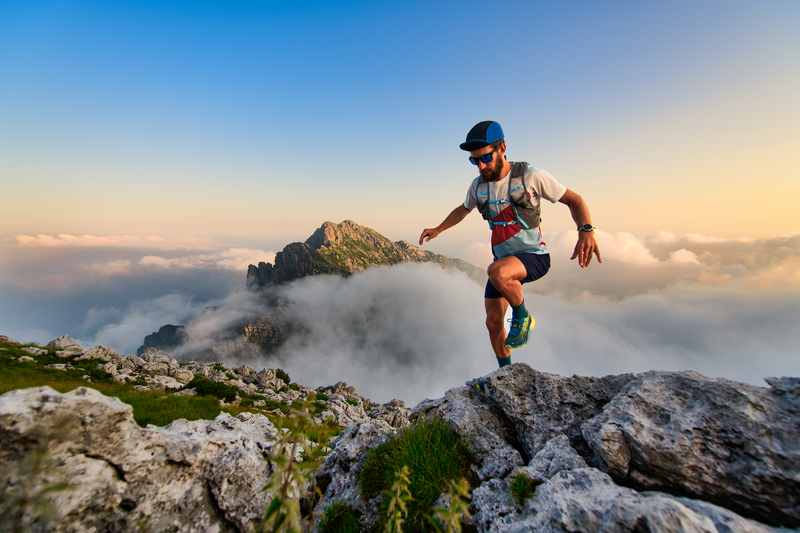
95% of researchers rate our articles as excellent or good
Learn more about the work of our research integrity team to safeguard the quality of each article we publish.
Find out more
TECHNOLOGY AND CODE article
Front. Space Technol. , 13 January 2023
Sec. Space Robotics
Volume 3 - 2022 | https://doi.org/10.3389/frspt.2022.1054360
This article is part of the Research Topic Advancing robotic exploration of Asteroids and Comets: a threat, an opportunity, or both? View all 6 articles
Comets are generally considered among the most pristine objects in our Solar System. There have thus been significant efforts to understand these bodies. During the past decades, we have seen significant progress in our theoretical understanding of planetesimal/cometesimals (the precursors of comets) formation. Recent space missions—such as ESA’s Rosetta mission to comet 67P/Churyumov-Gerasimenko—have provided observations claimed by proponents of different comet formation theories to validate their scenarios. Yet, no single formation paradigm could be definitively proven. Given the importance of understanding how the first bodies in our Solar System formed, we propose a dedicated mission to address this issue. ORIGO will deliver a lander to the surface of a cometary nucleus where it will characterise the first five m of the subsurface. With remote sensing instruments and the deployment of payload into a borehole, we will be able to study the physico-chemical structure of ancient, unmodified material. The mission has been designed to fit into the ESA M-class mission budget.
Comets are considered among the most pristine objects in our Solar System. They have presumably formed beyond Neptune in a massive primordial disk of precursory bodies, commonly referred to as planetesimals or comtesimals (e.g., Nesvorný, 2018, for a review of the dynamics in the early Solar System). When Neptune migrated through that disk these bodies were scattered into the current day Kuiper Belt—in particular the Scattered Disk—where they have remained until recently (e.g., Nesvorný et al., 2016; Nesvorný, 2018). The Scattered Disk is widely considered the reservoir of Jupiter family comets (Duncan et al., 2004; Duncan, 2008; Dones et al., 2015) before they enter the inner Solar System where they exhibit activity driven by the sublimation of ices (in particular H20).
Jupiter family comets (JFC) are the most easily accessible comets for spacecraft missions and as such have been targeted over the past three decades of space exploration. The first comet encounter was not with a Jupiter family comet though, but 1P/Halley, visited by ICE (Farquhar et al., 1985), Vega 1 & 2 (Sagdeev et al., 1985), Sakigake (Hirao & Itoh, 1988), and Giotto (Reinhard, 1982). Subsequently, the following JFCs were visited: 19P/Borrelly (Deep Space 1, Rayman, 2002), 81P/Wild 2 (StardustReichhardt, 1995), 9P/Tempel 1 (Stardust, and Deep Impact, and Deep Impact, A’Hearn et al., 2005), 103P/Hartley 2 (Deep Impact/EPOXI, act/EPOXI, A’Hearn et al., 2011), and most recently 67P/Churyumov-Gerasimeko (Rosetta, Taylor et al., 2017).
While our understanding has greatly improved (see Keller & Kührt, 2020, for a review) many questions remain open. In particular, the hope, that we might infer how comets formed has not been fulfilled and proponents of conflicting formation scenarios claim confirmation in the observations of these missions (Weissman et al., 2020). Although significant progress has been made to understand comet formation on the theoretical side (see Section 3), we seem to have reached an impasse on what ground truths tell us on which of the theories is correct.
We, therefore, propose that a dedicated and highly focused mission is needed to address one of the most important questions in planetary science: “How did comets form?”. Here we present the concept ORIGO for an ESA M-class mission to address this very question which is of paramount importance to understanding how our, and other, planetary systems formed.
To achieve our objective, we argue that access to sub-surface material is needed. This can be achieved by delivering a lander to the surface of a comet. With remote sensing instruments and the deployment of payload into a borehole (a.o. a borehole imager, Kereszturi et al., 2022), we will be able to study the physico-chemical structure of ancient, unmodified material.
We will lay out the scientific goals of this mission in Section 2 and provide the necessary understating of the current state of knowledge of formation theories in (Section 3) and how they can be tested (Section 4). Importantly, in Section 5 we will argue that undisturbed, pristine material is still accessible in the shallow sub-surface of a cometary nucleus.
As it stands, there are two paths to advance our understanding of planetesimal formation: 1) through a sample return mission, and 2) through a dedicated lander probing the subsurface, the latter of which we advocate for here (Section 6). Finally, we will describe the mission configuration (Section 7) and our strawman payload (Section 8).
The goal of ORIGO is to inform and challenge planetesimal formation theories. Understanding how planetesimals form in protoplanetary disks is arguably one of the biggest open questions in planetary science. To this end, it is indispensable to collect ground truths about the physico-chemical structure of the most pristine and undisturbed material available in our Solar System. ORIGO seeks to resolve the question of whether this icy material can still be found and thoroughly analysed in the sub-surface of comets. Specifically, ORIGO aims to address the following immediate science questions.
1. Were cometesimals formed by distinct building blocks such as e.g. “pebbles”, hierarchical sub-units, or fractal distributions?
2. How did refractory and volatile materials come together during planetesimal growth e.g. did icy and refractory grains grow separately and come together later (Figure 1i), or did refractory grains serve as condensation nuclei for volatiles (Figure 1ii)?
3. Did the building blocks of planetesimals all form in the vicinity of each other (Figure 1A), or was there significant mixing of material (Figure 1B) within the protoplanetary disk?
FIGURE 1. Sketch of the stratigraphy of a comet nucleus. Red colour represents dry refractory material, and blue and yellow represent water and CO2 ice respectively. The right-most column shows a column of material with a top shallow layer of ice-free material. Below that we expect a layer containing water ice and refractories, and below that a layer also containing CO2. The centre column illustrates the amount of material that can be removed during the high activity phase around perihelion. Inlay panels (a) and (b) illustrate two possible particle mixtures, one where the material has a similar composition (a) and the other where particles differ significantly (b). Inlay panels (i) and (ii) illustrate two possible mixtures of dust and ice, the first being an inter-mixture of dust and ice particles (i), and the second an encapsulation of dust particles by ice.
