 years of space exploitation led to a rising sustainability concern in the outer space environment: more than 35,000 human-made objects larger than 10 cm (ca. 10, 000 tonnes), are orbiting the Earth but only 15% are operational spacecraft (Krag, 2021; ESA, 2022). The remaining objects are uncontrolled space debris which is today a significant and constant danger for all space missions. Hence, the global environmental impact of the space sector in the outer space is expected to increase because of the limitation of the current set of Space Debris Mitigation (SDM) guidelines and standards. The emergence of innovative systems such as mega-constellation of satellites is driving the growth of the sector and its potential space debris-related impact (Dolado Perez, 2016; Liou et al., 2018; Somma et al., 2018; Krag, 2021).
Regarding more conventional environmental impacts, several actors of the European space industry have already worked on the life cycle environmental impact assessment (LCIA) of space activities during the last decade, particularly the European Space Agency (ESA) with its Clean Space Initiative launched in 2012. They have identified environmental life cycle assessment (LCA) according to ISO14040/44 as the most appropriate methodology to measure and then minimise their environmental impact (ESA, 2016). LCA is also the basis of the EU product environmental footprint (PEF) method, recommended by the European Commission (EC) since 2013 and now acknowledged as a reference method for the EC initiative on substantiating green claims (EC, 2021). In Europe, the development of space sector-specific guidelines compliant with the LCA conceptual framework and the PEFCRs methodology is currently debated (Wilson et al., 2021; Pinto, 2022).
By analogy to conventional environmental impacts, the potential release of debris or generation of fragments can be considered as the emission of an environmental stressor damaging the orbital ‘natural’ resource which supports space activities (Maury, 2019; Maury et al., 2020). Hence, it appears relevant to integrate systematically the use of the orbital resource and emission of debris-related impacts within the life cycle impact assessment step to broaden the scope of LCA for space systems and ensure consistency regarding the potential development of PEFCRs for the European space sector. The idea of integrating such metrics in LCA of space missions has been discussed for several years now.
Literature review. Within the aerospace engineering community, Letizia et al. (2017) review several indices that assess the criticality of a space mission against the current orbital debris population. These indices can be used 1) to evaluate the impact on the debris environment by adopting a risk assessment-based approach (Letizia and Lemmens, 2021); (ii) to target the best candidates for a future active debris removal campaign (Pardini and Anselmo, 2018; Borelli et al., 2021) as well as during the design phase of a space mission (iii) to identify the optimal strategy in term of end-of-life scenarios (Colombo et al., 2017). Numerical and analytical approaches have already been proposed and discussed by several authors, based on the density of debris (Rossi et al., 2015) or the flux of debris (Kebschull et al., 2014; Anselmo and Pardini, 2015; Letizia et al., 2016, 2019). More recently, the topic of space capacity, also linked to space traffic management (STM), has started to emerge (Krag et al., 2017; Letizia et al., 2020) and is now gaining interest (Colombo et al., 2021; Lewis and Marsh, 2021; Trozzi et al., 2021). The most advanced initiative regarding the application of space sustainability metrics to space missions comes from a joint work led by the ESA and the World Economic Forum, called Space Sustainable Rating (SSR) (World Economic Forum, 2021). However, the scope of such metrics is going well beyond the scope of conventional environmental footprint analysis by gathering socio-economic and competitive aspects with risk assessment metrics and potential environmental aspects within a composite indicator (Letizia et al., 2021).
Up to now, none of these indicators could be integrated as such in the development of a PEFCRs approach for space systems or programs, even if the coupling with the life cycle assessment methodology is not new. Colombo et al. (2017) developed a preliminary index while a comprehensive framework was published by Maury et al. (2017). These initial studies led to a joint work aiming at the creation of dedicated characterisation factors to include the space debris-related impacts within the LCAs of space missions. Once developed, this characterisation model was successfully applied to compare two mission design scenarios based on ESA Sentinel-3 mission parameters activities (Maury, 2019; Maury et al., 2020). In this way, the full life cycle of a space mission was covered with a holistic approach, also covering the “multicriteria” dimension of the standardised LCA methodology.
Research need. However, none of these contributions adopt full compliance with the life cycle impact assessment since they do not follow a clear LCIA impact pathway linking inventory (i.e., debris generation) and environmental impact through a consistent characterisation model with associated characterisation factors (Verones et al., 2017; Rosenbaum et al., 2018).
Goal. Consequently, the main objective of this article is to propose a set of characterisation factors (CFs) to assess the impact caused by the generation of debris within the orbital environment. To do so, the characterization model enables to link the emission of debris (after a collision or voluntary release) and final economic damages to space activities as a function of the orbital location of the generated debris and the exposed space object (such as a satellite).
2 Material and method
The proposed approach follows the methodology of emission-related characterization models in LCIA (Rosenbaum et al., 2018). In such a case, the characterisation factors of a given substance addressing environmental damages at the endpoint level are expressed as the product of the fate factor (FF), the exposure factor (XF), and the effect factor (FF). We propose to adapt each factor to the orbital environment (see Figure 1).
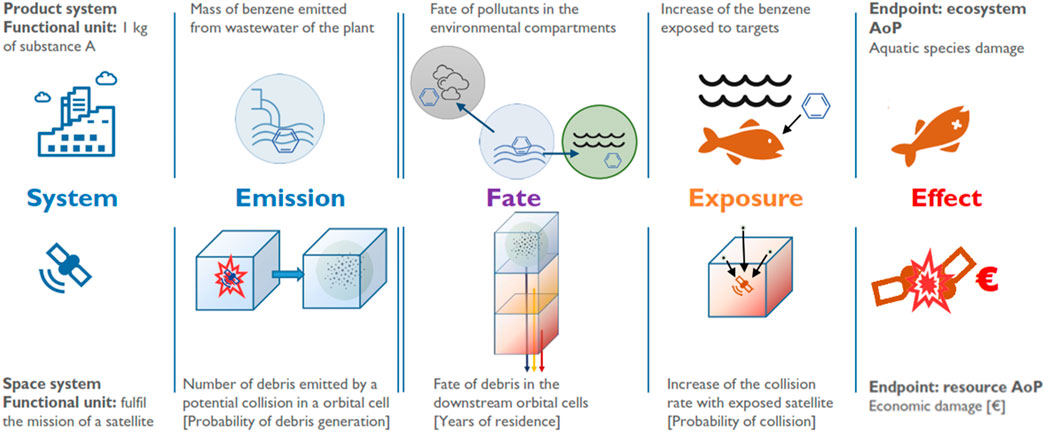
FIGURE 1. Analogy between emission related environmental impact and space debris related impact through the LCIA framework.
