- 1German Aerospace Center (DLR), Institute of Planetary Research, Berlin, Germany
- 2FB Geowissenschaften, Institut für Geologische Wissenschaften Planetologie und Fernerkundung, Freie Universität Berlin, Berlin, Germany
In 2022 JAXA issued an Announcement of Opportunity (AO) for receiving Hayabusa2 samples returned to Earth. We responded to the AO submitting a proposal based on using a multi-prong approach to achieve two main goals. The first goal is to address the subdued contrast of remote-sensing observations compared to measurements performed under laboratory conditions on analog materials. For this we will link the hyperspectral and imaging data collected from the spacecraft and the in-situ observations from the MASCOT lander instruments (MARA and MASCam) with laboratory-based measurements of Hayabusa2 samples using bi-directional reflectance spectroscopy under simulated asteroid surface conditions from UV to MIR/FIR achieved using three Bruker Vertex 80 V spectrometers in the Planetary Spectroscopy Laboratory. The second goal is the investigation of the mineralogy and organic matter of the samples collected by Hayabusa2, to better understanding the evolution of materials characterizing Ryugu and in general of protoplanetary disk and organic matter, investigating the aqueous alteration that took place in the parent body, and comparing the results with data collected from pristine carbonaceous chondrite analog meteorites. Spectral data will be complemented by Raman spectroscopy under simulated asteroid surface conditions, X-ray diffraction, would also allow us to define the bulk mineralogy of the samples as well as investigate the presence and nature of organic matter within the samples. In situ mineralogical and geochemical characterization will involve a pre-characterization of the sample fragments through scanning electron microscopy low voltage electron dispersive X-ray (EDX) maps, and micro IR analyses of the fragments. If allowed, a thin section of one grain will be used for electron microprobe analyses to geochemically characterize its mineralogical composition. To train our data collection and analysis methods on a realistic sample, we selected a piece of the Mukundpura meteorite, as one of the closer analogs to Ryugu’s surface (Ray et al., Planetary and Space Science, 2018, 151, 149–154). The Mukundpura chunk we selected for this study measures 3 mm in its maximum dimension, and we chose it so to have a test sample of the same size as the Hayabusa2 grain we requested in our proposal to JAXA’s AO. The test gave us confidence that we can measure with good SNR measurements in bi-directional reflectance for samples around 3 mm in size (see Figures 3, 4 below). To address our second goal the spectral data was complemented by Raman spectroscopy measured again under simulated asteroid surface conditions in our Raman Mineralogy and Biodetection Laboratory at DLR.
1 Introduction
The past decades of planetary exploration have been dominated by remote sensing because global reconnaissance of a planetary surface can only be obtained by remote sensing methods.
Hyperspectral (and to some degree multispectral) remote sensing methods are playing a key role in determining surface mineralogy, texture, weathering processes, volatile abundances and much more. It is a very versatile technique, which will continue to be of importance for many years to come. In the last decades we are seeing a shift towards in-situ exploration and sample return missions led by JAXA. Although we can learn a large amount about planetary bodies by remote sensing with cameras, spectrometers, and other instruments, and we can obtain complementary information from in-situ exploration, most sample science cannot be done this way. Exquisitely sensitive laboratory instruments on Earth are capable of determining the chemical, isotopic, mineralogical, structural, and physical properties of extraterrestrial samples from the macroscopic level down to the atomic scale, frequently all on the very same sample. This allows us to determine the origin and history of the material and answer questions far beyond the reach of current robotic technology. Moreover, sample return provides us with “ground truth” about the visited body, verifying and validating conclusions that can be drawn by remote sensing (both Earth-based and by spacecraft) and via landed instruments on other bodies. In addition, returned samples can be compared to astromaterials such as meteorites and cosmic dust using the same instrumentation, which apart from giving us clues about where those materials come from, potentially increases their scientific value as natural space probes. And finally, returned samples can be preserved for decades and used by future generations to answer questions we have not even thought of yet using laboratory instruments that have not even been imagined.
The detailed investigation of the mineralogy and geochemistry of Ryugu plays a fundamental role in the understanding of its formation processes, and thereby gather further knowledge about the building blocks of the Solar System. Based on the preliminary data, first from remote sensing measurements and then from laboratory-based measurements, Ryugu is rich in hydrated carbonaceous chondrite (CC) like material and more specifically it is very similar to Ivuna-like (CI) carbonaceous chondrites (Yada et al., 2022). These meteorites are characterized by a high abundance of phyllosilicates and organic matter (Cloutis et al., 2011), which makes them have a low albedo. However, Ryugu seems to be even darker than CIs, as well as being more porous and fragile (Yada et al., 2022).
Space weathering is not considered to be affecting the fundamental parameters used for mineralogical characterization, such as absorption band centers and relative band area (Gaffey et al., 2010), but rather the albedo. It is more likely that Ryugu surface had been modified by solar heating, as it also affects the asteroid surface at higher depths in comparison to space weathering, and it is also responsible for the reddening of Ryugu’s surface (Morota et al., 2020).