To answer these questions ORIGO will deliver a lander to a comet where we will characterise the first five m of the subsurface with a combination of remote-sensing and payloads lowered into a borehole. Our instruments will examine the small-scale physico-chemical structure. This approach will allow us to address the following objectives, each of which informs the respective science question above.
A) Reveal the existence of building blocks of a cometary nucleus from the (sub-)micron to metre scale by exploring unmodified material.
B) Determine the physical structure of these building blocks, in particular, the size distribution of components and how refractory and volatile constituents are mixed and/or coupled.
C) Characterise the composition of the building blocks by identifying and quantifying the major ices and refractory components.
In the following we will discuss how addressing these objectives will inform formation scenarios by reviewing the current state of knowledge, detailing which predictions will be put to the test, and where we will find the needed undisturbed material.
The leading hypotheses for how planetesimals formed from sub-micron dust and ice particles in the proto-planetary nebula can be classified into two groups (see, e.g., a recent review by Weissman et al., 2020).
1) the hierarchical accretion of dust and ice grains to form planetesimals (e.g., Weidenschilling, 1977; Kenyon & Luu, 1998; Windmark et al., 2012a; b; Davidsson et al., 2016); and
2) the growth of so-called “pebbles”, which are then brought to gentle gravitational collapse to form larger bodies by e.g. the streaming instability (e.g., Goldreich & Ward, 1973; Youdin & Goodman, 2005; Johansen et al., 2007; Blum et al., 2017).
It is currently uncontested that collisions between small sub-micron-sized dust and ice grains in the early proto-planetary nebula cause them to stick to each other. This process allows growth from the sub-micron up to the centimetre scale (Weidenschilling, 1977; Dominik & Tielens, 1997; Blum & Wurm, 2000; 2008; Wada et al., 2008; 2009; Güttler et al., 2010; Zsom et al., 2010; Blum et al., 2022). The upper limit of the growth depends on the particle composition and location in the disk. At these larger sizes, further growth is inhibited in most cases because collisions between these larger dust particles result in fragmentation, bouncing, and cratering, thus producing a “growth barrier” (Blum & Wurm, 2000; Güttler et al., 2010; Zsom et al., 2010; Blum, 2018; Schräpler et al., 2018).
The first scenario, hierarchical growth, circumvents this growth barrier in different ways. For example, very small grains can grow into very sticky agglomerates, which in turn can grow to much larger sizes than described above (Ohtsuki, 2012; Kataoka et al., 2013). Alternatively, collisions between particles with large mass ratios allow some mass to be transferred from the small particle to the larger one. Once objects have accreted to the 100-m scale they then primarily grow by equal-sized collisions and gravitational binding (de Niem et al., 2018).
In the second scenario, the growth barrier is overcome in one big leap from centimetre-sized particles, usually referred to as pebbles, to planetesimals. When pebbles are sufficiently concentrated within the disk the pebble cloud can gently collapse under its own gravity. Currently, the preferred and most-studied mechanism that can lead to such a pebble concentration is the streaming instability (Youdin & Goodman, 2005; Johansen et al., 2007; 2014; Wahlberg Jansson & Johansen, 2014; Wahlberg Jansson & Johansen, 2014; Simon et al., 2016; Schäfer et al., 2017; Simon et al., 2017; Wahlberg Jansson et al., 2017; Yang et al., 2017; Abod et al., 2019). This scenario directly forms large (up to 100 s of km) planetesimals (e.g., Simon et al., 2016; Schäfer et al., 2017). It is currently unknown if comets come from the small end of the formation size distribution or are fragments of larger planetesimals.
The theoretical study of planetesimal formation has made impressive advances in the past decade. But previous comet missions, including Rosetta (Taylor et al., 2017) to comet 67P/Churyumov-Gerasimenko, have provided proponents of both scenarios with supporting evidence (Weissman et al., 2020). Importantly though, it is a main motivating principle of this mission to decide which scenario should be preferred, or if another needs to be invoked.
The most straightforward discriminator between the two formation scenarios described above is the predicted internal structure. In particular, the respective building blocks are of very different sizes and compositional heterogeneity. Hierarchical growth occurs over a long time (millions of years) and results in an interior dominated by heterogeneous metre-scale building blocks (Figure 1B). The heterogeneity of the building blocks stems from the long formation time which allows the mixing of building blocks from different regions of the disk. The interior can be characterised as a fractal aggregate interior (Donn & Hughes, 1986) or rubble pile (Weissman, 1986). In particular, we would expect a size distribution of components within the cometary sub-surface that follows a power law (i.e., is scale invariant/fractal).
In contrast, the gravitational collapse of pebble clouds occurs fairly quickly. This scenario leads to relatively homogeneous nuclei (Figure 1A) with two porosity size scales: one on the length scale of the grains (micron) and the other on the length scale of the “pebbles” (cm) (e.g Skorov & Blum, 2012; Blum et al., 2017; Blum, 2018). The size distribution of components in this case would be expected to be bi-modal (or multi-modal) rather than scale invariant.
Therefore, to discriminate between these formation scenarios it is paramount to identify the building blocks of planetesimals (objective A), determine their physical properties (objective B), and their compositional heterogeneity (objective C).
Further, examining how refractories and ices are mixed within these building blocks (objective B) will reveal how dust growth occurred in the protoplanetary disk. For example, did refractory material grow first and then upon crossing of ice lines condensate volatile species around them (Figure 1ii), or did they grow together resulting in a more intimate mixture of the two components (Figure 1i)?
Although accomplishing our objectives could definitively confirm or reject a specific formation scenario, we should also recognise that our findings might not validate any. This would in turn make our measurements a key constraining factor and should inspire novel formation theories at a time when numerical modellers are beginning to be able to address such details more accurately. Crucially, the properties of planetesimal building blocks need to be characterised by the most pristine and undisturbed material.
A link between comets and planetesimals was first postulated by Opik (1961) making comet nuclei the prime target to find the most pristine, undisturbed material. They have been formed and stored in the cold outer Solar System and thus their interiors have not been significantly altered (Duncan et al., 1988; Levison & Duncan, 1997; Dones et al., 2015; Nesvorný, 2018). Thermal processing of the near sub-surface, and the potential origin of comets as collisional fragments of large planetesimals appear to make it unlikely to find undisturbed material close to the surface. As we will argue here, this is not the case.
First, the seasonal thermal skin depth for a comet that has entered the inner Solar System such as comet 67P/Churyumov-Gerasimenko lies at a depth of 1–2 m (Figure 1; Herny et al., 2021; Davidsson et al., 2022). Second, studies have shown that breakups only alter a small fraction of the body close to the surface through heating and compaction (Jutzi & Michel, 2020). Thus, alterations are confined to the near surface. A mechanism that can remove such processed layers would subsequently reveal pristine material. This mechanism is cometary activity. The erosion rate in certain regions of a comet peaking at perihelion can be substantial (e.g., the Southern hemisphere of comet 67P saw between 5 and 10 m of erosion; Figure 1) Herny et al., 2021; Davidsson et al., 2022).