By analogy with the work carried out by the ‘task force mineral resource’ in the frame of the Life Cycle Initiative hosted by UN Environnement (Berger et al., 2020), we define the following: within the area of protection ‘natural resources’, the safeguard subject for ‘orbital resources’ is the potential to make use of the value that orbital resources can hold for humans in the technosphere. The damage is quantified as the reduction or loss of this potential caused by human activities in outer space.
Hence, we develop a characterisation model based on a set of characterisation factors (CFj) assessing the potential impact (I) caused by the debris generation ND,j from a product system (spacecraft, launch vehicles, mission-related objects) within a given orbital compartment j (Eq. 1).
Modelling the potential emission of space debris (ND) is already possible in the LCA of space systems (Maury, 2019). The methodology for computing ND,j is provided in the Supplementary Information for different particles flux covering the LEO region.
A marginal impact approach can be adopted, which represents the additional environmental impact per additional emission on top of the existing background impact (which is not caused by the modeled product system) as described by Verones et al. (2017) for other environmental impacts. The total impact of the system under study on the orbital environment Itot is the sum of the impacts in each orbital compartment.
CFs represent the economic damage within this orbital cell associated with the increase of collision per debris emitted within an orbital cell. Following the impact assessment models based on emissions-related impacts (Van Zelm, 2010; Hauschild and Huijbregts, 2015; Rosenbaum et al., 2018), a CF of a given substance addressing environmental impacts can be expressed as the product of the following factors (see also the analogy presented in Figure 1):
i. Fate factor (FF), which corresponds to environmental processes causing transport, distribution, and transformation of the emitted substance.
ii. Exposure factor (XF), which consists of the contact of the substance with sensitive targets in the receiving environment
iii. Effect factor (EF), which represents the observed adverse effect on the sensitive target after exposure to the substance.
Hence, the characterisation factor for emissions-related characterization models can be expressed as proposed in Eq. 2.
Each following subsection describes one of these factors. We characterize an orbital cell j as a function of its altitude h and inclination i compared to the equatorial plane (see Supplementary Information, section 1- Inventory). The LEO region is a spherical shape around Earth extending until 2000 km. The model proposed in this article is valid for altitudes ranging from 250 km to 2000 km with a 50 km interval. In the following work, inclination varies between 0° and 180° with a 2° inclination interval.
2.1 Fate model
The fate model aims at linking the quantity of debris released in the orbital environment to the residence time of the debris in a given orbital cell. It is quantified by the fate matrix which represents the residence time of debris in each orbital compartment, as a function of the orbital cell of emission.
The residence time of debris can be computed with the model developed by Krag et al. (2018, 2017), which assesses the survivability S of fragments >10 cm released at an altitude
Where coefficients
Within Eq. (3), the time parameter
i. A debris is systematically decaying in a lower altitude compartment (i.e. it cannot reach an upper orbital cell)
ii. Every debris that has left the orbital cell j has resided in the orbital cell j-1 (i.e. it cannot join the orbital cell j-n directly, with n > 1)
Therefore, the residence time of the debris in a given orbital cell j (FFj, in years) can be expressed as in Eq. 4 and presented in Figure 2.
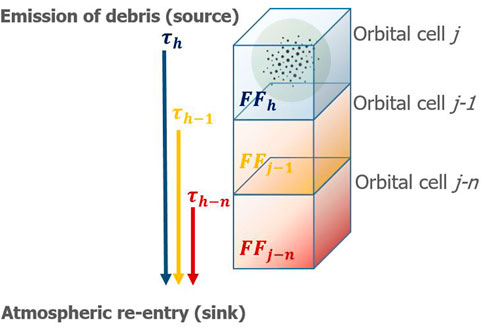
FIGURE 2. Graphical representation of the source and sink mechanisms. For a given emission compartment j, a residence time in the total orbital environment (τj) is computed while FFj represents the residence time in the given orbital cell.
The structure of the fate matrix FF is proposed in (Eq. 5). Columns represent the emission compartments while rows represent the receiving compartments. The sum of a column represents the total time spent by a debris in the orbital environment to reach the sink (i.e. upper layer of the atmosphere).
2.2 Exposure model
The exposure factor in a given orbital cell
We model the exposure factor for a given orbital cell (XFj) as the collision rate between one single debris and these space objects in the volume of a given orbital cell j per unit of time, following the analogy with the theory of gas dynamics presented by Klinkrad et al., 2006. The collision rate (c) per debris (N) in a compartment j is calculated (Eq. 6) as the product of the cumulative exposed area of both active and inactive objects within the orbital cell with the average relative velocity of collision of a debris with its target in the orbital volume.
Where
2.2.1 Average velocity of collision
We consider a non-oriented flux colliding a fictive sphere with a normal angle for a selected circular orbit. In such case, the velocity of collision is only dependent on the velocities of the fictive target and the flux particles because of the normal angle (see Supplementary Information SI1, section 3 for more details). The MASTER-2009 model allows us to obtain and discriminate the complete spectrum of the relative velocity of collision for given orbital coordinates (Klinkrad et al., 2006; Technische Universität Braunschweig, 2011; Technische Universität Braunschweig, 2014). As previously described in Letizia et al. (2018), the peak in the spectrum corresponds to the most probable velocity of collision (∼14.5 km/s). Instead of taking this parameter, the weighted average velocity is obtained by summing the relative contribution of each impact velocity class. To conform to the spatial discretization of our model (range of altitude [250; 2000 km] with a 50 km-interval and the range of inclination [0; 180°] with a 2° inclination interval), these average velocity values are computed to cover these discretized ranges (Supplementary Table S1).
2.2.2 Cumulative area
The cumulative area, expressed in m2, is obtained by summing the cross-section areas of all orbiting objects (active and inactive) within an orbital compartment j.
The cumulative area distribution of inactive objects in the LEO region has been given by the ESA’s space debris office1 based on the internal version of the ESA’s database called DISCOS (Database and Information System Characterising Objects in Space). It serves as a single-source reference for launch information, object registration details, launch vehicle descriptions, spacecraft information (e.g. size, mass, shape, mission objectives, owner), as well as orbital data histories for all trackable, unclassified objects which sum up to more than 40,000 objects. We assume that the number of inactive satellites has not significantly evolved since 2019. Conversely, the population of active satellites has changed significantly since 2020, following the launch of the Starlink constellation. In order to obtain up-to-date active population of satellites, we use the database assembled by the Union of Concerned Scientists (UCS- a non-profit science advocacy organisation based in the United States), freely available as a downloadable Excel file (UCS, 2022). The version used in the present article is the one of 1 January 2022. The type of satellite and their orbital parameters are available for almost all active satellites inventoried in the United Nations Registry of Space Objects to which all the spacefaring nations are required to report space launches. Unlike the DISCOS database, UCS only provides the mass of the active satellites (not their exposed area). The following relationship can be used to link the mass to the average cross-section area (Eq. 7, Dickey and Culpt, 1989). The conversion was found empirically against data from on-orbit payloads, empty rocket bodies, and the measurement of re-entered debris.