The surface of Ryugu is continuously space weathering, such as high-energy photon and ion radiation (e.g., Lantz et al., 2017.), and micro-meteorite impacts which will darken and redden the spectra and produce dust (Szalay & Horanyi, 2016). Furthermore, high temperatures can lead to thermal metamorphism (Hiroi et al., 1996) and dehydration of material (e.g., Bates et al., 2021). Thermal gradients leading to thermal fatigue which will gradually degrade the boulders to smaller particles which can influence the spectra of the surface material (Aronson & Emslie, 1974; Molaro et al., 2020).
Based on remote sensing the surface material of Ryugu was expected to be partially dehydrated (Kitazato 2019; 2021; Sugita et al., 2019). However, in-situ observations my MASCOT showed no measurable dust layer (Grott et al., 2019; Jaumann et al., 2019), and similarity to the aqueously altered CI chondrites (Hamm et al., 2022). These results were substantiated by the initial analysis of Ryugus samples from both sampling sites (Yada et al., 2022), implying that dehydration was not as effective on Ryugu as initially believed and that the samples analyzed by Yada et al., 2022 are comparable to the boulder surface observed by MASCOT. A more detailed study of the samples of Ryugu and their comparison to results from MASCOT which observed an exposed part of a common boulder on Ryugu, will corroborate the understanding of Ryugus surface evolution.
With this proposal we plan to use a multi-prong approach to achieve two main goals. The first goal is to address a fundamental challenge in the interpretation of remote sensing data which has also been seen during the initial analysis of the Hayabusa2 samples. Observations of planetary surfaces using spectroscopy have shown over and over again subdued contrast compared to measurements performed under laboratory conditions on analog materials. A strong focus of the work performed at PSL over the last decade has been to understand - and if possible minimize - the difference between laboratory and remote sensing observations (e.g., Maturilli et al., 2016 a; b; Beck et al., 2018; Yesiltas et al., 2020). Simulating the conditions on the target body as well as accurately reproducing the observing geometries have gone a long way towards that goal, however differences remain. A possible explanation was always the difference between terrestrial analog materials including even meteorites and the surfaces of planetary bodies. With Ryugu samples this hypothesis can be tested further, leading to a deeper understanding of the link between laboratory and remote sensing observing and thus benefiting not only the analysis of Hayabusa2 data but of all remote sensing observations of planetary surfaces using spectroscopy.
The second goal building on this is an investigation of the mineralogy and organic matter of the samples collected by Hayabusa2, to better: 1) understanding the evolution of the materials characterizing asteroid Ryugu and therefore advance our knowledge of the mineralogy of the protoplanetary disk and organic matter (OM); 2) investigating the aqueous alteration that took place in the parent body that led to its current chemical and mineralogical characteristics; 3) comparing the results with data collected from pristine carbonaceous chondrite meteorites rich in hydrated minerals and organic matter.
For achieving our first main objective, we plan to link the hyperspectral and imaging data collected from the spacecraft and the in-situ observations from the MASCOT lander instruments with laboratory-based measurements of Hayabusa2 samples using bi-directional reflectance spectroscopy under simulated asteroid surface conditions from UV to MIR/FIR achieved using three Bruker Vertex 80 V spectrometers in the Planetary Spectroscopy Laboratory. Covering this wide spectral range will allow accessing complementary sets of spectral characteristics to assess composition. For the bi-directional spectroscopy measurements, we plan to reproduce as closely as possible the observing conditions under which NIRS3 and the TIR imager on Hayabusa2 as well as MARA and MASCam on MASCOT observed Ryugu (e.g., Grott et al., 2019; Jaumann et al., 2019; Kitazato et al., 2019; Okada et al., 2020; Hamm et al., 2022). This will be complemented by high resolution hyperspectral mapping obtained with a Bruker Hyperion 2000 system. Using a Linkam cooling/heating stage we can again reproduce the surface conditions on the asteroid.
The part of the surface imaged by MASCam on Ryugu is only a local observation and may not be representative of the entire surface material. To characterize and assess the representativeness of the observed scenery it is necessary to compare the images with the returned samples. Vice versa this will also allow us to assess how representative the returned samples are for the general Ryugu surface. Using images from MASCam on Ryugu as well as on the returned samples and meteorites (using the MASCam Qualification Model), we aim to compare regolith and sample fragments geologically and morphologically. If existent, this will include an analysis of inclusion shapes, size frequency distributions and color as well as surface roughness, shapes and morphologic descriptors of the fragments (Otto et al., 2021; 2020; Schröder et al., 2021). In addition, we will be able to compare the morphology of the pristine materials from Ryugu with weathered meteorite samples which will allow us to assess the effect of terrestrial weathering on the samples’ morphology. Based on the MASCam pixel resolution of ∼200 µm we also envisage to examine sample and meteorite morphologies at high resolution complemented by the microscopic images using the Keyence VHX-7000 3D digital microscope at an even higher resolution. This will allow us to link high resolution laboratory data with real in-situ images and establish their morphologic connection spanning a wide range of spatial scales. We will also be able to include the investigation of a third dimension on the returned samples and meteorites which is only indirectly deduced from in-situ or laboratory images allowing us to access the quality of deduced three-dimensional data.