Furthermore, the sublimation fronts during perihelion activity are located very close to the surface (within the first tens of centimetres Herny et al., 2021; Davidsson et al., 2022). This is caused by the diurnal erosion depth being larger than the diurnal skin depth (mm). The seasonal erosion depth is larger than several seasonal thermal skin depths, the location of the H2O sublimation front and likely even the CO2 sublimation front.
Finally, the detection of CO activity at 67P (Morse et al., 2015; Fougere et al., 2016; Gasc et al., 2017) indicates that this highly volatile ice (free sublimation temperature of 26 K) has been retained and is close to the surface indicating minimal thermal processing.
The prime landing site will thus be in an area that has experienced high erosion (e.g., for comet 67P this is the southern hemisphere). This ensures that the most pristine material is accessible within the first 50 cm from the surface.
Because comets have been recognised as time capsules from the early Solar System, multiple missions to these objects have been flown. Europe, led by ESA, has become a leader in this field because of the success of Giotto (Reinhard, 1987) and Rosetta (Taylor et al., 2017) while also selecting Comet Interceptor as a fly-by mission to a dynamically new comet (Snodgrass & Jones, 2019). Though ORIGO would also be a mission to a comet, it should be understood that it will be the first with the primary goal of understanding the formation of these bodies rather than studying their activity.
Retaining leadership in this field can follow one of two paths. The first path is cryogenic or even non-cryogenic sample return. Unfortunately at this point such a mission is well beyond the scope of an ESA M-class mission. Second, significant leaps in our understanding of the formation of the Solar System can be achieved by a focussed mission to explore the sub-surface of a comet in situ as we propose here. The Philae lander on Rosetta (Ulamec et al., 2016; 2017) demonstrated that landing on a comet is possible. While Philae did not achieve all its goals, the Rosetta mission has provided us with sufficient information that a landing on the nucleus is now associated with significantly reduced risk.
Furthermore, our mission is conceived to be complementary to and not competitive with the science of a future sample return mission. Such a mission, e.g., the previously proposed CAESAR mission (Squyres et al., 2018) to NASA’s New Frontiers programme, would provide highly resolved chemical and isotopic information about cometary material. But it would leave open the exploration of the physical properties of cometary material and specifically the volatile-refractory relationships. In this sense sample return and ORIGO together would provide for a rather complete accounting of i) the isotopic and chemical composition (sample return) and ii) the physical structure (ORIGO) of the most pristine material in our Solar System. Furthermore, ORIGO would provide crucial information about the strength of cometary material which is an important property for sample return. Yet, the strength of cometary material is still under debate (Blum et al., 2006; Groussin et al., 2015). ORIGO will directly measure this strength by drilling into the sub-suraface and thus inform which possible sampling mechanisms are most effective for sample return mission.
Designing a comet mission to meet the scientific objectives within an ESA M-class budget is extremely challenging. However, the senior review committee noted “Given the undisputed relevance of a comet sample return mission, advances in the technology of collecting and storing cryogenic samples of cometary ices is highly recommended in view of future missions”1. Our aim here is to provide high-quality scientific results to a broad community in a European-led field while demonstrating new techniques and technologies to facilitate a future cryogenic sample return mission.
Our objectives can be addressed by a highly focused mission, designed to cost, that attacks a critical question in planetary formation theory that will not be addressed in any future NASA New Frontiers sample return mission (i.e., similar to a re-submitted CAESAR). We also note that missions such as CHopper and Chagall have been proposed and determined to be technically and financially feasible within the NASA Discovery programme with both mission concepts being more technically/financially challenging than what we propose here.
The mission approach assumes a mother spacecraft (S-O, the O standing for orbiter) and a lander (S-L, the L standing for lander). Unlike Rosetta, S-O only has three functions. It must identify a landing site, it must bring S-L to the target, and it must act as a data relay from S-L to the ground. S-O itself only provides serendipitous science returns and thus does not carry a science payload. S-L and its capabilities are focused strictly on scientific objectives.
To simplify the mission profile, our target will be one of the short-period comets that have already been the subject of reconnaissance. We choose 9P/Tempel 1, 67P/Churyumov-Gerasimenko, 103P/Hartley 2, and 19P/Borrelly in order of priority. 9P is, at present, the highest priority because it has a long rotation period and provides higher reachability for a lander (incl. suitable landing area, safety, and illumination conditions), allowing more straightforward operations. Typically the delta-V required on the spacecraft is of the order of 1,500–2,000 m/s for such a mission based on previous studies.
We expect that the total dry mass can be constrained to 1,000 kg with S-L contributing 250 kg. The higher mass of S-L with respect to Philae (100 kg) reflects the focus on science and the need to ensure successful landing and anchoring. We assume that the lander can support up to 35 kg of payload (a payload to mass ratio of around 14% which is reasonably conservative cf spacecraft B2 on Comet Interceptor; Snodgrass & Jones, 2019). For operations purposes, we assume an 8-year cruise followed by 4 months of mapping with the navigation camera of S-O only, to allow gravity determination, spin axis verification, and landing site selection. Experience from other small body missions (e.g., Rosetta, Hayabusa 2, Hera) shows that a standard navigation camera provides sufficient resolution (
The science objectives require access to the sub-surface. As drilling (as well as most other mechanical actuation) will lead to uncompensated angular momentum on the lander, some kind of anchoring will be required as implemented on Philae. Despite the failure of Philae’s anchors, the concept of firing individual harpoons, connected to the lander with a re-tensionable tether is still valid and should be re-considered in particular given that we are now aware of the bulk density and porosity of cometary material through Rosetta. The surface strength of pristine cometary material is still under debate (and indeed one aspect to be investigated in preparation for cryogenic sample return) because of contradictory results from Rosetta instruments. But strengths around 20 Pa on large scales and kPa on smaller (lander) scales are supported by most observations (Groussin et al., 2015; Vincent et al., 2015; Roll et al., 2016; Attree et al., 2018) indicating that optimisation/improvement of the anchoring approach can be made.
Other concepts for anchoring spacecraft or landers have been studied and can be traded off. Short (tens of seconds) thruster firings to push the lander downwards is an applicable concept during anchor firing to compensate for a rebound. More (occasionally “exotic”) concepts, including nails, grippers, self-opposing or even fluid systems, on how to anchor a spacecraft to a low gravity body have been published and deserve another critical survey. Following deployment and successful landing, we envisage 4 months of operation on the surface as the prime science phase. This may include perihelion or near-perihelion operations. Once on the surface, the lander will perform a set of activities based around the following three elements listed in Table 1.
To access the most primitive material available, we must study material either in the sub-surface of the nucleus (
Various drilling systems have been proposed for applications on comets (and/or asteroids), and all attempt to cope with the challenges of designing for a poorly defined environment. Generally, a drill offers maximum flexibility to penetrate the material of the widest range of physical properties such as porosity, density or structural strength. Considerable development has been put into the design of SD2 aboard Philae Di Lizia et al. (2016), leading to experience that has been partly re-used e.g., for the design of the ExoMars drill Re et al. (2008). Drilling will likely be an important part of other missions, including sample-return missions to asteroids and dwarf planets (Shi et al., 2021), and the exploration of the Moon (Savoia et al., 2017). For ORIGO at least four scenarios need to be studied.