Where M is the mass of the object, in kg; and Ac is its cross-sectional area, in m2. In this equation, 62 corresponds to the object density and 1.13 is a geometrical factor that represents a tradeoff between a hollow cylinder (1.0) and a solid cylinder (1.5).
Based on this relation, the cross-section area of each active satellite inventoried in the UCS database is estimated and the cumulative area of the active satellite population is then computed for each orbital compartment j and added to the exposed area of inactive satellite population (Supplementary Table S2).
2.2.3 Volume of the orbital compartment
To compute the volume of the orbital compartment, we first calculate the ring volume, which is equal to the difference between two spheres of successive orbit radii i.e. with an altitude range of 50 km in our spatial discretisation. Then, this volume is divided by 180 to ensure a 2° range per cell, in line with the discretisation previously defined (Supplementary Table S3).
2.3 Effect model
While the effect factors in LCIA usually targets human health or ecosystem quality at the endpoint level (see Figure 1), the novelty of our approach is to translate such adverse effect to final damages in the area of protection ‘resources’. Such a link between emission-related impacts and final resource damages at the endpoint level has been previously explored by Pradinaud et al. (2019) for water use. Considering the resource framework in LCA, ‘future efforts’ methods based on cost externalities are currently seen as a promising approach at the endpoint level (Berger et al., 2020; Sonderegger et al., 2020). Similarly, we propose to assess the potential damage that a collision with a debris can have from an economic perspective. We assume that the value of the orbital resource can be approximated by the future economic revenues generated by the active satellites’ population in each orbital compartment. It should be noted that this approach has been already investigated by Borelli et al. (2021). In the present study, we choose to consider the revenue associated with downstream segments (i.e., service providers), which also partly includes end-users potential applications, even if boundaries between the space downstream and the non-space activities that it enables are difficult to set (PwC, 2022). Indeed, the main economic added value of the space sector is not in the data or imagery transmitted through spacecraft, but rather in the potential downstream applications and new markets enabled by the tremendous quantity of data generated.
We adopt a mass criterion to estimate the yearly potential revenue generated by the downstream and potential end-users applications in $ per kg of an active satellite. Also, the revenue is different depending on the type of mission: earth observation and science, communications, navigation, and other application. The mass distribution according to the main missions carried out by the 4,078 active satellites in the LEO region according to the UCS database is the following: Earth observation and Earth science, 50%; communications and connectivity 49%; others 1%. It should be noted that navigation satellites are exclusively located in medium-earth orbit (MEO) which is out of our scope.
Regarding the revenues of the downstream segment according to the type of missions of each active satellite, we compile several sources of data coming from confidential market reports and other market reports focusing on Earth observation, Navigation, and Communication applications (PwC, 2019, 2022; EUSPA, 2022; OECD, 2022). The year 2030 is chosen as the reference year to estimate the potential future annual revenue per kg of active satellites depending on their mission. Indeed, satellites that were launched recently (such as Starlink constellations) will reach their maturity in several years.
Hence, the effect factor (
Where M is the total sum of each active satellite mass m launched to fulfill a main mission k within the orbital compartment j. The coefficient
2.4 Final characterization factors
Based on Eq. 2 and the methodological development above, the final characterization factors are obtained by multiplying the fate factor with the exposure and the effect (Eq. 9).
These characterization factors represent the potential damage caused by the release of one additional debris (on top of the background population) in a given orbital compartment of the LEO orbital environment. The final socio-economic damage is approximated by the potential loss of revenue at the downstream level to reflect the quality decrease of the orbital resource when additional debris is emitted.
3 Results
3.1 Fate
Fate factors represent the residence time of a debris in each orbital cell and are shown in Supplementary Table S4 and Figure 3. A debris emitted above 1,600 km will spend more than 100 years in the orbital environment, while if emitted below 500 km it will reach the upper layer of the atmosphere in less than 10 years due to the drag effect.
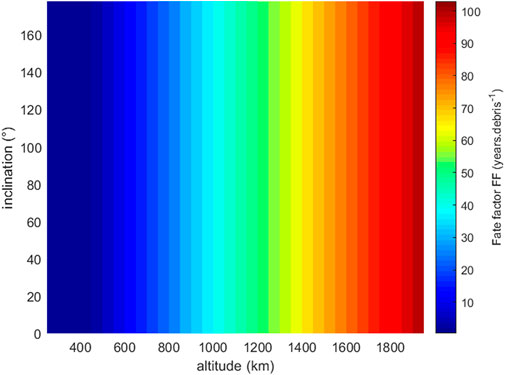
FIGURE 3. Fate factors for an altitude ranging from 250 to 2000 km and an inclination from 0 to 180°.
3.2 Exposure
Exposure factors represent the collision rate between a single debris and the exposed space objects in each orbital compartment. Figure 4 shows that exposure factors range from 10–7 and 10–2 collision·year−1. It means that a debris has a probability to collide with a space object from 0.00001% to 1% depending on the orbital compartment each year. The orbital cells with the highest exposure factors are at altitudes below 700 km and inclination <100° (Supplementary Table S2) because half of the total orbital population, active and inactive, are located in this range. Few inclinations are well suited for space observation which is typically the case for sun-synchronous orbits near the pole (around 96–98°) and other around 50° are adequate for communication purpose. This can be observed thanks to the distribution of cumulative area of satellites. The average velocity of collision retrieved from Master-2009 model, slightly increases for inclination 100° while the volume is bigger for orbital cell with a higher altitude, but it does not counterbalance the important presence of satellites for smaller inclinations and altitudes (Figure 4).
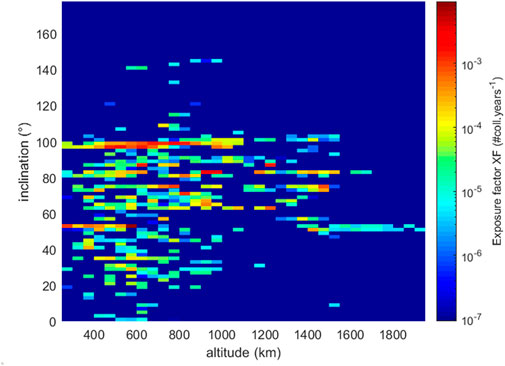
FIGURE 4. Exposure factor for an altitude ranging from 250 to 2000 km and an inclination from 0 to 180°.
Figure 5 shows the product between fate factors and exposure factors, which represents the total collision rate caused by the generation of a debris in each orbital compartment. The highest values are at high altitude because the debris generated in such compartment will cross all the downstream cells of the same inclination (as was shown with the fate factors). The potential emission of debris is very low at high altitudes because of the low flux of debris in the background. Therefore, the impact calculated with Eq. (1) are more likely to be low at high altitudes because of the low value of ND (potential emission of debris) that does not counterbalance the high residence time of debris at high altitudes.