This first objective mainly drives the selection of samples. Based on preliminary investigations using a piece of the Mukundpura meteorite we can obtain good SNR measurements in bi-directional reflectance for samples larger than 3 mm (see Figures 3, 4 below). In addition, it would be beneficial if at least some of the samples have already been spectrally analyzed during the initial investigation phase.
To address our second goal the spectral data will be complemented by Raman spectroscopy again under simulated asteroid surface conditions in our Raman Mineralogy and Biodetection Laboratory at DLR. This, with the support of X-ray diffraction, would also allow us to define the bulk mineralogy of the samples as well as investigate the presence and nature of organic matter within the samples.
Complementary to the spectroscopy investigation we will carry out in-situ mineralogical and geochemical characterization of the returned material to identify the OM contained in the samples and investigate their relationship with the surrounding mineralogy. In-situ analyses will involve a pre-characterization of the sample fragments through scanning electron microscopy (SEM), low voltage electron dispersive X-ray (EDX) maps, and micro IR analyses of the fragments at DLR and at the Natural History Museum in Berlin. Moreover, at the Natural History Museum in Berlin, one sample in the form of a thin section (if permitted by the Hayabusa2 sample allocation committee) will be used for electron microprobe analyses (EMPA) to geochemically characterize its mineralogical composition.
This approach is already being applied for bulk and in-situ study of primitive carbonaceous chondrite meteorites, and therefore it would be of great impact to compare data collected from a pristine asteroid sample with meteoritic materials which has been studied for the past decades. This would also allow us to infer if there is any influence, both at micro and nanometer scale, to the mineralogy and organic matter due to the re-entry of meteoritic material through the atmosphere.
For this analysis, grains with a size of approximately 4 mm would be beneficial for the spectral investigations, therefore we have identified A0112, C0012, A0016, A0208, and C0011 as most suitable. Meanwhile JAXA selected our proposal and agreed to deliver the sample A0112 to our consortium; the sample will be available for analysis from around end of August 2022.
2 Research plan
The crucial point of our proposed work is to use the very large collection of analytical instruments in our consortium to measure the requested Hayabusa2 grains using many non-destructive techniques. Using our FTIR spectrometers (3 identical Bruker Vertex80V) at the Planetary Spectroscopy Laboratory (PSL) of DLR we can measure bi-directional bulk sample spectroscopy of the received Hayabusa2 samples completely under vacuum in the whole spectral range from UV to FIR (0.25 µm to at least 25 µm spectral range). A Bruker Hyperion 2000 FT-IR microscope allows mapping the Hayabusa2 grains from VIS to MIR (0.4 to at least 16 µm spectral range) with a spatial resolution down to 50 µm. The laboratory configuration can be seen in Figure 1; details on the instrumental set-up are discussed in Maturilli et al. (2019).
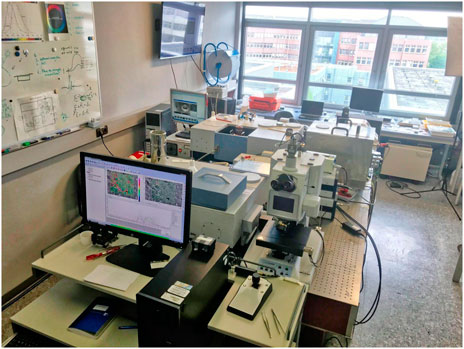
FIGURE 1. PSL configuration - Bruker Hyperion 2000 in the front right with a meteorite sample, on the left three Bruker Vertex 80V spectrometers with internal vacuum sample chambers, UV to FIR detectors, beamsplitter and sources and in the back a portable XRD system (orange box).
Under the best spectrometer optical set-up optimized for each wavelength range (UV + VIS + VNIR + MIR + FIR), bidirectional reflectance of Hayabusa2 samples will be measured in vacuum and under several illumination conditions using the spectrometer automatization features.
To prepare for measurements on Hayabusa2 returned samples, we selected a small particle from the Mukundpura meteorite, a recently fallen meteorite that is considered as one of the best Ryugu analogs in the meteorite collection (Ray&Shukla, 2018). We have chosen a piece with diameter 4mm, typically of the larger grains available in the Hayabusa2 sample collection. We manufactured for this purpose a special sample holder for reflectance measurements in the FTIR spectrometer. Figures 2, 3 show the small 4 mm selected Mukundpura particle compared with the whole Mukundpura sample available at PSL and the 4 mm particle in our sample holder.
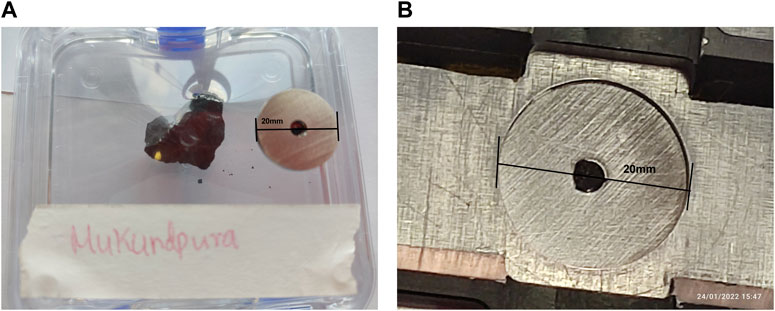
FIGURE 2. (left): 4 mm particle and the whole Mukundpura at PSL; Figure 2 (right): the 4 mm particle in our sample holder.