1. Drill, remove the drill and lower payload(s) into the empty hole
2. Drill with some payload in the drill then retract and lower down the additional payload
3. Drill with a fully integrated payload in the drill structure
4. Digging and/or scooping, subsequent deployment of the payload onto the revealed surface
Drills and scoops have individual advantages and disadvantages. A drill would allow access to deeper depths and could be combined with scientific instruments (microscopic/borehole imager, Raman/LIBS, and a terahertz, .5-6 THz, spectrometer). This could be done by integrating optics, optical fibres and antennas into the drill itself (De Sanctis et al. (2017)). However, a borehole implementation would be difficult with the proposed Scanning Electron Microscope (SEM) and probably impossible with a Laser Ablation Mass Spectrometer (LAMS). Previous studies have shown that a drill would contribute 9 kg and 35 W (peak)/15 W (average).
A scoop would allow better access for the SEM and LAMS instruments but only at a limited depth (past missions, e.g., Phoenix and Viking, have typically dug down to 20 cm. We envisage here, 1-2 thermal skin depths or 5 cm). We consider it necessary to incorporate both options (which have the advantage of providing redundancy). The maintenance of the integrity of the material to be studied by the payload is one of the key difficulties that must be overcome for mission success. It is a requirement that the sampling mechanism does not heat the material significantly. Slow drilling reduces thermal impact. The approach must maintain the microstructure of the material at the μm scale (the size range of the fundamental monomers) and it would be highly desirable on cm scales (the expected size of the primordial pebbles from the disk accretion phase).
Our strawman payload is shown in Table 2. All science instruments will be on S-L. The current total mass of the payload is estimated at
TABLE 2. Strawman payload of ORIGO and the associated measurements, expected mass and power. The second to last indicates the deployment location of the instruments either in the borehole (B), on scooped surface (S), or remain on the lander (L). The last column specifies which science objective is addresses by the respective instrument. The list also indicate the priority of the payload from highest to lowest (objectives A/B, C, to context) and thus provide potential descope options.
To achieve objectives A, B, and C a payload is required that can cover several decades in spatial scales from sub-microns to metres. Figure 2 summarises the payload and their resolution limits (lower bound of the respective boxes) and fields of view (upper bounds of the respective boxes). The payload can also be divided into three tasks: an examination of the physical properties, examination of the chemical properties, and characterisation and monitoring of the environment.
FIGURE 2. The ORIGO strawman payload and associated spacial scales are shown in this graphic: Scanning electron microscope (SEM), Borehole optical microscope (BOM), Terahertz tomo-/spectrograph (THZ), Ground-penetrating radar (GPR), Raman/LIBS (RLIBS), Laser Ablation Mass Spectrometer (LAMS), Panoramic cameras (PanCam), and Environmental package (EP). The instrument details are summarized in Table 2.
The lower bound of each box represents the resolution limit and the upper bound field of view for each instrument. Orange boxes refer to a payload that is examining the physical structure, blue boxes payload dedicated to chemical analysis, and green boxes to payload focused on characterising the environment.
The four primary instruments are the following. First, the highest resolution data will be returned by the Scanning Electron Microscope (SEM). It will be deployed into the borehole and deliver both physical and chemical information about the smallest scale structure. Second, a Borehole Optical Microscope (BOM) will reveal information, amongst others, about how particles are packed, and how the sub-surface matrix is constituted. Third, a Terahertz tomo-/spectrograph (THZ) will provide measurements of the spatial distribution and mixing of refractory and volatile material on the mm-to cm-scales. Fourth, a high-frequency Ground-penetrating radar (GPR) will sit on the lander. It will provide cm-resolution radar imaging of the first 5 m below the landing site which will allow amongst others to identify the stratigraphy and transition between desiccated and different volatile enriched layers.
Two instruments are solely dedicated to retrieving the elemental composition of the sub-surface material. We have chosen Raman spectroscopy and Laser-Induced Breakdown Spectroscopy (LIBS) as well as Laser Ablation/Ionisation Mass Spectrometer (LAMS) to perform this task. Their prime task is to objective C.
Finally, it will be crucial to characterise and monitor the landing site to understand the environment within which all of the measurements are taken. This will be achieved with a panoramic camera suite, which will not only provide a 360° view of the landing site but also feature a downward-facing camera to capture the landing site during the landing and post-landing phase. It will also include an upward-facing camera to catch any potential activity above the lander. The lander will also be equipped with an Environmental Package consisting of small sensors to measure, for example, the temperature and gas pressure at the landing site, the amount of dust deposition onto the lander, and the dielectric properties of the near subsurface. These sensors will be attached to the lander and its landing gear to allow surface contact when needed.
The orbiter (S-O) will be required to carry a high-resolution navigation camera for landing site selection, an S-O to S-L communication link, and lander support hardware (i.e., ejection system). An ultra-stable oscillator (USO) is considered to be highly useful for near-nucleus navigation and could provide additional science.
To achieve our stated mission goals a payload suite (Table 2) is needed that includes instruments that currently have low Technology Readiness Level (TRL). Therefore, a development of instruments associated with respective funding is needed in the coming years to bring these instruments to TRL 5. We will discuss the most important investment needs in the coming years.
The scanning electron microscope is one of the key instruments that requires further development. Currently, there is an SEM named Mochii available from a Seattle-based startup company called Voxa (Martinez et al., 2019). Mochii was flown to the ISS in 2020 (Own et al., 2020) and can therefore be considered TRL 5. For ORIGO an SEM would need additional development. For example, the instrument could be significantly simplified for cometary applications because it would not require a vacuum chamber (the comet’s surface is already at vacuum conditions).
Broadband THz spectroscopy in the .5-6 THz range is a relatively new technique that is advancing rapidly. There are already commercial suppliers of laboratory devices that can be used to study material at a resolution of
The ORIGO Ground-penetrating radar (O-GPR) is a High Frequency Radar (1–5 GHz) with full polar capability to support deep structure characterisation. Optimised to operate at close distance to the surface, the radar benefits from a full-deramp architecture with slow CW modulation and limited transmitted power. The Tx- and the Rx-antennas are implanted on a 1-m boom while the rotation of the platform is providing diversity of observation geometries and synthetic aperture capabilities. The addition of an Rx-antenna accommodated on the platform provides bistatic capacities, thereby improving resolution. The instrument is inheriting from Wisdom/Exomars Rover (TRL 9) (Ciarletti et al., 2017) and Chimera (Herique et al., 2019) as redesigned in the frame of the NEOMAPP H2020 study (TR5). With regard to these instruments, the O-GPR electronics requires a partial redesign for an enlarged bandwidth (1–5 GHz). The selected architecture is validated: A first breadboard has been validated and no difficulties have been identified at design nor component level. The Vivaldi antenna system (Plettemeier et al., 2009) is a scaled version of the Wisdom one.
The RLIBS has significant space heritage. This includes ChemCam (only LIBS) aboard Curiosity Rover (Fabre et al., 2011), SuperCam aboard Perseverence Rover (Manrique et al., 2020), ExoMars RLS (Raman only; Veneranda et al., 2021), and RAX - the small Raman spectrometer developed for MMX Rover (Cho et al., 2021). The same is true for the LAMS, which has heritage from the Phobos-Grunt Mission (Managadze et al., 2010) and Luna-Glob (Chumikov et al., 2021). Thus, the instruments to meet objective C already have high TRL and therefore will not need significant development.