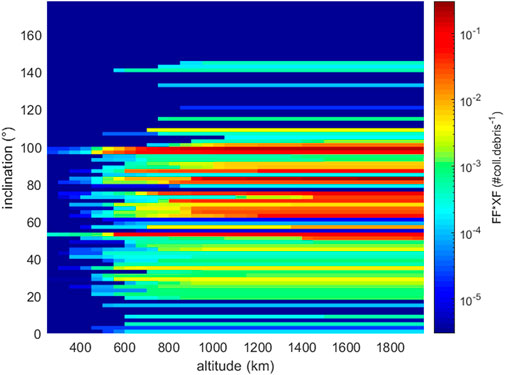
FIGURE 5. Product between fate factor and exposure factor (FF*XF, i.e., number of collisions per debris) for an altitude ranging from 250 to 2000 km and an inclination from 0 to 180°.
3.3 Effect
Figure 6 shows the effect factors, representing the total yearly revenue of each orbital compartment. Starlink constellation (communication), located at an altitude 550–600 km with a 52–54° inclination, generates more than one-third of the total yearly revenue of the orbital environment (estimated as 16.5 billion dollars/year considering our assumptions). As a result, the effect factor is the highest in this orbital environment (Figure 6). To a lesser extent, the OneWeb constellation (communication) generates high revenue at 1200km and 86°–88° inclination (estimated as 2.9 billion dollars/year). Earth observation satellites located around 98–100 (near-polar orbits) also generate a substantial revenue (i.e., nine billion dollars/year considering our assumptions). Overall, the total LEO annual revenues accounted for in the scope of our study are estimated to ca. 45.1 billions.
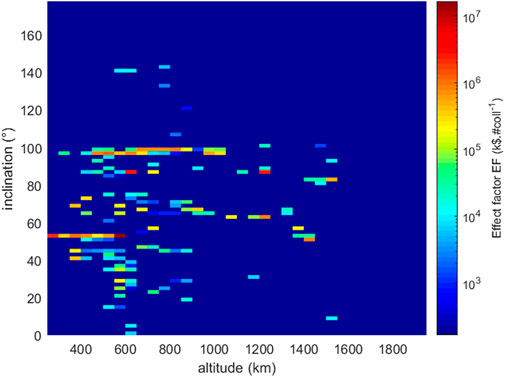
FIGURE 6. Effect factor (i.e., economic revenue generated in each orbital compartment) for an altitude ranging from 250 to 2000 km and an inclination from 0 to 180°.
3.4 Characterization factors
Final characterization factors represent the potential economic damage related to the emission of one debris in each orbital compartment (Figure 7). This is the first attempt to link the emission of a debris (modelled by the inventory) and the endpoint damage considering a full cause-effect chain with fate, exposure, and effect. However, it is to be noted that the effect factor is only a proxy for computing the economic damage caused by a collision with a debris. The effect considers that a debris collision leads to yearly revenue losses related to all the satellites in each orbital compartment. This is an overestimation of revenue losses because one debris collision would not jeopardize the entire orbital compartment. Therefore, the values of CFs should be used with caution and for relative comparison between orbital compartments rather than an absolute valuation of economic losses due to the collision with debris.
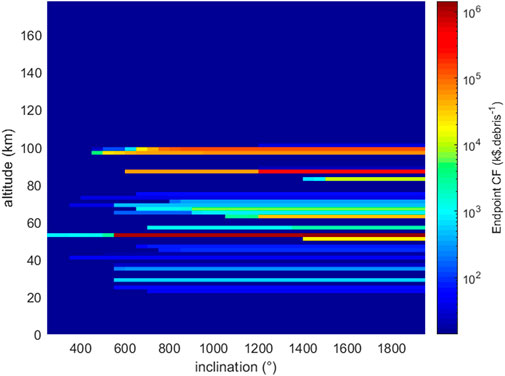
FIGURE 7. Characterization factor for an altitude ranging from 250 to 2000 km and an inclination from 0 to 180°.
That being said, the highest CFs are located in:
- Inclination 52°–54° and altitude above 550 km because debris generated in the [550 km–2000 km] and [52°–54°] orbital band will reach the compartment Starlink constellation is located, according to our model.
- Inclination 86°–88° and altitude above 1,200 km because debris generated in the [1,200 km–2000 km] and [86°–88°] orbital band will reach the compartment OneWeb constellation is located.
- Inclination 96°–100° and altitude above 400 km because debris generated in the [400km–2000 km] and [96°–100°] orbital band will reach the near-polar orbits where earth observation satellites are located.
4 Discussion
4.1 Completeness of the scope and environmental relevance
The paper presents a new methodology and a set of CFs for assessing the impact of a space mission on the orbital resources within the LCA framework. The CFs represent the potential economic damages caused by the generation of debris (which is calculated in the life cycle inventory). The potential damage is computed through a cause-effect chain including the fate of debris in downstream cells, the exposure of targeted space objects, and the economic revenue loss in case of space object collision with the debris. This is in line with emission-related LCIA methodologies as depicted in Figure 1.
The characterization model is suitable for the LEO (300–2000 km) region which is the most exposed to space debris generation (ESA Space Debris Office, 2022). The methodology could also be adapted for MEO and GEO regions. The space debris pressure into MEO region seems nonetheless less important (Johnson, 2010). As for the temporal representativeness, the characterization model is based on values valid for the year 2021 concerning the satellite population (UCS database), and on forecasted economic revenues for the year 2030. The model can be updated yearly to take into account the new satellite launches which are still increasing with satellite constellations generation (ESA Space Debris Office, 2022).
Most of the satellites launched today can perform collision avoidance maneuvers for debris with a diameter >10 cm, which has been disregarded in the exposure model. However, the initial risk calculation to compute the inventory considers all the debris > 1 cm (see Supplementary File S0. Flux of debris-inventory”) meaning that a substantial part of the flow cannot be systematically tracked and avoided by space systems. Regarding secondary collisions assessed within the characterization model, the consequences in term of break-up (e.g. catastrophic collision event) are not assessed. This could be done within the EF model by estimating a representative mass of the exposed satellites (for both active and inactive population) within each orbital slot. Eqs 6–8 provided in the text document of supporting information characterise the nature of the collision while Eq. 5 gives the quantity of fragments generated. Such estimations would also require the average velocity of collision provided in Supplementary File S1 but also the estimated mass of the debris e.g. thanks to the statistic distribution proposed by the MASTER model (Klinkrad et al., 2006; Technische Universität Braunschweig, 2011; Technische Universität Braunschweig, 2014).