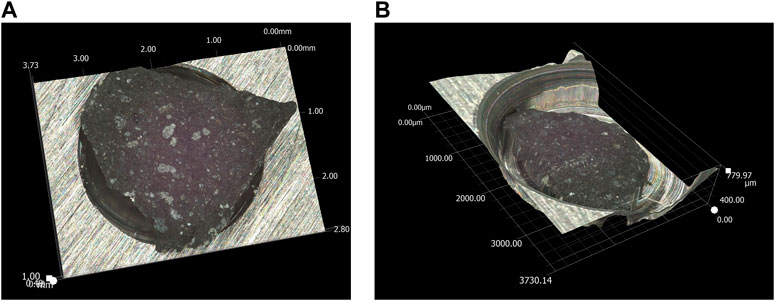
FIGURE 3. A Keyence 3D microscope will be used to acquire high resolution images and topographic maps of the samples-here shown an example with the Mukundpura test sample.
We adapted our standard measurement approach at PSL reducing the aperture to fit the size of the samples. With this measurement configuration we obtained measurements of our test sample to show the feasibility of our spectral method on Hayabusa2 samples. As an example of this, in Figure 4 we show the MIR spectrum of the 4 mm Mukundpura particle, obtained reducing the aperture of our light source beam to the minimum, 0.25 mm compared with a measurement of the same meteorite acquired with a much larger aperture (4mm, as standard). This figure shows that even after drastically reducing our beam aperture, we are able to obtain high quality FTIR spectra of an Hayabusa2 sample by just using our traditional spectroscopic set-up. Therefore, these spectra are directly comparable to the thousands of spectra obtained already at PSL on a wide range of analogs including meteorites.
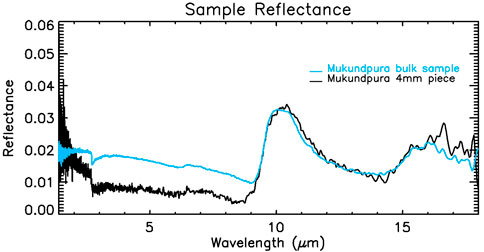
FIGURE 4. Mukundpura bulk sample measured with traditional beam aperture of 4 mm (light-blue) and with reduced aperture (0.25 mm) on the small 4 mm piece of the same meteorite. Below 9 µm we observe a lower reflectance of the piece compared to the bulk - reminiscent of what was observed during the preliminary investigation for the Hayabusa two sample.
As can be seen in Figure 4 the whole spectral shape between 9 and 18 µm is identical for the two curves. The major feature around 10 µm is perfectly comparable, even small features around 6.5 and 2.7 µm are detectable in the noisier measurement on the 4 mm Mukundpura particle. Below 9 µm we observe a lower reflectance of the piece compared to the bulk - reminiscent of what was observed during the preliminary investigation for the Hayabusa2 sample. These preliminary tests give us confidence that for particles of this size good SNR spectra can be obtained that are comparable to bulk sample measurements. Particle size is therefore one of the main drives in our selection of samples that we request for our analysis.
Once the Hayabusa2 grains are spectrally characterized, we could compare their spectra to our database of minerals to confirm remote sensing and preliminary analysis identifications, and furthermore compare their spectra to measurements of meteorites acquired in our PSL lab under exactly the same conditions as the Hayabusa2 samples.
The samples will be characterized in parallel with a Keyence VHX-7000 3D digital microscope with the VHX-7100 observation system that allows very high-resolution 3D mapping of samples and grain size analysis. An example of the type of imaging and sample topography that can be obtained is shown in Figure 3 for the Mukundpura test sample.
This analysis in the spectroscopy laboratory will be followed by measurements of the samples with the MASCOT MASCam qualification model (QM), thereby reproducing the imaging conditions on Ryugu during MASCOT’s surface mission. From a functional point of view, the MASCam QM is identical to the flight model.
To produce images most representative of the images of Ryugu’s surface and the carbonaceous chondrites samples acquired by MASCam previously, we will place the sample material approximately 20 cm in front of the camera to achieve a spatial resolution of about 0.2 mm per pixel. We will then illuminate the samples with four coloured LEDs in the visible wavelength mounted to the camera. Using a color composite image, we will map the inclusions within the samples and derive the inclusion brightness relative to the matrix in the red light, the relative spectral slope of the inclusions, the inclusion size frequency distribution and the matrix volume abundance.
While the available samples are too small to be measured with the MARA flight spare, at DLR the PSL laboratory MIR spectra of the sample will be resampled using the MASCOT MARA’s instrument functions to compare to the in-situ observations as reported in Hamm et al. (2022).