The PanCam also has significant hardware heritage. Similar systems have flown on Rosetta’s Philae (CIVA, and ROLIS; Bibring et al., 2007a; Mottola et al., 2007), on Hayabusa II (MASCOT MasCam Jaumann et al., 2017), and were developed and built for the Rosalind Franklin PanCam (Coates, 2019). The necessary modifications would likely include a new sensor board to allow for a new-generation CMOS detector. Furthermore, some minor development would be needed on the data and commanding interface and to the power supply.
The Environmental Package (EP) is conceived as a multi sensor package for in situ measurements to investigate the physical properties of the surface, soil and subsoil and the environment of the comet. The parameters that could be measured are.
1) Surface temperature, thermal conductivity and inertia to investigate steady and transient thermal properties (e.g., by heating resistor cells, drilling operations).
2) Surface strength, material density, cohesion, porosity, particle size distribution and layering to assess the mechanical properties of comet’s material.
3) Electrical properties such as soil permittivity, conductivity, electric field, discharges and dust electrification.
4) Monitoring dust and volatiles to investigate comet’s environment and possible activity.
Taking advantage from previous in situ missions (e.g., Rosetta/Philae at the comet 67P (Bibring et al., 2007b; Biele & Ulamec, 2009), Hayabusa II/MASCOT (Ho et al., 2017) for asteroid sample return, Cassini/Huygens at Titan (Lebreton & Matson, 2003), ExoMars/Schiaparelli (Esposito et al., 2018; Ball et al., 2022) and InSight (Banerdt et al., 2020) at Mars), various types of sensors could be proposed to perform measurements at different sites of the lander (e.g., on the lander’s feet, within the drill, etc.,) and depths. The flexible structure of the EP allows for the integration of multiple sensors served by a common electronics and data management unit. Although the heritage from instrumentation flown in previous in situ missions grants a high TRL for the sensors to be included into the EP, new technological development could be envisaged for developing new types of measurements, sensor miniaturisation and performance improvement.There is a significant heritage of drilling systems for comets. SD2 on Rosetta demonstrated the technology in the operative environment (Di Lizia et al., 2016), even though it was not possible to perform all the planned operations. The drill had a vertical translational degree of freedom (DoF) to reach the comet surface and thus the selected drilling site, which could be the basis for further technological development to increase the TRL of a multiple-hole technology. Multiple drilling locations would increase the scientific return of the proposed mission, but consistent development steps are required since there are no relevant demonstrations of the technology. The Ma_MISS instrument on the ExoMars Drill demonstrates the possibility of integrating scientific instruments directly in a drill. Further improvements are necessary to allow the possibility to accommodate bigger instruments, ensuring the required connections for power and data without degrading the performance of the drill. The required development steps face both the miniaturisation of the instruments and, as mentioned, the connection between the instruments on the lander and their probes on the drill.
How comets formed remains a crucial open question in planetary science. Answering this question will allow us to understand how our, and other, planetary systems formed.
Although significant advances to understanding comet formation have been made on the theoretical side, we still lack the definitive proof to decide between conflicting theories. We therefore believe that a highly focused and dedicated mission is needed to prove ground truths and perform unambiguous measurements that can disentangle formation theories. This concept was designed to fit into the ESA M-class mission program.
The objectives of the ORIGO mission are to 1) understand if cometesimals are formed by distinct building blocks such as e.g., “pebbles”, hierarchical sub-units, or fractal distributions; 2) determine if refractory and volatile materials came together during planetesimal growth e.g., did icy and refractory grains grow separately and come together later, or did refractory grains serve as condensation nuclei for volatiles; and 3) examine if the building blocks of planetesimals all formed in the vicinity of each other, or if there was significant mixing of material within the protoplanetary disk. These objectives can be met by delivering a lander to the surface of a cometary nucleus.
Once at the surface, the lander will produce a borehole into which different instruments (scanning electron microscope, borehole optical imager, Terahertz tomo-/spectrograph, Raman/LIBS) will be lowered to examine the physico-chemical structure of pristine material. These measurements will be complemented by a high-frequency ground penetrating radar and a Laser Ablation Mass Spectrometer. Panoramic cameras and an environmental package will deliver the key context of the landing site.
By providing in-situ and remote sensing measurements of the most pristine material remaining in our Solar System ORIGO will directly constrain the earliest phases of planet formation when the precursors of comets formed.
The original contributions presented in the study are included in the article/Supplementary Material, further inquiries can be directed to the corresponding author.
All authors listed have made a substantial, direct, and intellectual contribution to the work and approved it for publication.
Chimera development has received funding from the European Union’s Horizon 2020 research and innovation program under grant agreement No. 870377 (project NEO-MAPP).
RM acknowledges the funding from the European Research Council (ERC) under the European Union’s Horizon 2020 research and innovation programme (Grant agreement No. 101019380).
AF was empolyed by the Airbus Defence and Space GmbH. FC was empolyed by the GMV.
The remaining authors declare that the research was conducted in the absence of any commercial or financial relationships that could be construed as a potential conflict of interest.
All claims expressed in this article are solely those of the authors and do not necessarily represent those of their affiliated organizations, or those of the publisher, the editors and the reviewers. Any product that may be evaluated in this article, or claim that may be made by its manufacturer, is not guaranteed or endorsed by the publisher.
1https://www.cosmos.esa.int/web/voyage-2050.