The proposed methodology is novel in the frame of LCA as it concerns impacts and damages (considering fate, exposure, effect) on resources (i.e., orbits) and not on ecosystem quality or human health. However, further studies should investigate the economic losses generated by collision with space debris with more accuracy. It is to be noted that the proposed effect factor is a first proxy: we consider that a collision with a debris damages the full orbital compartment where the collision occurs. This leads to an overestimation of the economic losses since not all satellites of the orbital compartment will break up after a collision. On the other hand, the potential economic losses are underestimated since the total revenue per cell is computed for 1 year, while the average lifetime of a satellite in LEO ranges from 5 to 10 years. It should be noted that the direct economic cost of the satellites (including R&D, manufacturing and launch expenses) are not covered by this proxy which focuses only on the “service” delivered by space systems. This upstream direct revenue, including spacecraft manufacturing and launch services, represents around 10% of the total amount against ca. 85% for downstream application (Euroconsult, 2022). Potential insurance costs for company, estimated around $ 500 k-1million for a small LEO satellites (Hussain and Cohn, 2021) are not included neither. In our study, the downstream revenues generated for 1 year ($45.1 b) seems in the same order of magnitude than Euroconsult estimations (Euroconsult, 2022) when navigation satellites in MEO (51% of the total revenue), as well as GEO communication satellites are excluded. This is because both categories are not part of our scope which focuses on LEO activities.
Quantification of endpoint damage for the ‘resources’ area of protection in LCIA is still under debate in the LCA community. Economic valuation (such as done here) seems promising since it may enable to directly compare and possibly integrate the damage on different natural resources. However, some natural resources (e.g. minerals) are evaluated and traded in the markets, while others (e.g. orbits, freshwater, soil) differ from free markets trade mechanisms (UNEP/SETAC Life Cycle Initiative, 2021). Therefore, the economic valuation of natural resources can include different scopes in the valuation (such as use value and non-use value) depending on the adopted approaches. The economic valuation as proposed in this paper could be adapted as a function of the methodological advances in the field.
The current methodology only takes into account the impact related to space debris. Space systems launched in the orbital regions also generate space congestion which can lead to competition with other users to access the same orbital resource. This impact pathway has already been explored by Maury et al. (2019) but would deserve deeper investigations in the future.
The potential environmental impact of one space mission on the orbital environment could be determined by the proposed characterization model. Going further, it appears possible to rank or compare each space mission by adopting a unit of comparison related to the amount of data they generate. Hence, the orbital resource intensity required to generate a certain amount of data by type of mission could be derived A similar approach has been already investigated in the LCA community with the absolute environmental sustainability assessment (AESA) methodology proposed by Bjørn et al. (2019) and based on the physical carrying capacity of ecosystems. An outer space carrying capacity seems an appropriate way to offer a common basis of comparison, e.g. via a normalization factor. (Bjørn and Hauschild, 2015; Bjørn et al., 2020). This approach fits well with the concept of space capacity which is already widely discussed in the aerospace community (Letizia et al., 2020, 2021; Trozzi et al., 2021). The space capacity for each orbital compartment could complement our proposed characterization model via a normalization factor. To ensure consistency with the LCA framework, only parameters related to the space environment should be considered (such as the maximum additional debris that could be emitted before reaching the Kessler syndrome in a given orbital compartment (Miraux, 2022). However, a distance-to-target approach could also be an option for a “composite indicator” not reflecting only environmental mechanisms. In such a case, the normalisation factor for each characterised orbital compartment can be based on international political consensus as proposed by Krag et al. (2017), Krag et al. (2018) and Letizia et al. (2021) or theoretical socio-economic threshold (Rouillon, 2020) allowing normalisation of each space mission contribution and based on international political consensus could be a relevant option.
4.2 Scientific robustness
Even if the computation of the CFs could appear rather simple compared to the ones published by the aerospace experts community (Letizia et al., 2020; Colombo et al., 2021; Letizia and Lemmens, 2021), the impact assessment model allows the calculus of easy-to-handle factors. This is typical of the parsimonious perspective adopted for other LCIA models already available, e.g. in the toxicology field (Rosenbaum et al., 2008).
The fate factor is based on the temporal survivability of the debris in the orbital environment and is only dependent on the altitude in our model. More complete approaches based on a density model of the cloud of debris based on a probabilistic approach could be considered in further development to characterise the most likely behaviour of a fragment at any point in space and time (Letizia et al., 2015; Letizia et al., 2016; Letizia et al., 2017; Frey et al., 2017; Frey et al., 2019).
The exposure factor is mainly dependent of the orbital population composed of both active and inactive spacecraft. The total exposed area provided in the paper represents a picture of the “recent past” based on both launch and space debris databases. Therefore, the exposed area should be regularly updated to obtain representative XFs. A forecast of future launches and orbital population -considering future fragmentation (i.e. collision and break up), could be implemented. This would allow the computation of prospective XFs taking into account the evolution of the orbital environment. This approach has already been proposed in LCA e.g. to characterise water resource scarcity (Baustert et al., 2022).
The methodology to assess the EF factors via the revenue of each type of satellite mission (earth observation, communication, etc.) should be further refined according to a more robust economic valuation method, as already discussed in the previous sub-section.
Finally, potential discrepancies could occur when retrieving the orbital parameters of both active and inactive orbital populations. For instance, this can be the case when recent satellites are inventoried in the UCS database but do not have reached yet their operating orbits. Some potential mismatch between both sources of datasets (UCS and DISCOS databases) could be solved by favouring the exclusive use of the DISCOS database where the active population is regularly updated by the ESA.
4.3 Applicability
This methodology is mainly targeted to LCA practitioners studying the environmental impact of space systems. The developed CFs can be applied for the LCA related to any space system (such as a satellite or launcher) that is located in the LEO region. A prerequisite is to know the potential debris emission (life cycle inventory) which can be computed with the methodology in the SI, and also available in Maury et al. (2019). The number of potential debris ND can be multiplied by the CF of the environment where the debris is generated (see Eq. (1)) in order to compute potential economic damage.
The results can be then integrated into the full LCA of a space system, along with other environmental impacts (such as climate change, toxicity, mineral resource use, etc.). This is useful for comparing the impacts of different scenarios for a space mission, e.g., using different propellants for satellite re-entry. In this case, propellant with higher theoretical efficiency implies the possibility of re-entry of the spacecraft at the end of life and therefore its associated exposure to space debris (Maury et al., 2020). Such scenarios can be compared to typical environmental impacts related to the production of propellants, but also on the emission, fate, exposure, and effect of debris potentially generated during the use and the end-of-life of the satellite thanks to the present methodology. Other relevant studies can include the selection of orbital location for a space system depending on the environmental and orbital impacts.