The comparison of remote sensing data from NIRS3, TIR and MARA with the laboratory spectral data collected under asteroidal conditions as well as the comparison of MASCam data from Ryugu with data obtained with the MASCam qualification model, will reveal unique insights into the relation between data obtained during the mission with laboratory data. Such measurements would also provide additional data for analyzing and improving the design of future spectrometers and cameras to be used on planetary surfaces. Specifically, for MASCam the performance on Ryugu and under controlled conditions in the lab on representative material can be studied in detail, e.g., regarding effective resolution, effect of compression on science analysis, confirmation of the radiometric calibration, etc. The design of future cameras with LED-illumination would benefit from studies regarding the angles of illumination. Using separate LEDs for each wavelength (like on MASCam) it changes the angles of illumination when changing wavelengths. The resulting spectra are difficult to calibrate. Multi-wavelengths LEDs would solve this problem, however there is no spaceflight heritage (yet) for these.
All this combined will be key to a better understanding of the nature of Ryugu because it allows (if necessary) to recalibrate the remote sensing datasets and link them to the actual ground truth obtained from the Hayabusa2 samples. At the same time, it will help the preparation of future missions, by obtaining a deeper understanding on how to translate laboratory measurements to instrument measurements, thereby improving performance predictions and future instrument designs.
Moreover, comparison of those data with spectroscopic data of meteorite samples, will be of fundamental help for understanding which processes affect meteorites during their atmospheric re-entry. This will help to understand whether we have a fundamental bias in analog spectral data sets obtained from our meteorite collections.
In order to investigate the nature of Ryugu deeper (and at the same time prepare for future data analysis from the JAXA MMX mission) the samples will be analyzed using Raman spectroscopy. Building on the experience gathered from the analysis of Hayabusa one particles by Raman microspectroscopy, we can measure micro particles under neutral atmosphere with our WiTec Alpha 300 confocal Raman microscope (Böttger et al., 2013b). Our Raman system is equipped with a ×10 long working distance objective with a 0.25 numerical aperture, a piezo-driven scan table, a UHTS 300 spectrometer with an ultrafast EMCCD detector, and a frequency-doubled Nd:YAG laser at 532 nm excitation wavelength. The spectral resolution is about 4 cm−1 with a 600 L/mm grating, and the spot diameter on the sample is about 1 µm. Measurement times can be varied to improve signal to noise ratios under low laser power (e.g., 200 µW as used for Hayabusa1 particles, Böttger et al., 2013a). Raman spectroscopy complements IR spectroscopy in the determination of the mineralogical composition of the sample, is performed in a contactless manner, and under neutral (e.g., nitrogen) atmosphere thanks to the long working distance objective. Figure 5 shows our tests on the Mukundpura particle; different measurement modes were used (single spectra, line scans, and area scans) to reveal the presence of olivine, carbon, and magnesium silicates.
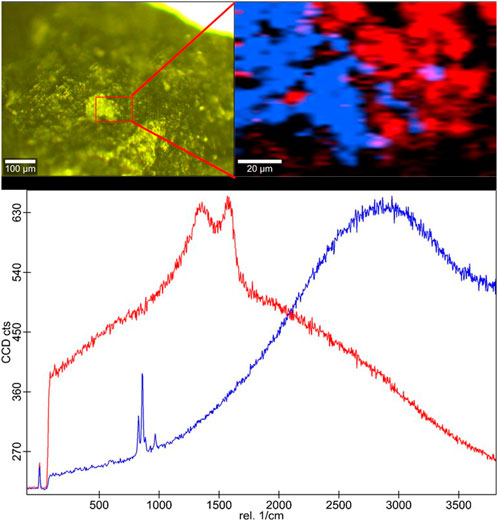
FIGURE 5. Example using the Mukundpura particle of an image scan of 30 points per line and 60 lines per image on a 120 × 80 µm area. The blue color denotes the presence of the olivine doublet (827 and 859 cm−1) and red the presence of carbon D and G bands (1,340 and 1,568 cm−1). The latter is in accordance with the abundance of organic matter in the chondrite discussed above.
Following the FTIR and Raman analyses, the particles will be characterized regarding their mineralogy and mineral chemistry using non-destructive scanning electron microscopy (SEM) and energy-dispersive X-ray spectrometry (EDS), whereas the bulk chemical compositions of the samples will be determined non-destructively by micro X-ray fluorescence (µXRF) analysis. In addition, electron microprobe techniques will be performed on a representative sample from which a section will be produced if permitted by the Hayabusa2 sample allocation committee. This complementary data is considered crucial for the interpretation of the results obtained by the spectroscopic methods, because it has to be anticipated that returned samples will be affected by space weathering and shock-metamorphic overprint caused by hypervelocity impacts (e.g., Kitazato et al., 2019; Noguchi et al., 2022; Thompson et al., 2022). Thus, a precise knowledge of potential shock histories and space-weathering effects is crucial for correct interpretation of acquired spectroscopic data. The Museum für Naturkunde Berlin (MfN) is equipped with state-of-the-art optical microscopes (e.g., a Leica M205C stereomicroscope), electron microscopes (a JEOL JSM-6610 LV and a Zeiss Evo LS 10), a field-emission electron microprobe (JEOL JXA-8500F), and preparation facilities that allow for handling, imaging, and precise micro-chemical analysis of the phases within the Hayabusa2 samples. These techniques have recently been successfully applied to characterize cosmic spherules in the form of urban micrometeorites, which are in the same size range as the requested Hayabusa2 samples. The MfN operates two µXRF spectrometers, one of which (a M4 Tornado Plus by Bruker) is capable of establishing the chemical compositions of millimeter-sized samples and their mineral components (Hamann et al., 2021). To test whether bulk compositions can be obtained from fine-grained, water-bearing, carbonaceous material by µXRF analysis, instrument tests on a slab of the Murchison CM2 carbonaceous chondrite as analog material for the material returned from Ryugu were performed. The MfN also hosts one of the largest meteorite collections worldwide, including material from CI1 and CM2 chondrites that may serve as analog material for the Hayabusa2 samples.