Abod, C. P., Simon, J. B., Li, R., Armitage, P. J., Youdin, A. N., and Kretke, K. A. (2019). The mass and size distribution of planetesimals formed by the streaming instability. II. The effect of the radial gas pressure gradient. ApJ 883, 192. doi:10.3847/1538-4357/ab40a3
A’Hearn, M. F., Belton, M. J. S., Delamere, A., and Blume, W. H. (2005). Deep Impact: A large-scale active experiment on a cometary nucleus. Space Sci. Rev. 117, 1–21. doi:10.1007/s11214-005-3387-3
A’Hearn, M. F., Belton, M. J. S., Delamere, W. A., Feaga, L. M., Hampton, D., Kissel, J., et al. (2011). EPOXI at comet hartley 2. Science 332, 1396–1400. doi:10.1126/science.1204054
Attree, N., Groussin, O., Jorda, L., Nebouy, D., Thomas, N., Brouet, Y., et al. (2018). Tensile strength of 67P/Churyumov–Gerasimenko nucleus material from overhangs. A&A 611, 611A33. doi:10.1051/0004-6361/201732155
Ball, A. J., Blancquaert, T., Bayle, O., Lorenzoni, L. V., and Haldemann, A. F. C.the Schiaparelli EDM team (2022). The ExoMars schiaparelli entry, descent and landing demonstrator module (EDM) system design. Space Sci. Rev. 218, 44. doi:10.1007/s11214-022-00898-z
Banerdt, W. B., Smrekar, S. E., Banfield, D., Giardini, D., Golombek, M., Johnson, C. L., et al. (2020). Initial results from the InSight mission on Mars. Nat. Geosci. 13, 183–189. doi:10.1038/s41561-020-0544-y
Bibring, J. P., Lamy, P., Langevin, Y., Soufflot, A., Berthe, M., Borg, J., et al. (2007a). Space Sci. Rev. 128, 397–412. doi:10.1007/s11214-006-9135-5
Bibring, J. P., Rosenbauer, H., Boehnhardt, H., Ulamec, S., Biele, J., Espinasse, S., et al. (2007b). The Rosetta lander (“Philae”) investigations. Space Sci. Rev. 128, 205–220. doi:10.1007/s11214-006-9138-2
Biele, J., and Ulamec, S. (2009). in Origin and early evolution of comet nuclei. Editors H. Balsiger, K. Altwegg, W. Huebner, and T. Owen (New York: R. Schulz), 28, 275. doi:10.1007/978-0-387-85455-7_18
Blum, J., Bischoff, D., and Gundlach, B. (2022). formation of comets. Universe 8, 381. doi:10.3390/universe8070381
Blum, J. (2018). Dust evolution in protoplanetary discs and the formation of planetesimals. Space Sci. Rev. 214, 52. doi:10.1007/s11214-018-0486-5
Blum, J., Gundlach, B., Krause, M., Fulle, M., Johansen, A., Agarwal, J., et al. (2017). Evidence for the formation of comet 67P/Churyumov-Gerasimenko through gravitational collapse of a bound clump of pebbles. MNRAS 469, S755–S773. doi:10.1093/mnras/stx2741
Blum, J., Schräpler, R., Davidsson, B. J. R., and Trigo-Rodríguez, J. M. (2006). The physics of protoplanetesimal dust agglomerates. I. Mechanical properties and relations to primitive bodies in the solar system. ApJ 652, 1768–1781. doi:10.1086/508017
Blum, J., and Wurm, G. (2000). Experiments on sticking, restructuring, and fragmentation of preplanetary dust aggregates. Icarus 143, 138–146. doi:10.1006/icar.1999.6234
Cho, Y., Böttger, U., Rull, F., Hubers, H. W., Belenguer, T., Borner, A., et al. (2021). In situ science on Phobos with the Raman spectrometer for MMX (RAX): Preliminary design and feasibility of Raman measurements. Earth, Planets Space 73, 232. doi:10.1186/s40623-021-01496-z
Chumikov, A. E., Cheptsov, V. S., Wurz, P., Lasi, D., Jost, J., and Managadze, N. (2021). Design, characteristics and scientific tasks of the LASMA-LR laser ionization mass spectrometer onboard Luna-25 and Luna-27 space missions. Int. J. Mass Spectrom. 469, 116676. doi:10.1016/j.ijms.2021.116676
Ciarletti, V., Clifford, S., Plettemeier, D., Le Gall, A., Herve, Y., Dorizon, S., et al. (2017). The WISDOM radar: Unveiling the subsurface beneath the ExoMars rover and identifying the best locations for drilling. Astrobiology 17, 565–584. doi:10.1089/ast.2016.1532
Davidsson, B. J. R., Samarasinha, N. H., Farnocchia, D., and Gutiérrez, P. J. (2022). Modelling the water and carbon dioxide production rates of Comet 67P/Churyumov–Gerasimenko. MNRAS 509, 3065–3085. doi:10.1093/mnras/stab3191
Davidsson, B. J. R., Sierks, H., Güttler, C., Marzari, F., Pajola, M., Rickman, H., et al. (2016). The primordial nucleus of comet 67P/Churyumov-Gerasimenko. A&A 592, 592A63. doi:10.1051/0004-6361/201526968
de Niem, D., Kührt, E., Hviid, S., and Davidsson, B. (2018). Low velocity collisions of porous planetesimals in the early solar system. Icarus 301, 196–218. doi:10.1016/j.icarus.2017.09.024
De Sanctis, M. C., Altieri, F., Ammannito, E., Biondi, D., De Angelis, S., Meini, M., et al. (2017). Ma_MISS on ExoMars: Mineralogical characterization of the martian subsurface. Astrobiology 17, 612–620. doi:10.1089/ast.2016.1541
Di Lizia, P., Bernelli-Zazzera, F., Ercoli-Finzi, A., Mottola, S., Fantinati, C., Remetean, E., et al. (2016). Planning and implementation of the on-comet operations of the instrument SD2 onboard the lander Philae of Rosetta mission. Acta Astronaut. 125, 183-195. doi:10.1016/j.actaastro.2015.11.027
Dominik, C., and Tielens, A. G. G. M. (1997). The physics of dust coagulation and the structure of dust aggregates in space. ApJ 480, 647–673. doi:10.1086/303996
Dones, L., Brasser, R., Kaib, N., and Rickman, H. (2015). Origin and evolution of the cometary reservoirs. Space Sci. Rev. 197, 191–269. doi:10.1007/s11214-015-0223-2
Donn, B., and Hughes, D. (1986). “ESA special publication,” in ESLAB symposium on the exploration of Halley’s comet. Editors B. Battrick, E. J. Rolfe, and R. Reinhard, 250, 523.
Duncan, M. J. (2008). Dynamical origin of comets and their reservoirs. Space Sci. Rev. 138, 109–126. doi:10.1007/s11214-008-9405-5
Duncan, M., Levison, H., and Dones, L. (2004). in Comets II. Editors M. C. Festou, and H. U. Keller (Tucson: H. A. Weaver), 193.