The characterisation model developed in the paper could represent a good starting point to complete the environmental footprint of space activities based on the EC-recommended product environmental footprint (PEF) methodology (EC, 2021). In the coming years, the European space sector could define and agree on sector-specific common guidelines for environmental footprint such as Product Environmental Footprint Categories Rules (PEFCRs) for space activities (Pinto, 2022). In such a case, a robust environmental indicator, compliant with the LCA framework might be needed to cover environmental impacts occurring in outer space. A generic methodological framework is proposed by Zampori and Pant (2019) and has been successfully deployed in recent years for other sectors, i.e. energy storage with lithium-ion batteries.
5 Conclusion
In this paper, we propose a set of CFs aiming at characterising the full environmental impact pathways for space debris emitted in the orbital environment. A clear impact pathway has been developed in line with the LCA framework and emission-related LCIA models by linking inventory (i.e., debris generation) and impact (i.e., break-up of exposed space objects) and/or final damages (i.e., economic losses due to satellites breakup) in the Area-of-protection ‘resources’.
The entire LEO region is characterised by discretized orbital compartments. For each of them, the temporal fate of debris (fate factors), the exposure of targeted space objects to this debris (exposure factors), and the economic damage in case of collision (effect factors) are computed. Therefore, the present work extends the scope of the LCA studies and potential PEF for complete space missions by integrating environmental impacts occurring in outer space.
Further developments could focus on findings synergies between the proposed model and up-to-date orbital mechanics computation to improve the robustness of the modelisation. The environmental impact assessment could also be automatized via softwares as proposed by (Colombo et al., 2021). This digital solution would allow merging both inventory calculation and final damage results and would ease the assessment of the contribution of a given space mission (for which the design and orbital parameters are known by the user) to potential space-debris related impacts in the orbital environment. The application of normalisation factors based on the environmental space capacity concept could also deserve further attention as this could enhance the sustainable management of the orbital resources by comparing the environmental impact of applications requiring space infrastructure.
Data availability statement
The datasets presented in this study can be found in online repositories. The names of the repository/repositories and accession number(s) can be found in the article/Supplementary Material.
Author contributions
TM-M, AM-M, AH, GS, and PL contributed to the conception and the design of the study. TM-M, AM-M, and PL conducted the methodological developments, organized the database, performed the calculations and wrote the first draft of the manuscript. All authors contributed to manuscript revision, read, and approved the submitted version.
Acknowledgments
The author would like to thank the ESOC space debris office for the satellites population datasets retrieved from the ESA Discos database (data from personal communications shared in August 2019). Flux and velocity of debris used in this study are based on simulations previously obtained thanks to a collaboration with researchers of the project COMPASS at Politecnico Di Milano funded by the European Research Council (Grant agreement No 679086). The authors also acknowledge the support of the French National Association for Technical Research (CIFRE Convention 2015/1269).
Conflict of interest
The authors declare that the research was conducted in the absence of any commercial or financial relationships that could be construed as a potential conflict of interest.
Publisher’s note
All claims expressed in this article are solely those of the authors and do not necessarily represent those of their affiliated organizations, or those of the publisher, the editors and the reviewers. Any product that may be evaluated in this article, or claim that may be made by its manufacturer, is not guaranteed or endorsed by the publisher.
Supplementary material
The Supplementary Material for this article can be found online at: https://www.frontiersin.org/articles/10.3389/frspt.2022.998064/full#supplementary-material
Footnotes
1Personal communication 19/08/2019.
References
Anselmo, L., and Pardini, C. (2015). Compliance of the Italian satellites in low Earth orbit with the end-of-life disposal guidelines for Space Debris Mitigation and ranking of their long-term criticality for the environment. Acta Astronaut. 114, 93–100. Elsevier. doi:10.1016/j.actaastro.2015.04.024
Baustert, P., Igos, E., Schaubroeck, T., Chion, L., Mendoza Beltran, A., Stehfest, E., et al. (2022). Integration of future water scarcity and electricity supply into prospective LCA: Application to the assessment of water desalination for the steel industry. J. Ind. Ecol. 26, 1182–1194. doi:10.1111/jiec.13272
Berger, M., Sonderegger, T., Alvarenga, R., Bach, V., Cimprich, A., Dewulf, J., et al. (2020). Mineral resources in life cycle impact assessment: part II – recommendations on application-dependent use of existing methods and on future method development needs. Int. J. Life Cycle Assess. 25, 798–813. doi:10.1007/s11367-020-01737-5
Bjørn, A., and Hauschild, M. Z. (2015). Introducing carrying capacity-based normalisation in LCA: framework and development of references at midpoint level. Int. J. Life Cycle Assess. 20, 1005–1018. doi:10.1007/s11367-015-0899-2
Bjørn, A., Richardson, K., and Hauschild, M. Z. (2019). A framework for development and communication of absolute environmental sustainability assessment. Methods J. Ind. Ecol. 23, 838–854. doi:10.1111/jiec.12820
Bjørn, A., Chandrakumar, C., Boulay, A.-M., Doka, G., Fang, K., Gondran, N., et al. (2020). Review of life-cycle based methods for absolute environmental sustainability assessment and their applications. Environ. Res. Lett. 15, 083001. doi:10.1088/1748-9326/ab89d7
Borelli, G., Trisolini, M., Massari, M., and Colombo, C. (2021). “A comprehensive ranking framework for active debris removal missions candidates,” in 8th European Conference on Space Debris. Darmstadt, Germany (virtual conference). April 20–23, 2021. Editors T. Flohrer, S. Lemmens, and F. Schmitz (ESA Space Debris Office). Available at: http://hdl.handle.net/11311/1173681.
Colombo, C., Trisolini, M., Letizia, F., Lewis, H. G., Chanoine, A., Duvernois, P., et al. (2017). “Spacecraft design indicator for space debris,” in Clean space industrial days (Noordwijk: ESTEC, ESA - Clean Space).
Colombo, C., Trisolini, M., Gonzalo Gómez, J. L., Giudici, L., Frey, S., Sànchez-Ortiz, N., et al. (2021). “Design of a software to assess the impact of a space mission on the space environment,” in 8th European Conference on Space Debris. Darmstadt, Germany (virtual conference). April 20–23, 2021. Editors T. Flohrer, S. Lemmens, and F. Schmitz (ESA Space Debris Office). Available at: http://hdl.handle.net/11311/1173725.
Dickey, M. R., and Culpt, R. D. (1989). Determining characteristic mass for low-earth-orbiting debris objects. J. Spacecr. Rockets 26, 460–464. doi:10.2514/3.26092
Dolado Perez, J. C. (2016). “Effect of EOL strategies for large LEO constellations on the space environment,” in 6th EU workshop on Satellites End-of-Life. Paris, France (Paris: Centre National d’Etudes Spatiales HQ).
EC (2021). Commission recommendation on the use of the environmental footprint methods. Brussels, Belgium: European Commission. C(2021), 1–23.
ESA Space Debris Office (2022). ESA’s annual space environment report. GEN-DB-LOG. Available at: https://www.sdo.esoc.esa.int/environment_report/Space_Environment_Report_latest.pdf.