3 Sample history and handling
The Institute of Planetary Research of the German Aerospace Center (DLR) in Berlin has already gathered crucial experience on handling extraterrestrial samples including lunar samples, meteorites and samples from the first Hayabusa mission. DLR was part of one of the consortia receiving some of the Hayabusa one particles (Busemann et al., 2013). The Hayabusa one samples received in our institute were set in holes in a stainless-steel base in a special flange of stainless steel, which had been baked beforehand to decrease its background (Yada et al., 2014). The original container provided by JAXA was modified with a Quartz glass port to allow Raman measurements under nitrogen conditions (Böttger et al., 2013b). Figure 6 shows this sample container (used for Hayabusa1) in our laboratory: we intend to use the same sample container and the procedures already used at the time of Hayabusa one sample distribution to warrant obtaining the same results in the subject of safe sample preservation and transportation.
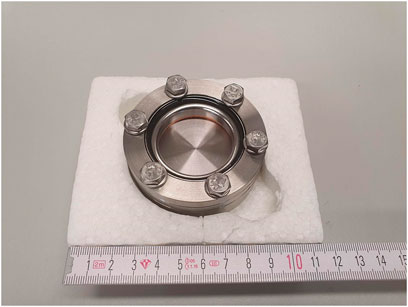
FIGURE 6. Hayabusa1 samples have been received, measured in the Raman laboratory in our institute (Böttger et al., 2013a), and successfully circulated to other partner of the 2013 consortium, giving us confidence in the procedures for receiving and storing the sample.
Once the samples arrive in our institute, it will be stored in a vacuum desiccator. Humidity and temperature sensors are located inside the desiccator, and those values are continuously read by a datalogger, allowing continuous real-time monitoring of the sample conditions. The samples will be removed from the desiccator only to perform spectroscopic measurements FTIR, Micro-FTIR, Raman and SEM analyses. For FTIR, the sample is placed inside the sample compartment of the spectrometer, that can be evacuated at 0.7 mbar, therefore the sample will be under vacuum during the whole measurement procedure. After the spectra acquisition is completed, the sample is immediately returned back to its sample container and stored back in the vacuum desiccator. FTIR, Micro-FTIR, Raman and SEM analyses are non-destructive techniques, also the samples will be returned back without damages that may occur from using high energy laser, contact with other specimens, or exposure to extreme temperatures Jaumann et al., 2017.
Data availability statement
The original contributions presented in the study are included in the article/Supplementary Material, further inquiries can be directed to the corresponding author.
Author contributions
AM is responsible for designing, performing and calibrating the FTIR spectroscopic measurements. SS is an expert on modeling of chemical diffusion to determine the thermal history of individual mineral grains. JH and GA are both co-responsible for designing, performing and calibrating the FTIR spectroscopic measurements. MD’A is responsible for data reduction of spectroscopic measurements. EB and GA are responsible for designing, performing and calibrating the Micro-FTIR spectroscopic measurements. MH, will be responsible for the comparison of MIR sample spectra to in-situ observations by MARA. MB is responsible for the Raman measurements and their calibration.
Funding
All the authors are funded by their national agencies, universities, and institutions.
Conflict of interest
The authors declare that the research was conducted in the absence of any commercial or financial relationships that could be construed as a potential conflict of interest.
Publisher’s note
All claims expressed in this article are solely those of the authors and do not necessarily represent those of their affiliated organizations, or those of the publisher, the editors and the reviewers. Any product that may be evaluated in this article, or claim that may be made by its manufacturer, is not guaranteed or endorsed by the publisher.
References
Aronson, J. R., and Emslie, A. G. (1973). Spectral reflectance and emittance of particulate materials. 2: Application and results. Appl. Opt. 12, 2573–2584. doi:10.1364/ao.12.002573
Bates, H. C., Donaldson Hanna, K. L., King, A. J., Bowles, N. E., and Russell, S. S. (2021). A spectral investigation of aqueously and thermally altered CM, CM-An, and CY chondrites under simulated asteroid conditions for comparison with OSIRIS-REx and Hayabusa2 observations. JGR. Planets 126, e2021JE006827. doi:10.1029/2021je006827
Beck, P., Maturilli, A., Garenne, A., Vernazza, P., Helbert, J., Quirico, E., et al. (2018). What is controlling the reflectance spectra (0.35–150 µm) of hydrated (and dehydrated) carbonaceous chondrites? Icarus 313, 124–138. doi:10.1016/j.icarus.2018.05.010
Böttger, U., Alwmark, C., Bajt, S., Busemann, H., Gilmour, J. D., Hübers, H.-M., et al. (2013a). Raman micro-spectroscopy of Hayabusa particles, Proceedings of the HAYABUSA 2013 symposium of solar systems materials, Tokyo.