Duncan, M., Quinn, T., and Tremaine, S. (1988). The origin of short-period comets. ApJ 328, L69. doi:10.1086/185162
Esposito, F., Debei, S., Bettanini, C., Molfese, C., Arruego Rodriguez, I., Colombatti, G., et al. (2018). The DREAMS experiment onboard the schiaparelli module of the ExoMars 2016 mission: Design, performances and expected results. Space Sci. Rev. 214, 103. doi:10.1007/s11214-018-0535-0
Fabre, C., Maurice, S., Cousin, A., Wiens, R., Forni, O., Sautter, V., et al. (2011). Onboard calibration igneous targets for the Mars Science Laboratory Curiosity rover and the Chemistry Camera laser induced breakdown spectroscopy instrument. Spectrochim. Acta 66, 280–289. doi:10.1016/j.sab.2011.03.012
Fougere, N., Altwegg, K., Berthelier, J. J., Bieler, A., Bockelee-Morvan, D., Calmonte, U., et al. (2016). Direct Simulation Monte Carlo modelling of the major species in the coma of comet 67P/Churyumov-Gerasimenko. MNRAS 462, S156–S169. doi:10.1093/mnras/stw2388
Gasc, S., Altwegg, K., Balsiger, H., Berthelier, J. J., Bieler, A., Calmonte, U., et al. (2017). Change of outgassing pattern of 67P/Churyumov–Gerasimenko during the March 2016 equinox as seen by ROSINA. MNRAS 469, S108–S117. doi:10.1093/mnras/stx1412
Goldreich, P., and Ward, W. R. (1973). The Formation of planetesimals. ApJ 183, 1051. doi:10.1086/152291
Groussin, O., Jorda, L., Auger, A. T., Kuhrt, E., Gaskell, R., Capanna, C., et al. (2015). Gravitational slopes, geomorphology, and material strengths of the nucleus of comet 67P/Churyumov-Gerasimenko from OSIRIS observations. A&A 583, A32. doi:10.1051/0004-6361/201526379
Güttler, C., Blum, J., Zsom, A., Ormel, C. W., and Dullemond, C. P. (2010). A&A 513, 513A56. doi:10.1051/0004-6361/200912852
Herique, A., Plettemeier, D., Lange, C., Grundmann, J. T., Ciarletti, V., Ho, T. M., et al. (2019). A radar package for asteroid subsurface investigations: Implications of implementing and integration into the MASCOT nanoscale landing platform from science requirements to baseline design. Acta Astronaut. 156, 317–329. doi:10.1016/j.actaastro.2018.03.058
Herny, C., Mousis, O., Marschall, R., Thomas, N., Rubin, M., Pinzon-Rodriguez, O., et al. (2021). New constraints on the chemical composition and outgassing of 67P/Churyumov-Gerasimenko. Space Sci. 200, 105194. doi:10.1016/j.pss.2021.105194
Ho, T.-M., Baturkin, V., Grimm, C., Grundmann, J. T., Hobbie, C., Ksenik, E., et al. (2017). MASCOT—the mobile asteroid surface scout onboard the Hayabusa 2 mission. Space Sci. Rev. 208, 339–374. doi:10.1007/s11214-016-0251-6
Jaumann, R., Schmitz, N., Koncz, A., Michaelis, H., Schroeder, S. E., Mottola, S., et al. (2017). The camera of the MASCOT asteroid lander on board Hayabusa 2. Space Sci. Rev. 208, 375–400. doi:10.1007/s11214-016-0263-2
Johansen, A., Blum, J., Tanaka, H., Ormel, C., Bizzarro, M., and Rickman, H. (2014). in Protostars and planets VI. Editors H. Beuther, R. S. Klessen, C. P. Dullemond, and T. Henning, 547. doi:10.2458/azu_uapress_9780816531240-ch024
Johansen, A., Oishi, J. S., Mac Low, M.-M., Klahr, H., Henning, T., and Youdin, A. (2007). Rapid planetesimal formation in turbulent circumstellar disks. Nature 448, 1022–1025. doi:10.1038/nature06086
Jutzi, M., and Michel, P. (2020). Collisional heating and compaction of small bodies: Constraints for their origin and evolution. Icarus 350, 113867. doi:10.1016/j.icarus.2020.113867
Kataoka, A., Tanaka, H., Okuzumi, S., and Wada, K. (2013). Fluffy dust forms icy planetesimals by static compression. A&A 557, 557L4. doi:10.1051/0004-6361/201322151
Keller, H. U., and Kührt, E. (2020). Cometary nuclei—from Giotto to Rosetta. Space Sci. Rev. 216, 14. doi:10.1007/s11214-020-0634-6
Kenyon, S. J., and Luu, J. X. (1998). Accretion in the early kuiper Belt. I. Coagulation and velocity evolution. AJ 115, 2136–2160. doi:10.1086/300331
Kereszturi, A., Duvet, L., Gróf, G., Gyenis, A., Gyenis, T., Kapui, Z., et al. (2022). Optical borehole-wall analysis – useful method for planetary environment reconstruction. Acta Astronaut. 196, 57–72. doi:10.1016/j.actaastro.2022.03.034
Lebreton, J.-P., and Matson, D. L. (2003). in The huygens probe: Science, payload and mission overview. Editor C. T. Russell (Dordrecht: Springer Netherlands), 59–100. doi:10.1007/978-94-017-3251-2_2
Levison, H. F., and Duncan, M. (1997). From the kuiper Belt to jupiter-family comets: The spatial distribution of ecliptic comets. Icarus 127, 13–32. doi:10.1006/icar.1996.5637
Managadze, G. G., Wurz, P., Sagdeev, R. Z., Chumikov, A. E., Tuley, M., Yakovleva, M., et al. (2010). Study of the main geochemical characteristics of Phobos’ regolith using laser time-of-flight mass spectrometry. Sol. Syst. Res. 44, 376–384. doi:10.1134/S0038094610050047
Manrique, J. A., Lopez-Reyes, G., Cousin, A., Rull, F., Maurice, S., Wiens, R. C., et al. (2020). SuperCam calibration targets: Design and development. Space Sci. Rev. 216, 138. doi:10.1007/s11214-020-00764-w
Martinez, J. E., Own, C. S., Galeano, M. P., Weppelman, G., and Pettit, D. R. (2019). Flight readiness of Mochii S: Portable spectroscopic scanning electron microscope facility on the international space station (ISS). Microsc. Microanal. 25, 700–701. doi:10.1017/S1431927619004239
Morse, A., Mousis, O., Sheridan, S., Morgan, G., Andrews, D., Barber, S., et al. (2015). Low CO/CO2ratios of comet 67P measured at the Abydos landing site by thePtolemymass spectrometer. A&A 583, 583A42. doi:10.1051/0004-6361/201526624
Mottola, S., Arnold, G., Grothues, H.-G., Jaumann, R., Michaelis, H., Neukum, G., et al. (2007). The rolis experiment on the Rosetta lander. Space Sci. Rev. 128, 241–255. doi:10.1007/s11214-006-9004-2
Nesvorný, D. (2018). Dynamical evolution of the early solar system. ARA&A 56, 137–174. doi:10.1146/annurev-astro-081817-052028
Nesvorný, D., Vokrouhlický, D., and Roig, F. (2016). The orbital distribution of trans-neptunian objects beyond 50 au. ApJ 827, 827L35. doi:10.3847/2041-8205/827/2/L35
Ohtsuki, K. (2012). Collisions and gravitational interactions between particles in planetary rings. Prog. Theor. Phys. Suppl. 195, 29–47. doi:10.1143/PTPS.195.29
Opik, E. (1961). Symposium: Comets: The survival of comets and cometary material. AJ 66, 381. doi:10.1086/108441
Own, C., Martinez, J., DeRego, T., Own, L., Morales, Z., Thomas-Keprta, K., et al. (2020). Mochii ISS-nl: Electron microscopy has arrived at the international space station. Microsc. Microanal. 26, 1578–1581. doi:10.1017/S1431927620018590
Plettemeier, D., Ciarletti, V., and Hamran, S.-E. (2009). 2009 IEEE radar conference, 1–6. doi:10.1109/RADAR.2009.4977120
Rayman, M. D. (2002). The Deep Space 1 extended mission: Challenges in preparing for an encounter with comet Borrelly. Acta Astronaut. 51, 507–516. doi:10.1016/S0094-5765(02)00070-X
Re, E., Magnani, P., Izzo, M., et al. (2008). in 10th workshop on advanced space technologies for Robotics and automation, noordwijk (Netherlands).