ESA (2022). Space debris by the numbers. ESA website. Available at: https://www.esa.int/Safety_Security/Space_Debris/Space_debris_by_the_numbers (Accessed May 9, 2022).
Euroconsult (2022). Euroconsult estimates that the global space economy totaled $370 billion in 2021. Press release. Available at: https://www.euroconsult-ec.com/press-release/euroconsult-estimates-that-the-global-space-economy-totaled-370-billion-in-2021/.
EUSPA (2022). EUSPA EO and GNSS market report. Luxembourg: Publications Office of the European Union. doi:10.2878/94903
Frey, S., Colombo, C., Lemmens, S., and Krag, H. (2017). “Evolution of fragmentation cloud in highly eccentric orbit using representative objects,” in 68th International Astronautical Congress (IAC 2017), Adelaide, Australia, September 25–29, 2017, 1–11.
Frey, S., Colombo, C., and Lemmens, S. (2019). “Interpolation and integration of phase space density for estimation of fragmentation cloud distribution,” in 29th AAS/AIAA Space Flight Mechanics Meeting, Ka’anapali, HI, USA, January 13–17, 2019.
Hauschild, M. Z., and Huijbregts, M. A. J. (2015). “Introducing the life cycle impact assessment,” in Life cycle impact assessment (Springer Netherlands), 1–16. doi:10.1007/978-94-017-9744-3
Hussain, N. Z., and Cohn, C. (2021). Launching into space? Not so fast. Insurers balk at new coverage. Reuters. Available at: https://www.reuters.com/lifestyle/science/launching-into-space-not-so-fast-insurers-balk-new-coverage-2021-09-01/(Accessed October 5, 2022).
Johnson, N. L. (2010). “Medium earth orbits: is there a need for a third protected region,” in Proc. 61st Int. Astronaut. Congr. Int. Astronaut. Fed., Paris, France, 2010, 1–11.
Kebschull, C., Radtke, J., and Krag, H. (2014). “Deriving a priority list based on the environmental criticality,” in Proc. 65th Int. Astronaut. Congr., Toronto, Canada, September 29–October 3, 2014, 1–9.
Klinkrad, H., Wegener, P., Bendisch, J., and Bunte, K. (2006). “Modeling of collision flux for the current space debris environment,” in Space debris: Models and risk analysis (Darmstadt: European Space Agency, Springer), 115–142. doi:10.1007/3-540-37674-7_4
Krag, H., Lemmens, S., and Letizia, F. (2017). “Space traffic management through the control of the space environment’s capacity,” in 1st IAA Conference on Space Situational Awareness (ICSSA), Orlando, FL, USA, November 13–15, 2017.
Krag, H., Letizia, F., and Lemmens, S. (2018). “Space traffic management through the control of the space environment’s capacity,” in 5th European Workshop on Space Debris Modeling and Remediation, Paris, France, June 27, 2018 (Paris: CNES HQ).
Letizia, F., and Lemmens, S. (2021). “Evaluation of the debris environment impact of the esa fleet,” in 8th European Conference on Space Debris. Editors T. Flohrer, S. Lemmens, and F. Schmitz (ESA Space Debris Office). Available at: https://conference.sdo.esoc.esa.int/proceedings/sdc8/paper/94/SDC8-paper94.pdf.
Letizia, F., Colombo, C., and Lewis, H. G. (2015). Analytical model for the propagation of small-debris-object clouds after fragmentations. J. Guid. Control Dyn. 38, 1478–1491. doi:10.2514/1.G000695
Letizia, F., Colombo, C., Lewis, H. G., and Krag, H. (2016). Assessment of breakup severity on operational satellites. Adv. Space Res. 58, 1255–1274. doi:10.1016/j.asr.2016.05.036
Letizia, F., Colombo, C., Lewis, H. G., and Krag, H. (2017). “Extending the ECOB space debris index with fragmentation risk estimation,” in 7th European Conference on Space Debris, Darmstadt, Germany, April 18–21, 2017(Darmstadt: ESOC).
Letizia, F., Colombo, C., Lewis, H. G., and Krag, H. (2018). “Development of a debris index,” in Stardust final conference. Editors M. Vasile, E. Minisci, L. Summerer, and P. McGinty (Springer International Publishing), 191–206. doi:10.1007/978-3-319-69956-1_12
Letizia, F., Lemmens, S., Bastida Virgili, B., and Krag, H. (2019). Application of a debris index for global evaluation of mitigation strategies. Acta Astronaut. 161, 348–362. doi:10.1016/j.actaastro.2019.05.003
Letizia, F., Lemmens, S., and Krag, H. (2020). Environment capacity as an early mission design driver. Acta Astronaut. 173, 320–332. doi:10.1016/j.actaastro.2020.04.041
Letizia, F., Lemmens, S., Wood, D., Rathnasabapathy, M., Lifson, M., Steindl, R., et al. (2021). “Framework for the space sustainability rating,” in Proc. 8th European Conference on Space Debris (virtual conference), Darmstadt, Germany, April 20–23, 2021. Editors T. Flohrer, S. Lemmens, and F. Schmitz (ESA Space Debris Office).
Lewis, H. G., and Marsh, N. (2021). “Deep time analysis of space debris and space,” in 8th European Conference on Space Debris (virtual conference), Darmstadt, Germany, April 20–23, 2021. Editors T. Flohrer, S. Lemmens, and F. Schmitz (ESA Space Debris Office).
Liou, J. C., Matney, M., Vavrin, A., Manis, A., and Gates, D. (2018). NASA ODPO’s large constellation study. Orbital Debris Q. News 22. doi:10.17226/4765
Maury, T., Loubet, P., Ouziel, J., Saint-Amand, M., Dariol, L., and Sonnemann, G. (2017). Towards the integration of orbital space use in Life Cycle Impact Assessment. Sci. Total Environ. 595, 642–650. doi:10.1016/j.scitotenv.2017.04.008
Maury, T., Loubet, P., Trisolini, M., Gallice, A., Sonnemann, G., and Colombo, C. (2019). Assessing the impact of space debris on orbital resource in life cycle assessment: A proposed method and case study. Sci. Total Environ. 667, 780–791. doi:10.1016/j.scitotenv.2019.02.438
Maury, T., Morales Serrano, S., Loubet, P., Sonnemann, G., and Colombo, C. (2020). Space debris through the prism of the environmental performance of space systems: the case of sentinel-3 redesigned mission. J. Space Saf. Eng. 7, 198–205. doi:10.1016/j.jsse.2020.07.002
Maury, T. (2019). Consideration of space debris in the life cycle assessment framework. Talence, France: Université de Bordeaux.