Böttger, U., Alwmark, C., Bajt, S., Busemann, H., Gilmour, J. D., Hübers, H.-M., et al. (2013b). Raman microscopy of Hayabusa particle RA-QD02-0051, Proceedings of the 44th Lunar and Planetary Science Conference, The Woodlands, TX, March 2013.
Busemann, H., Alwmark, C., Bajt, S., Böttger, U., Gilmour, J. D., Heitmann, U., et al. (2013). A consortium status report: The characterisation of the asteroid itokawa regolith—a correlated study by X-Ray tomography, micro-raman spectroscopy, and high-sensitivity noble gas analysis. Proceedings of the 44th Lunar and Planetary Science Conference, The Woodlands, March 2013.
Cloutis, E. A., Hiroi, T., Gaffey, M. J., Alexander, C. O., and Mann, P. (2011). Spectral reflectance properties of carbonaceous chondrites: 1. CI chondrites. Icarus 212, 1 180–209. doi:10.1016/j.icarus.2010.12.009
Gaffey, M. (2010). Space weathering and the interpretation of asteroid reflectance spectra. Icarus 209 (2), 564–574. doi:10.1016/j.icarus.2010.05.006
Grott, M., Knollenberg, J., Hamm, M., Ogawa, K., Jaumann, R., Otto, K. A., et al. (2019). Low thermal conductivity boulder with high porosity identified on C-type asteroid (162173) Ryugu. Nat. Astron. 3, 971–976. doi:10.1038/s41550-019-0832-x
Hamann, C., Collinet, M., Schwinger, S., Kaufmann, F. E. D., Bonato, E., Greshake, A., et al. (2021). Petrography of fine-grained domains in ungrouped achondrite erg chech 002: Evidence for different cooling histories? 84th Annual Meeting of the Meteoritical Society, Chicago, Illinois, abstract #6236.
Hamm, M., Grott, M., Senshu, H., Knollenberg, J., de Wiljes, J., Hamilton, V. E., et al. (2022). Mid-infrared emissivity of partially dehydrated asteroid (162173) Ryugu shows strong signs of aqueous alteration. Nat. Commun. 13, 364. doi:10.1038/s41467-022-28051-y
Hiroi, T., Zolensky, M. E., Pieters, C. M., and Lipschutz, M. E. (1996). Thermal metamorphism of the C, G, B, and F asteroids seen from the 0.7 μm, 3 μm, and UV absorption strengths in comparison with carbonaceous chondrites. Meteorit. Planet. Sci. 31, 321–327. doi:10.1111/j.1945-5100.1996.tb02068.x
Jaumann, R., Schmitz, N., Ho, T. M., Schroder, S. E., Otto, K. A., Stephan, K., et al. (2019). Images from the surface of asteroid Ryugu show rocks similar to carbonaceous chondrite meteorites. Science 365, 817–820. doi:10.1126/science.aaw8627
Jaumann, R., Schmitz, N., Koncz, A., Michaelis, H., Schroeder, S. E., Mottola, S., et al. (2017). The camera of the MASCOT asteroid lander on board Hayabusa 2. Space Sci. Rev. 208, 375–400. doi:10.1007/s11214-016-0263-2
Kitazato, K., Milliken, R. E., Iwata, T., Abe, M., Ohtake, M., Matsuura, S., et al. (2021). Thermally altered subsurface material of asteroid (162173) Ryugu. Nat. Astron. 5, 246–250. doi:10.1038/s41550-020-01271-2
Kitazato, K., Milliken, R. E., Iwata, T., Abe, M., Ohtake, M., Matsuura, S., et al. (2019). The surface composition of asteroid 162173 Ryugu from Hayabusa2 near-infrared spectroscopy. Science eaav7432. doi:10.1126/science.aav7432
Lantz, C., Brunetto, R., Barucci, M. A., Fornasier, S., Baklouti, D., Bourçois, J., et al. (2017). Ion irradiation of carbonaceous chondrites: A new view of space weathering on primitive asteroids. Icarus 285, 43–57. doi:10.1016/j.icarus.2016.12.019
Maturilli, A., Helbert, J., and Arnold, G. (2019). The newly improved set-up at the Planetary Spectroscopy Laboratory (PSL). Proceedings Volume 11128, Infrared Remote Sensing and Instrumentation XXVII; 111280T, San Diego, CA: SPIE Optical Engineering + Applications. doi:10.1117/12.2529266
Maturilli, A., Helbert, J., Ferrari, S., and D’Amore, M. (2016). On the effect of emergence angle on emissivity spectra: Application to small bodies. Earth Planets Space 68 (1), 84. doi:10.1186/s40623-016-0464-7
Maturilli, A., Helbert, J., Ferrari, S., Davidsson, B., and D’Amore, M. (2016). Characterization of asteroid analogues by means of emission and reflectance spectroscopy in the 1- to 100-µm spectral range. Earth Planets Space 68 (1), 113. doi:10.1186/s40623-016-0489-y
Molaro, J., Walsh, K. J., Jawin, E. R., Ballouz, R. L., Bennett, C. A., DellaGiustina, D. N., et al. (2020). Evidence for thermally induced rock breakdown widespread on Bennu’s surface. Nat. Commun. 11 (1), 1–11. doi:10.1038/s41467-020-16528-7
Morota, T., Sugita, S., Cho, Y., Kanamaru, M., Tatsumi, E., Sakatani, N., et al. (2020). Sample collection from asteroid (162173) Ryugu by Hayabusa2: Implications for surface evolution. Science 368 (6491), 654–659. doi:10.1126/science.aaz6306
Noguchi, T., Matsumoto, T., Miyake, A., Miyake, Y., Miyake, M., Saito, H., et al. (2022). Mineralogy and space weathering of fine fraction recovered from asteroid (162173) Ryugu. 53rd Lunar and Planetary Science Conference, abstract #1747, The Woodlands, March 2022.