Reichhardt, T. (1995). US space agency escapes the worst of cuts. Nature 378, 325. doi:10.1038/378325a0
Reinhard, R. (1987). The Giotto mission to comet Halley. J. Phys. E Sci. Instrum. 20, 700–712. doi:10.1088/0022-3735/20/6/029
Reinhard, R. (1982). The Giotto mission to Halley's comet. Adv. Space Res. 2, 97–107. doi:10.1016/0273-1177(82)90293-9
Roll, R., Witte, L., and Arnold, W. (2016). ROSETTA lander Philae – soil strength analysis. Icarus 280, 359–365. doi:10.1016/j.icarus.2016.07.004
Sagdeev, R. Z., Avanesov, G. A., Barinov, I. V., Debabov, A., Kvasikov, V., Moroz, V., et al. (1985). Comet Halley: Nucleus and jets (results of the VEGA mission). Adv. Space Res. 5, 95–104. doi:10.1016/0273-1177(85)90072-9
Schäfer, U., Yang, C.-C., and Johansen, A. (2017). Initial mass function of planetesimals formed by the streaming instability. A&A 597, A69. doi:10.1051/0004-6361/201629561
Schräpler, R., Blum, J., Krijt, S., and Raabe, J.-H. (2018). The physics of protoplanetary dust agglomerates. X. High-Velocity collisions between small and large dust agglomerates as a growth barrier. ApJ 853, 74. doi:10.3847/1538-4357/aaa0d2
Shi, X., Castillo-Rogez, J., Hsieh, H., Hui, H., Ip, W. H., Lei, H., et al. (2021). Gauss - Genesis of asteroids and evolution of the solar system. Exp. Astron. doi:10.1007/s10686-021-09800-1
Simon, J. B., Armitage, P. J., Li, R., and Youdin, A. N. (2016). ApJ 822, 55. doi:10.3847/0004-637X/822/1/55
Simon, J. B., Armitage, P. J., Youdin, A. N., and Li, R. (2017). Evidence for universality in the initial planetesimal mass function. ApJ 847, 847L12. doi:10.3847/2041-8213/aa8c79
Skorov, Y., and Blum, J. (2012). Dust release and tensile strength of the non-volatile layer of cometary nuclei. Icarus 221, 1–11. doi:10.1016/j.icarus.2012.01.012
Snodgrass, C., and Jones, G. H. (2019). The European space agency’s comet interceptor lies in wait. Nat. Commun. 10, 5418. doi:10.1038/s41467-019-13470-1
Squyres, S. W., Nakamura-Messenger, K., Mitchell, D. F., Moran, V. E., Houghton, M. B., Glavin, D. P., et al. (2018). in 49th annual lunar and planetary science conference, lunar and planetary science conference, 1332.
Taylor, M. G. G. T., Altobelli, N., Buratti, B. J., and Choukroun, M. (2017). The Rosetta mission orbiter science overview: The comet phase. Philosophical Trans. R. Soc. Lond. Ser. A 375, 20160262. doi:10.1098/rsta.2016.0262
Ulamec, S., Fantinati, C., Maibaum, M., Geurts, K., Biele, J., Jansen, S., et al. (2016). Rosetta lander – landing and operations on comet 67P/Churyumov–Gerasimenko. Acta Astronaut. 125, 80–91. doi:10.1016/j.actaastro.2015.11.029
Ulamec, S., O’Rourke, L., Biele, J., Grieger, B., Andres, R., Lodiot, S., et al. (2017). Rosetta Lander - Philae: Operations on comet 67P/Churyumov-Gerasimenko, analysis of wake-up activities and final state. Acta Astronaut. 137, 38–43. doi:10.1016/j.actaastro.2017.04.005
Veneranda, M., Manrique-Martinez, J. A., Garcia-Prieto, C., Sanz-Arranz, A., Saiz, J., Lalla, E., et al. (2021). Raman semi-quantification on Mars: ExoMars RLS system as a tool to better comprehend the geological evolution of martian crust. Icarus 367, 114542. doi:10.1016/j.icarus.2021.114542
Vincent, J.-B., Bodewits, D., Besse, S., Sierks, H., Barbieri, C., Lamy, P., et al. (2015). Large heterogeneities in comet 67P as revealed by active pits from sinkhole collapse. Nature 523, 63–66. doi:10.1038/nature14564
Wada, K., Tanaka, H., Suyama, T., Kimura, H., and Yamamoto, T. (2008). Numerical simulation of dust aggregate collisions. II. Compression and disruption of three-dimensional aggregates in head-on collisions. ApJ 677, 1296–1308. doi:10.1086/529511
Wahlberg Jansson, K., Johansen, A., Bukhari Syed, M., and Blum, J. (2017). ApJ 835, 109. doi:10.3847/1538-4357/835/1/109
Wahlberg Jansson, K., and Johansen, A. (2014). Formation of pebble-pile planetesimals. A&A 570, 570A47. doi:10.1051/0004-6361/201424369
Weidenschilling, S. (1977). Aerodynamics of solid bodies in the solar nebula. MNRAS 180, 57–70. doi:10.1093/mnras/180.2.57
Weissman, P., Morbidelli, A., Davidsson, B., and Blum, J. (2020). Origin and evolution of cometary nuclei. Space Sci. Rev. 216, 6. doi:10.1007/s11214-019-0625-7
Windmark, F., Birnstiel, T., Güttler, C., Blum, J., Dullemond, C. P., and Henning, T. (2012a). Planetesimal formation by sweep-up: How the bouncing barrier can be beneficial to growth. A&A 540, 540A73. doi:10.1051/0004-6361/201118475
Windmark, F., Birnstiel, T., Ormel, C. W., and Dullemond, C. P. (2012b). Breaking through: The effects of a velocity distribution on barriers to dust growth. A&A 544, L16. doi:10.1051/0004-6361/201220004
Yang, C.-C., Johansen, A., and Carrera, D. (2017). Concentrating small particles in protoplanetary disks through the streaming instability. A&A 606, 606A80. doi:10.1051/0004-6361/201630106
Youdin, A. N., and Goodman, J. (2005). Streaming instabilities in protoplanetary disks. ApJ 620, 459–469. doi:10.1086/426895
Keywords: mission, comet, planetesimal, formation, lander and rover
Citation: Marschall R, Thomas N, Ulamec S, Hviid S, Mottola S, Vincent J-B, Ferri F, Herique A, Plettemeier D, Kereszturi Á, Lavagna MR, Prinetto J, Dottori A, Falke A and da Silva Pais Cabral F (2023) ORIGO: A mission concept to challenge planetesimal formation theories. Front. Space Technol. 3:1054360. doi: 10.3389/frspt.2022.1054360
Received: 26 September 2022; Accepted: 25 November 2022;
Published: 13 January 2023.
Edited by:
Josep M. Trigo-Rodríguez, Institute of Space Sciences, Spanish National Research Council (CSIC), SpainReviewed by:
Koki Ho, Georgia Institute of Technology, United StatesCopyright © 2023 Marschall, Thomas, Ulamec, Hviid, Mottola, Vincent, Ferri, Herique, Plettemeier, Kereszturi, Lavagna, Prinetto, Dottori, Falke and da Silva Pais Cabral. This is an open-access article distributed under the terms of the Creative Commons Attribution License (CC BY). The use, distribution or reproduction in other forums is permitted, provided the original author(s) and the copyright owner(s) are credited and that the original publication in this journal is cited, in accordance with accepted academic practice. No use, distribution or reproduction is permitted which does not comply with these terms.
*Correspondence: Raphael Marschall, cmFwaGFlbC5tYXJzY2hhbGxAb2NhLmV1
Disclaimer: All claims expressed in this article are solely those of the authors and do not necessarily represent those of their affiliated organizations, or those of the publisher, the editors and the reviewers. Any product that may be evaluated in this article or claim that may be made by its manufacturer is not guaranteed or endorsed by the publisher.
Research integrity at Frontiers
Learn more about the work of our research integrity team to safeguard the quality of each article we publish.