Miraux, L. (2022). Environmental limits to the space sector’s growth. Sci. Total Environ. 806, 150862. doi:10.1016/j.scitotenv.2021.150862
Pardini, C., and Anselmo, L. (2018). Evaluating the environmental criticality of massive objects in LEO for debris mitigation and remediation. Acta Astronaut. 145, 51–75. Elsevier Ltd. doi:10.1016/j.actaastro.2018.01.028
Pinto, V. (2022). “EU environmental policies,” in ESA clean space industry days (Noordwijk: ESTEC). Available at: https://indico.esa.int/event/416/contributions/7317/attachments/4857/7481/20221011 - ESA Clean Space Industry Days.pdf.
Pradinaud, C., Northey, S., Amor, B., Bare, J., Benini, L., Berger, M., et al. (2019). Defining freshwater as a natural resource: a framework linking water use to the area of protection natural resources. Int. J. Life Cycle Assess. 24, 960–974. doi:10.1007/s11367-018-1543-8
PwC (2019). Corpernicus market report. Luxembourg: Publications Office of the European Union. doi:10.2873/011961
PwC (2022). Main trends & challenges in the space sector. 3rd Edition, 41–42. Available at: https://www.pwc.fr/fr/assets/files/pdf/2020/12/en-france-pwc-main-trends-and-challenges-in-the-space-sector.pdf.
Rosenbaum, R. K., Bachmann, T. M., Gold, L. S., Huijbregts, M. A. J., Jolliet, O., Juraske, R., et al. (2008). USEtox - the UNEP-SETAC toxicity model: Recommended characterisation factors for human toxicity and freshwater ecotoxicity in life cycle impact assessment. Int. J. Life Cycle Assess. 13, 532–546. doi:10.1007/s11367-008-0038-4
Rosenbaum, R. K., Hauschild, M. Z., Boulay, A.-M., Fantke, P., Laurent, A., Núñez, M., et al. (2018). “Life cycle impact assessment,” in Life cycle assessment, theory and practice. Editors M. Z. Hauschild, R. K. Rosenbaum, and S. I. Olsen (Springer International Publishing), 633–959. doi:10.1007/978-3-319-56475-3
Rossi, A., Valsecchi, G. B., and Alessi, E. M. (2015). The criticality of spacecraft index. Adv. Space Res. 56, 449–460. doi:10.1016/j.asr.2015.02.027
Rouillon, S. (2020). A physico-economic model of low earth orbit management. Environ. Resour. Econ. (Dordr). 77, 695–723. doi:10.1007/s10640-020-00515-z
Somma, G. L., Lewis, H. G., and Colombo, C. (2018). Sensitivity analysis of launch activities in low earth orbit. Acta Astronaut. 158, 129–139. doi:10.1016/j.actaastro.2018.05.043
Sonderegger, T., Berger, M., Alvarenga, R., Bach, V., Cimprich, A., Dewulf, J., et al. (2020). Mineral resources in life cycle impact assessment—part I: a critical review of existing methods. Int. J. Life Cycle Assess. 25, 784–797. doi:10.1007/s11367-020-01736-6
Technische Universität Braunschweig (2011). Final report - maintenance of the ESA MASTER model. Braunschweig: European Space Agency. Available at: http://scholar.google.com/scholar?hl=en&btnG=Search&q=intitle:Final+Report+Maintenance+of+the+ESA+MASTER+Model#0.
Technische Universität Braunschweig (2014). Software user manual MASTER-2009. Braunschweig: European Space Agency.
Trozzi, V., Colombo, C., and Trisolini, M. (2021). “Analysis of possible definitions of the space environment capacity to pursue long-term sustainability of space activities,” in 72 nd Int. Astronaut. Congr., Dubai, United Arab Emirates, October, 2021, 25–29. Available at: https://www.researchgate.net/publication/355939329.
UCS (2022). Satellite database. Excel format. Available at: https://www.ucsusa.org/resources/satellite-database.
UN COPUOS (2017). The “Space2030” agenda and the global governance of outer space activities. Available at: http://www.unoosa.org/res/oosadoc/data/documents/2018/aac_105/aac_1051166_0_html/AC105_1166AEVE.pdf.
UNEP/SETAC Life Cycle Initiative (2021). Global LCIA guidance phase 3 - creation of a global life cycle impact assessment method: Scoping document. Available at: https://www.lifecycleinitiative.org/wp-content/uploads/2021/02/GLAM3-Scoping-document.pdf.
Van Zelm, R. (2010). Damage modeling in life cycle impact assessment. Nijmegen, Netherlands: Radboud University.
Verones, F., Bare, J., Bulle, C., Frischknecht, R., Hauschild, M. Z., Hellweg, S., et al. (2017). LCIA framework and cross-cutting issues guidance within the UNEP-SETAC Life Cycle Initiative. J. Clean. Prod. 161, 957–967. doi:10.1016/j.jclepro.2017.05.206
Wilson, A. R., Morales Serrano, S., Baker, K. J., Oqab, H. B., Dietrich, G. B., Vasile, M., et al. (2021). “From life cycle assessment of space systems to environmental communication and reporting,” in 72nd International Astronautical Congress (IAC), Dubai. Available at: https://strathprints.strath.ac.uk/78417/1/Wilson_etal_IAC2021_From_life_cycle_assessment_of_space_systems_to_environmental_communication.pdf.
World Economic Forum (2021). Space sustainability rating. Shap. Futur. Mobil. Platf. Available at: https://www.weforum.org/projects/space-sustainability-rating.
Yang, J., Gong, P., Fu, R., Zhang, M., Chen, J., Liang, S., et al. (2013). The role of satellite remote sensing in climate change studies. Nat. Clim. Chang. 3, 875–883. doi:10.1038/nclimate1908
Keywords: characterisation model, emission-related impacts, space debris, orbital environment, space activities, space sustainability, life cycle assessment, LCIA (life cycle impact assessment)
Citation: Maury-Micolier T, Maury-Micolier A, Helias A, Sonnemann G and Loubet P (2022) A new impact assessment model to integrate space debris within the life cycle assessment-based environmental footprint of space systems. Front. Space Technol. 3:998064. doi: 10.3389/frspt.2022.998064
Received: 19 July 2022; Accepted: 14 November 2022;
Published: 29 November 2022.
Edited by:
Andreas Losch, University of Bern, SwitzerlandReviewed by:
Joseph N. Pelton, International Space University, United StatesDenilson Paulo Souza Dos Santos, São Paulo State University, Brazil
Copyright © 2022 Maury-Micolier, Maury-Micolier, Helias, Sonnemann and Loubet. This is an open-access article distributed under the terms of the Creative Commons Attribution License (CC BY). The use, distribution or reproduction in other forums is permitted, provided the original author(s) and the copyright owner(s) are credited and that the original publication in this journal is cited, in accordance with accepted academic practice. No use, distribution or reproduction is permitted which does not comply with these terms.
*Correspondence: Philippe Loubet, philippe.loubet@u-bordeaux.fr