Okada, T., Fukuhara, T., Tanaka, S., Taguchi, M., Arai, T., Senshu, H., et al. (2020). Highly porous nature of a primitive asteroid revealed by thermal imaging. Nature 579, 518–522. doi:10.1038/s41586-020-2102-6
Otto, K. A., Matz, K. D., Schroder, S. E., Parekh, R., Krohn, K., Honda, R., et al. (2020). Surface roughness of asteroid (162173) Ryugu and comet 67P/Churyumov–Gerasimenko inferred from in situ observations. Mon. Not. R. Astron. Soc. 500, 3178–3193. doi:10.1093/mnras/staa3314
Otto, K. A., Schroder, S. E., Scharf, H. D., Greshake, A., Schmitz, N., Trauthan, F., et al. (2021). Spectral and petrographic properties of inclusions in carbonaceous chondrites and comparison with in situ images from asteroid Ryugu. Planet. Sci. J. 2, 188. doi:10.3847/PSJ/ac034b
Ray, D., and Shukla, A. D. (2018). The Mukundpura meteorite, a new fall of CM chondrite. Planet. Space Sci. 151, 149–154. doi:10.1016/j.pss.2017.11.005
Schröder, S., Otto, K., Scharf, H., Matz, K. D., Schmitz, N., Scholten, F., et al. (2021). Spectrophotometric analysis of the Ryugu rock seen by MASCOT: Searching for a carbonaceous chondrite analog. Planet. Sci. J. 2, 58. doi:10.3847/PSJ/abbb97
Szalay, J. R., and Horanyi, M. (2016). The impact Ejecta environment of near Earth asteroids. Astrophys. J. Lett. 830, L29. doi:10.3847/2041-8205/830/2/l29
Thompson, M. S., Zanetta, P.-M., Zega, T. J., Noguchi, T., Yurimoto, H., Nakamura, T., et al. (2022). Evidence for micrometeoroid bombardment on the surface of asteroid Ryugu. 53rd Lunar and Planetary Science Conference, abstract #2134, The Woodlands, March 2022.
Yada, T., Abe, M., Okada, T., Nakato, A., Yogata, K., Miyazaki, A., et al. (2022). Preliminary analysis of the Hayabusa2 samples returned from C-type asteroid Ryugu. Nat. Astron. 6, 214–220. doi:10.1038/s41550-021-01550-6
Yada, T., Fujimura, A., Abe, M., Nakamura, T., Noguchi, T., Okazaki, R., et al. (2014). Hayabusa-returned sample curation in the planetary material sample curation facility of JAXA. Meteorit. Planet. Sci. 49 (2), 135–153. doi:10.1111/maps.12027
Keywords: remote sensing, organics, mineralogy, origins, spectroscopy, calibration, DATA FUSION
Citation: Maturilli A, Schwinger S, Bonato E, Helbert J, Baqué M, Hamm M, Alemanno G and D’Amore M (2022) Linking remote sensing, in situ and laboratory spectroscopy for a Ryugu analog meteorite sample. Front. Space Technol. 3:1023393. doi: 10.3389/frspt.2022.1023393
Received: 19 August 2022; Accepted: 03 October 2022;
Published: 24 October 2022.
Edited by:
Yuichi Tsuda, Japan Aerospace Exploration Agency, JapanReviewed by:
Yun Zhang, University of Maryland, United StatesEdward Anthony Cloutis, University of Winnipeg, Canada
Copyright © 2022 Maturilli, Schwinger, Bonato, Helbert, Baqué, Hamm, Alemanno and D’Amore. This is an open-access article distributed under the terms of the Creative Commons Attribution License (CC BY). The use, distribution or reproduction in other forums is permitted, provided the original author(s) and the copyright owner(s) are credited and that the original publication in this journal is cited, in accordance with accepted academic practice. No use, distribution or reproduction is permitted which does not comply with these terms.
*Correspondence: Alessandro Maturilli, Alessandro.maturillil@dlr.de